DOI:
10.1039/C5RA04762E
(Paper)
RSC Adv., 2015,
5, 49633-49642
Cyclosporine A loaded self-nanoemulsifying drug delivery system (SNEDDS): implication of a functional excipient based co-encapsulation strategy on oral bioavailability and nephrotoxicity†‡
Received
18th March 2015
, Accepted 20th May 2015
First published on 20th May 2015
Abstract
The present work focusses on the formulation development and evaluation of a functional excipient (surfactant stabilizer), a vitamin E TPGS loaded self-nanoemulsifying drug delivery system (SNEDDS), for improving the deliverability and safety profile of cyclosporine A (CyA). The saturation solubility of individual bioactive compounds were evaluated in a series of oils and surfactants. In addition, a ternary phase diagram-based exhaustive optimization was performed to identify the nanoemulsification region that yields the desired quality of attributes and maximum loading capacity of CyA and vitamin E TPGS. The optimized formulation exhibited excellent stability in simulated gastrointestinal fluids. In vitro drug release studies revealed significantly higher rapid release of CyA from CyA-TPGS SNEDDS as compared to that of the clinically available counterpart Bioral® or in-house CyA-SNEDDS. In vivo pharmacokinetics further demonstrated a 4.48-fold increase in oral bioavailability in the case of the developed formulation as compared to Bioral®. CyA induced reactive oxygen species (ROS) generation in HEK cell lines was significantly diminished in the case of CyA-TPGS SNEDDS in contrast to that of CyA SNEDDS, Bioral® and the CyA + vitamin E TPGS physical mixture. The results were further corroborated by in vivo nephrotoxicity studies wherein the levels of biochemical markers of nephrotoxicity, blood urea nitrogen and serum creatinine levels were comparable to that of a negative control in the case of the developed formulation in contrast to that of Bioral®. In a /nutshell, the employed strategy of functional excipient loaded SNEDDS poses a viable strategy for developing value-added nanoformulations of CyA.
1. Introduction
Cyclosporine A (CyA), a lipophilic cyclic polypeptide, was first isolated from a crude extract of the fungus Tolypocladium inflatum Gams in 1972. It acts as an immunosuppressant agent and is clinically utilized for immunomodulation in different surgical processes and prevents the physiological rejection mechanisms following kidney, liver, bone marrow and pancreas transplantation. CyA is also used in rheumatoid arthritis, psoriasis, aplastic anemia, atopic dermatitis, ulcerative colitis and severe corticosteroid dependent asthma. The FDA has approved CyA for the prevention of transplant rejection and various dermatological diseases such as psoriasis, alopecia areata, pyoderma gangrenosum etc.1
Noteworthily, it is one of few peptide drugs which is insensitive to enzymatic degradation in gastrointestinal fluids.2 CyA possess a very low aqueous solubility (6.6 μg ml−1) and low intestinal permeability due to the presence of a cyclic undecapeptide chain and a high molecular weight. Extensive pre-systemic metabolism in the gut wall, liver and P-glycoprotein (P-gp) efflux in enterocytes further reduces the oral bioavailability of CyA.3 The low oral bioavailability of CyA led to the development of Sandimmune®, which is an oily concentrate consisting of a lipidic ingredient (olive oil) and solubility enhancers intended for oral administration after the dilution with different fluids like fruit juice or cola. This nonstandard method resulted in the formation of larger droplet sizes of the emulsion, which after oral administration leads to variable oral bioavailability varying from 10–60% along with patient noncompliance due to the unpleasant taste of the formulation.4 Subsequently, Neoral® (a microemulsion formulation of CyA) was developed which could overcome the problems of low and variable absorption exhibited by Sandimmune®. Neoral® showed rapid drug absorption which was revealed by 70% and 40% increases in the peak concentration and area under curve (AUC) respectively as compared to Sandimmune®.5 However, the commercially available formulations of CyA exhibit nephrotoxicity during different surgical procedures.6 Sandimmune® has shown nephrotoxicity in 25% of cases of renal transplantation, 38% of cases of cardiac transplantation, and 37% of cases of liver transplantation. The nephrotoxicity of CyA is associated with elevated levels of blood urea nitrogen (BUN) and creatinine in the ranges of 35–45 mg dl−1 and 2.0–2.5 mg dl−1, respectively. Of note, the relatively newer formulation of CyA, Neoral®, is also associated with nephrotoxicity measured as a function of reduction in the renal plasma flow and glomerular filtration rate.7 Besides the nephrotoxicity, hepatotoxicity, hypertension, hyperglycaemia and other malignancies are also associated with the free CyA as well as with the marketed formulations.6
Furthermore, in order to overcome the problems of low oral bioavailability and nephrotoxicity associated with free CyA and marketed formulations, various nanoformulation based approaches have also been investigated for oral bioavailability enhancement and reducing the nephrotoxicity of CyA. In this regard, PLGA nanoparticles,8–10 solid lipid nanoparticles,11 pH sensitive nanoparticles,12 charged nanoparticles,13 cubic nanoparticles,14 hydroxypropyl methylcellulose phthalate nanoparticles,15 polycaprolactone nanoparticles,16 stearic acid nanoparticles17 etc. have been mainly formulated for oral bioavailability enhancement and some of the formulations have been investigated for reducing the nephrotoxicity of CyA.
Additionally, co-administration of different antioxidants with CyA has also been investigated to reduce the side effects associated with CyA. These include different exogenous antioxidants like vitamin E, vitamin C, epigallocatechin gallate, resveratrol, curcumin, melatonin etc.18–20 Among the various reported antioxidants, vitamin E TPGS (D-alpha tocopherol polyethylene glycol 1000 succinate) is a water soluble derivative of vitamin E and has been employed to enhance the bioavailability of many poorly soluble drugs including CyA by inhibiting the P-gp efflux.21 Vitamin E TPGS is converted into vitamin E which acts as an antioxidant22 and scavenges free radicals, thereby reducing free radical toxicity. Nevertheless the oral bioavailability of most of the antioxidants is limited by their poor aqueous solubility and instability in the GI tract, which ultimately impairs their antioxidant potential.
In this regard, different nanoformulations containing the antioxidant have been investigated to reduce CyA induced nephrotoxicity.9,23 A quercetin loaded self-nanoemulsifying drug delivery system has been recently developed by our group which revealed significantly higher antioxidant potential as compared to the free drug suspension against CyA induced nephrotoxicity.24
The present research work encompasses the co-loading of CyA and the functional antioxidant, vitamin E TPGS, into a self-emulsifying formulation comprising an oil, surfactant and co-surfactant with the intention to increase the oral bioavailability of CyA with a vis-à-vis reduction in CyA induced nephrotoxicity. Furthermore, the problems of poor loading of antioxidant, poor industrial scalability and stability have also been addressed. The said potential of the developed formulation has been established in comparison to the free drug and Bioral™ (a commercially available formulation of CyA in India, manufactured by Panacea Biotec, bioequivalent to Neoral®).
2. Materials and methods
2.1 Materials
Cyclosporine A and vitamin E TPGS were obtained as generous gifts from Panacea Biotec Limited, New Delhi, India and Isochem, Vert-le-Petit, France, respectively. Plurol Oleique, Peceol, Gelucire, Labrafil M 2130 CS and Labrafil 1944 CS were obtained as gift samples from Gattefosse, France. Captex 355, Capmul MCM and Capmul MCM-C8 were procured as generous gifts from Abitec Corporation, Janesville, USA. Cremophor RH 40, Tween 20, Tween 80, Triton X-100 and Coumarin-6 (C-6) were purchased from Sigma, St. Louis, MO, USA. All other oils were procured from local suppliers. Dulbecco’s modified Eagle’s medium (DMEM), fetal bovine serum (FBS), antibiotics (antibiotic–antimycotic solution) and Hanks’s balanced salt solution (HBSS) were purchased from PAA Laboratories GmbH, Pasching, Austria. Ethyl acetate (LR grade), acetonitrile (HPLC grade), methanol (LR grade) and methanol (HPLC grade) were purchased from Fisher Scientific, Pittsburgh, PA, USA. Creatinine Assay Kit and Urea Assay Kit were purchased from Accurex Biomedical Pvt Ltd, Mumbai, India. Ultra-pure deionized water (LaboStar™ ultrapure water systems, Erlangen, Germany) was used for all the experiments. All other reagents used were of analytical grade.
2.2 Screening of oily phases and compatibility testing of CyA and vitamin E TPGS
The oily phases to be used in the formulation of a self-nanoemulsifying drug delivery system were screened on the basis of their solubilization potential. The saturation solubility of both CyA and vitamin E TPGS in a series of oils was estimated using the shake flask method.25 Briefly, excess amounts of CyA and vitamin E TPGS were added to vials containing 0.5 g of the oils and vortexed for 2 min. The obtained homogenous mixture was then incubated in a shaker water bath (Lab Tech, Korea) operated at 50 strokes per min for 72 h at 37 °C. Subsequently, mixtures were centrifuged at 500g for 10 min to separate excess drug and supernatant diluted with ethyl acetate and methanol. The amounts of CyA and vitamin E TPGS solubilized in various oils were quantified by a validated HPLC method at 210 nm for CyA and at 285 nm for vitamin E TPGS using methanol as a blank (ESI‡). In a separate set of experiments, the compatibility testing of CyA and vitamin E TPGS with the selected oil was also carried out incubating the mixture for a stipulated period of time. The bioactive compounds were then extracted in methanol and estimated quantitatively using the validated HPLC method.10
2.3 Screening of surfactants and co-surfactants
Different surfactants were screened for emulsification ability of the selected oil phase. Briefly, a 1
:
1 ratio of surfactant and oil were mixed and gently heated at 50 °C for homogenization of the components. Similarly, co-surfactants were also screened by mixing oil, surfactant and co-surfactant in the ratio of 3
:
2
:
1, respectively. 250 mg of each mixture was then diluted with distilled water to 50 ml in a stoppered volumetric flask. The screening parameters were set as droplet size, PDI, % transparency and ease of emulsification, estimated as per the standard protocols.24
2.4 Construction of a pseudo ternary phase diagram
Ternary diagrams with different concentrations of surfactant, co-surfactant and oil were plotted, each of them representing an apex of the triangle. In the ternary mixtures the concentration of the selected oil, surfactant and co-surfactant was varied from 30–70% (w/w), 10–70% (w/w) and 0–30% (w/w), respectively. Various compositions were prepared by incrementing the co-surfactant concentration by 5% while keeping the oil concentration constant. Surfactant concentration was adjusted appropriately so as to make the resulting mixture 100%.26 Forty-seven such mixtures with varying compositions of surfactant, co-surfactant and oil were prepared and evaluated for droplet size, PDI and % transparency. The compositions which formed submicron emulsions <200 nm were plotted on the pseudo ternary phase diagram.
2.5 Optimization of CyA and vitamin E TPGA loading into the SNEDDS
The effect of CyA on the phase behavior and area of the nanoemulsion formation was studied independently and in combination with vitamin E TPGS. Briefly, varying concentrations of CyA were dissolved in the representative formulations of the nanoemulsion region obtained previously with a blank formulation and the resulting droplet size and PDI were plotted in the ternary diagram. Similarly, the effect of vitamin E TPGS on the resulting nanoemulsification region was studied. Special attention was given to consider the surfactant properties of the vitamin E TPGS.
2.6 Stability studies
Stability in simulated gastrointestinal fluids. The representative formulations from the nanoemulsification region were evaluated for their ability to form the nanoemulsion in simulated gastric fluids (SGF, pH 1.2) and simulated intestinal fluids (SIF, pH 6.8). Briefly, 250 mg of the selected SNEDDS formulation was diluted with 50 ml of SGF and SIF and allowed to stand for 2 h. Subsequently, droplet size, PDI and self-emulsification time of the resultant nanoemulsion were determined.
Robustness to dilution. The optimized SNEDDS composition was subjected to dilution with various vehicles such as water and buffer solutions (pH 1.2 and pH 6.8). Briefly, 50 mg of the SNEDDS was diluted with the above vehicles around 200, 400, 600 and 800 times dilution. The diluted nanoemulsion was studied at 0, 2 and 6 h by droplet size analysis and visual observation of precipitation of CyA and vitamin E TPGS.
Freeze thaw stability. The optimized SNEDDS composition was further exposed to freeze thaw cycles to evaluate the stability of the formulation at extreme temperatures for a short period of time. The formulation was subjected to 3 freeze–thaw cycles, which included freezing below 0 °C for 24 h followed by thawing at 40 °C for 24 h. The formulation was then evaluated for the critical quality attributes such as droplet size, PDI and self-emulsification time.
Long term stability. The room temperature stability of the optimized formulation was evaluated for six months. The formulation was checked for the spontaneity of nano emulsion formation, droplet size, PDI and drug content at regular intervals as per the aforementioned protocol.
2.7 Physicochemical evaluation and characterization of the CyA-TPGS-SNEDDS
Transmission electron microscopy (TEM) of the reconstituted nanoemulsion. The morphology of the submicron oil droplets after dilution of the CyA-TPGS-SNEDDS was evaluated by transmission electron microscopy (FEI Tecnai G2). Diluted samples (1
:
1000) were negatively stained with a 1% aqueous solution of phosphotungstic acid and visualized after placing on 200-mesh carbon coated grids under the electron microscope at 10–100k-fold enlargements at an accelerating voltage of 60.0 kV.27
2.8 In vitro drug release
The CyA SNEDDS and CyA-TPGS-SNEDDS equivalent to 25 mg of CyA dispersed in simulated gastric (pH 1.2 for 2 h) and intestinal fluid (pH 6.8 for 8 h and pH 7.4 up to 24 h) were added to a dialysis bag (MWC 12
000 Dalton, Sigma, USA). The formulation filled dialysis bag was dispensed in 20 ml of 0.1 N HCl and a phosphate buffer containing 0.25% w/v SLS and subjected to shaking over a shaker water bath operated at 37 °C and 100 rpm. Aliquots of 1 ml were withdrawn at the predetermined time intervals (0.5, 1, 2, 4, 8, 12, 18 and 24 h) and replenished with fresh media each time to maintain the sink conditions. The cumulative amount of CyA released was analyzed by a validated HPLC method.
2.9 In vivo pharmacokinetic study
All of the animal experiments were carried out under the guidelines compiled by CPCSEA (Committee for the Purpose of Control and Supervision of Experiments on Animals, Ministry of Culture, Govt. of India) and the study protocols were duly approved by the Institutional Animal Ethics Committee of NIPER, Mohali, India. In vivo pharmacokinetic studies of the CyA-TPGS-SNEDDS were performed using female Sprague Dawley Rats. The animals were acclimatized at standard housing conditions for one week before the experiments. Rats were divided into five groups each containing five animals. Group I received the marketed formulation of CyA (Bioral™) and group II received the free CyA. Group III received the free CyA and vitamin E TPGS. Group IV and V received the CyA-SNEDDS and CyA-TPGS-SNEDDS, respectively. All of the formulations were orally administered in the dose of 25 mg kg−1 and 80 mg kg−1 equivalent to CyA and free TPGS respectively. Considering the poor aqueous solubility of CyA, it was administered as a suspension with 1% xanthum gum as the suspending agent. The blood samples (∼0.2 ml) were collected at 1, 2, 4, 8, 12, 24, 48 and 72 h from the retro-orbital plexus under mild ether anesthesia into heparinized microcentrifuge tubes. The plasma was separated by centrifuging the blood samples at 500g for 10 min at 15 °C and the CyA content was estimated using a validated HPLC method. The obtained plasma concentration profile was then subjected to pharmacokinetic modeling using Kinetica Software (Version 5.0, Thermo) and various pharmacokinetic parameters were assessed.28,29 The limit of quantification for CyA was 1.76 μg ml−1 and that of vitamin E TPGS was 1.89 μg ml−1 with an injection volume of 20 μl (ESI‡).
2.10 CyA induced intracellular reactive oxygen species (ROS) in HEK cell lines
The intracellular ROS generation by CyA was evaluated by employing a standard 2′,7′-dichlorodihydrofluorescein diacetate (H2DCFDA) fluorescence assay protocol.24 Briefly, Human Embryonic Kidney (HEK) cells were maintained in minimum Eagle’s medium supplemented with 2.2% sodium bicarbonate, 1 mM sodium pyruvate, 10% fetal bovine serum (FBS), 100 U ml−1 penicillin and 100 μM streptomycin (Sigma, USA) at 37 °C/5% CO2. The confluent cells were harvested and seeded at a cell density of 1.0 lac cells per well in a 6-well tissue culture plate. The seeded cells were allowed to adhere overnight. The cells were then exposed to CyA, CyA + vitamin E TPGS, the CyA SNEDDS and CyA-TPGS SNEDDS for 12 h. The doses of 10 μg ml−1 of CyA and 32 μg ml−1 of vitamin E TPGS, proportional to that loaded in the developed formulation were employed for the studies. After 12 h, the cells were then washed with PBS, pH 7.4 and incubated with 50 μM H2DCFDA for 30 min. The cells were then fixed using glutaraldehyde 2.5% v/v and visualized under a confocal laser scanning microscope (CLSM) (Olympus FV1000, USA) with Ex/Em: ∼492–495/517–527 nm. In a separate set of experiments, a cell cytotoxicity assay for the exposed time period was assessed using a standard MTT cell viability assay.
2.11 In vivo nephrotoxicity
Nephrotoxicity was investigated in healthy mice (Swiss strain) of uniform body weight (25 ± 2 g) with no prior drug treatment. Mice were divided into five groups, wherein group I received the marketed formulation of CyA (Bioral™), group II received a suspension of CyA containing 1% xanthum gum as a suspending agent, group III received a suspension of CyA and vitamin E TPGS solution, group IV received the CyA-SNEDDS and group V was orally administered the CyA-TPGS-SNEDDS. All of the formulations were orally administered daily for 1 week at the dose of 25 mg kg−1 equivalent to the free CyA and 80 mg kg−1 equivalent to vitamin E TPGS. Following the treatment blood was collected and evaluated for creatinine and blood urea nitrogen (BUN) levels using commercially available kits.30
2.12 Statistical analysis
All of the data are expressed as mean ± standard deviation (SD) for all in vitro results and as mean ± standard error of mean (SEM) for all in vivo results. Statistical analysis was performed using Sigma Stat (version 3.5) utilizing a one-way ANOVA followed by a Tukey–Kramer multiple comparison test. p < 0.05 was considered as a statistically significant difference.
3. Results
3.1 Saturation solubility and compatibility studies
Fig. 1 depicts the solubility of CyA and vitamin E TPGS in various oils. Capmul MCM showed the highest solubilization potential for both CyA and vitamin E TPGS. Although no statistically significant difference in solubility was noted for Capmul MCM and Capmul MCM C8, the former was preferably chosen owing to its greater ease of emulsification being a mixture of mono- and diglycerides.31 Compatibility studies were also carried out which revealed no signs of interaction. A HPLC chromatogram of blank Capmul showed a peak at 0.67 min which could be due to the caprylic acid content of the Capmul MCM. A HPLC chromatogram of CyA extracted from Capmul MCM showed an intense peak at 2.833 min whereas the vitamin E TPGS extracted from Capmul MCM showed a peak at 7.87 min. In a similar manner, the oily sample containing CyA and vitamin E TPGS showed the peaks at the same retention time as revealed in drug samples solubilized in Capmul MCM separately (ESI‡).
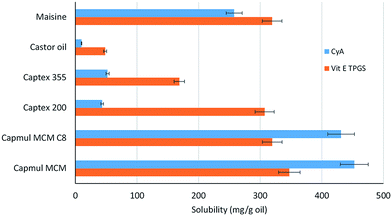 |
| Fig. 1 Solubility of CyA and vitamin E TPGS in various oils. Each data point represents the mean ± SD (n = 6). | |
3.2 Screening of surfactants and co-surfactants
Table 1 depicts various quality attributes of the formulations subjected to surfactant screening. A remarkable difference in droplet size, PDI and % transmittance values were noted with different surfactants. Based on the observed quality attributes, Cremophor RH 40 was chosen as the preferred surfactant. Tween 20, Tween 80 and Cremophor EL also showed marginally acceptable results hence a secondary screening step was employed which comprised an estimation of the CyA solubility in the presence of a 10% surfactant solution. The results revealed a remarkably higher solubilisation potential of Cremophor RH 40 and hence it was selected for further studies (ESI‡).
Table 1 Emulsification ability of various surfactants (oily phase-Capmul MCM)a
Surfactants |
Particle size |
PDI |
% Transmittance |
Values are expressed as the mean ± SD (n = 6). |
Tween 20 |
162.3 ± 13.02 |
0.56 ± 0.052 |
72.00 ± 0.156 |
Tween 40 |
188.8 ± 20.48 |
0.987 ± 0.240 |
69.39 ± 0.52 |
Tween 60 |
256.9 ± 14.05 |
0.581 ± 0.134 |
68.28 ± 0.243 |
Tween 80 |
194.7 ± 12.07 |
0.461 ± 0.042 |
73.17 ± 0.286 |
Gelucire |
214.7 ± 16.26 |
0.457 ± 0.321 |
36.92 ± 0.168 |
Cremophor EL |
147.3 ± 8.24 |
0.49 ± 0.025 |
75.10 ± 0.137 |
Cremophor RH 40 |
89.12 ± 12.37 |
0.37 ± 0.049 |
88.24 ± 0.581 |
Similarly, co-surfactant screening was also carried out which revealed Labrafil 1944 CS to yield the best quality attributes among the tested combinations (Table 2). Although % transmittance of ethanol, ethylene glycol and transcutol was relatively better, no statistical difference was noted and hence on the basis of droplet size and PDI, Labrafil 1944 CS was selected as a co-surfactant for further studies.
Table 2 Emulsification studies on Capmul MCM
:
Cremophor RH 40
:
co-surfactanta
Co-surfactant |
Droplet size |
PDI |
% Transmittance |
Values are expressed as mean ± SD (n = 6). |
Labrafil 1944 CS |
38.91 ± 4.57 |
0.289 ± 0.146 |
85.90 ± 2.84 |
Ethylene glycol |
101.8 ± 12.05 |
1 ± 0.025 |
87.16 ± 6.22 |
PEG |
63.67 ± 9.57 |
0.92 ± 0.752 |
85.83 ± 0.57 |
Transcutol |
117.7 ± 11.25 |
0.675 ± 0.54 |
87.23 ± 4.73 |
Ethanol |
96.86 ± 3.45 |
0.637 ± 0.613 |
88.30 ± 6.64 |
Plurol Oleique |
252.8 ± 3.47 |
0.474 ± 0.428 |
57.7 ± 7.24 |
3.3 Construction of pseudo ternary phase diagrams and optimization of drug loading
Pseudo ternary phase diagrams were constructed using Capmul MCM as the oil, Cremophor RH 40 as the surfactant and Labrafil 1944 CS as the co-surfactant, representing the three apexes of the triangle. Fig. 2A represents the ternary phase diagram constructed with a blank formulation and the filled area denotes the nanoemulsion region. Fig. 2B denotes the effect of CyA loading on the nanoemulsification region as noted for the blank formulation whereas Fig. 2C depicts the effect of co-loading of CyA and vitamin E TPGS. Based on the preliminary studies, the quanta of CyA and vitamin E TPGS were set as 25 mg and 80 mg per gram of formulation, respectively (ESI‡). A representative formulation from the nanoemulsion region of Fig. 2C i.e. 40
:
40
:
20 (oil
:
surfactant
:
co-surfactant), was considered as optimized and selected for further studies. The quality attributes of said formulation (CyA and vitamin E TPGS loaded) were as follows: droplet size 72.19 ± 7.48 nm, PDI 0.24 ± 0.186, and % transmittance 89.53 ± 4.26.
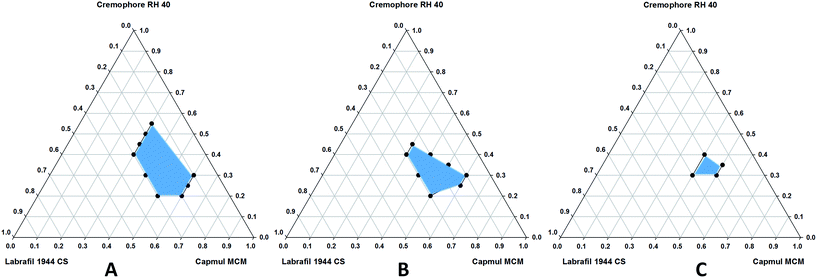 |
| Fig. 2 Ternary phase diagrams depicting the nanoemulsion region of (A) the blank formulation, (B) the CyA-SNEDDS and (C) the CyA-TPGS-SNEDDS. | |
3.4 Stability studies
Stability in simulated gastrointestinal fluids and robustness to dilution. Table 3 reflects the critical quality attributes of the developed CyA-TPGS-SNEDDS formulation when reconstituted using various media. As is evident, no statistically significant difference in the quality attributes was noted either as a function of the nature of the diluting media or the time period. The attributes were found to be consistent over a period of time irrespective of the diluting agent and no evidence of drug precipitation or phase separation was noted. Similarly, the developed formulation was also challenged for its robustness to dilution and the quality attributes of the formulations were found to be consistent (data not shown).
Table 3 Stability of the CyA-TPGS-SNEDDS in simulated gastrointestinal fluidsa
Medium |
Initial |
After 2 h |
After 8 h |
Size (nm) |
PDI |
ZP (mV) |
Size (nm) |
PDI |
ZP (mV) |
Size (nm) |
PDI |
ZP (mV) |
ZP: zeta potential; values are expressed as the mean ± SD (n = 6). |
Water |
72.19 ± 7.48 |
0.243 ± 0.01 |
−7.41 ± 1.88 |
79.22 ± 4.22 |
0.216 ± 0.01 |
−8.11 ± 2.17 |
81.39 ± 7.34 |
0.251 ± 0.01 |
−10.79 ± 1.52 |
SGF, pH 1.2 |
80.62 ± 5.11 |
0.292 ± 0.081 |
−4.19 ± 2.33 |
89.34 ± 5.62 |
0.312 ± 0.042 |
−5.68 ± 3.98 |
86.85 ± 9.06 |
0.303 ± 0.038 |
−6.95 ± 3.02 |
SIF, pH 6.8 |
89.28 ± 7.15 |
0.256 ± 0.037 |
−11.24 ± 3.96 |
96.53 ± 12.94 |
0.293 ± 0.015 |
−12.25 ± 2.14 |
98.54 ± 17.79 |
0.314 ± 0.043 |
−11.15 ± 2.91 |
Long term stability studies and at stress conditions of freeze–thaw. The stability of the developed formulation was further assessed as a function of freeze thaw stresses and long term storage. Table 4 and Table 5 depict the quality attributes of the reconstituted nanoemulsion from the CyA-TPGS-SNEDDS subjected to three freeze–thaw cycles and long term stability studies, respectively. As is evident no statistical significance in the quality attributes were noted revealing the robustness of the developed formulation against temperature fluctuations and long term standing.
Table 4 Freeze thaw stability studies of the CyA-TPGS-SNEDDSa
Stress condition |
Initial |
After |
Size |
PDI |
Size |
PDI |
Values are expressed as the mean ± SD (n = 6). |
Room temperature |
72.69 ± 8.44 |
0.254 ± 0.029 |
81.68 ± 7.50 |
0.204 ± 0.045 |
Freeze thaw cycle |
78.15 ± 4.25 |
0.242 ± 0.027 |
82.56 ± 5.99 |
0.257 ± 0.034 |
Table 5 Long term stability of the CyA-TPGS-SNEDDSa
Time period |
Size |
PDI |
Zeta potential (mV) |
CyA content |
Values are expressed as the mean ± SD (n = 6). |
Initial |
57.95 ± 9.46 |
0.221 ± 0.01 |
−8.55 ± 1.44 |
97.24 ± 1.73 |
1 month |
52.99 ± 5.24 |
0.229 ± 0.01 |
−9.12 ± 2.56 |
96.51 ± 1.99 |
2 months |
60.69 ± 8.56 |
0.230 ± 0.02 |
−7.94 ± 2.41 |
96.18 ± 2.62 |
3 months |
50.25 ± 4.75 |
0.232 ± 0.02 |
−9.36 ± 2.27 |
95.90 ± 3.98 |
3.5 Physicochemical evaluation and characterization of the CyA-TPGS-SNEDDS
TEM analysis. Fig. 3 depicts the representative TEM image of the reconstituted CyA-TPGS-SNEDDS nanoemulsion. As is evident, all droplets upon dilution possess a spherical shape, thus confirming the formation of the nanoemulsion and the droplet size correlating well with that of the dynamic light scattering results.
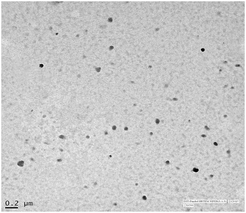 |
| Fig. 3 Representative TEM image of the reconstituted CyA-TPGS-SNEDDS nanoemulsion. | |
3.6 In vitro drug release studies
Fig. 4 shows the cumulative in vitro drug release profile of the free CyA, CyA-SNEDDS and CyA-TPGS-SNEDDS formulations. It was observed that about ∼27%, ∼59% and ∼90% of CyA was released from the developed formulation when incubated with SGF (pH 1.2) for 2 h, SIF (pH 6.8) for 8 h and PBS (pH 7.4) for 24 h, respectively. Of note, ∼33%, ∼46% and ∼68% of CyA could be released from the CyA SNEDDS. In contrast, ∼20% of the CyA was released from the free CyA over a period of 24 h. The results are suggestive of a positive contribution of the vitamin E TPGS in improving the drug release from the developed formulation.
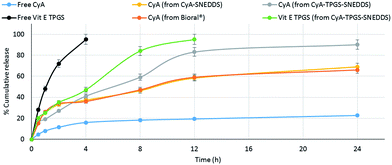 |
| Fig. 4 In vitro drug release profile of various formulations. Each data point represents the mean ± SD (n = 6). | |
3.7 In vivo pharmacokinetics
Fig. 5 represents the plasma concentration time profile of the various formulations. The co-administration of vitamin E TPGS solution along with the free CyA marginally increased the drug plasma concentration of CyA (Table 6). The loading of CyA in the SNEDDS formulation significantly increased the drug plasma concentration as compared to the free CyA and free CyA co-administered with vitamin E TPGS. The CyA-SNEDDS significantly increased the total plasma concentration of CyA at all the time points as compared to that of the free drug and that co-administered with vitamin E TPGS. However the plasma concentration time profile of the CyA-SNEDDS and Bioral® were found to be comparable. Of note, the CyA-TPGS-SNEDDS could significantly increase the oral bioavailability of CyA as compared to that of both the CyA-SNEDDS and Bioral®. Overall, 4.48-fold, 1.96-fold and 1.46-fold increases in the AUC were noted in case of the developed formulation as compared to that of the free CyA, free CyA + vitamin E TPGS and Bioral®, respectively. A similar appreciation in Cmax was also noted in case of the CyA-TPGS-SNEDDS exhibiting 7.04-fold, 3.95-fold and 1.49-fold increases as compared to that of the free CyA, free CyA + vitamin E TPGS and Bioral®, respectively (Table 6).
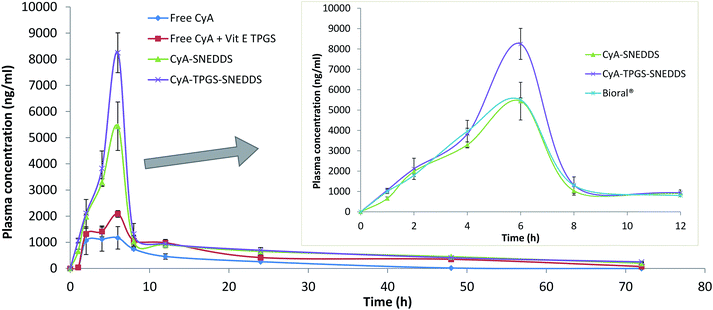 |
| Fig. 5 Plasma concentration time profile of various formulations; each data point represents the mean ± S.E.M (n = 5). | |
Table 6 Mean pharmacokinetic parameters in various formulationsa
Formulation |
Cmax (ng ml−1) |
Tmax (h) |
AUC0–∞(ng ml−1 h−1) |
t1/2 (h) |
Values are expressed as the mean ± S.E.M (n = 5). |
Free CyA |
1171.85 |
6 |
17 195.12 |
7.35 |
Free CyA + vit E TPGS |
2086.065 |
6 |
39 325.39 |
18.05 |
CyA-SNEDDS |
5438.56 |
6 |
63 335.52 |
28.55 |
CyA-TPGS-SNEDDS |
8248.56 |
6 |
76 970.65 |
32.62 |
Bioral® |
5500.58 |
6 |
52 677.2 |
22.4 |
3.8 CyA induced intracellular reactive oxygen species (ROS) in HEK cell lines
The intracellular ROS generation potential of CyA and the capability of vitamin E TPGS to combat the generated ROS were assessed in HEK cell lines to establish the rationale for the selected combination. A H2DCFDA assay revealed remarkably higher ROS generation within HEK cells treated with CyA alone in contrast to that of CyA + vitamin E TPGS (Fig. 6). Interestingly, the ROS generation was also remarkably higher in the treatment group of the CyA SNEDDS as compared to that of the plain CyA, CyA-TPGS SNEDDS and CyA + vitamin E TPGS.
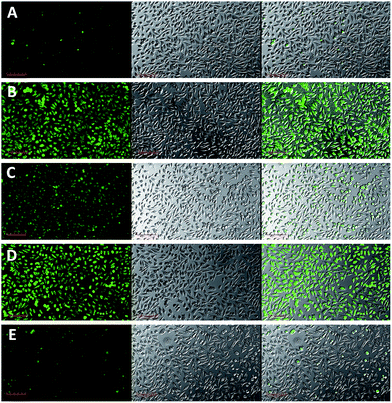 |
| Fig. 6 Intracellular ROS generation of S. cerevisiae exposed to (A) vehicle treated, (B) CyA, (C) CyA + vitamin E TPGS, (D) the CyA SNEDDS and (E) the CyA-TPGS SNEDDS. Channel 1 represents the fluorescence emerging from intracellular DCF converted from H2DCFDA in response to oxidative stress. Channel 2 represents the phase contrast images of the cells and Channel 3 represents the overlay of Channel 1 and Channel 2. Scale bar represents 100 μm. | |
3.9 In vivo nephrotoxicity
A remarkable increase in the levels of creatinine and BUN were noted in the free CyA treated group suggestive of the potential nephrotoxicity of CyA (Fig. 7). A statistically significant reduction (p < 0.05) in the levels of biochemical markers was noted when vitamin E TPGS was co-administered with the free CyA, however the levels still remained quite higher than that of the vehicle treated animal group. Noteworthily, no statistically significant difference (p > 0.05) in the creatinine and BUN levels of the CyA-SNEDDS and Bioral® treated animal groups was noted. However, the levels were remarkably higher (p < 0.001) than the control group in both cases. Interestingly, the CyA-TPGS-SNEDDS significantly reduced the BUN levels (p < 0.05) and creatinine levels (p < 0.001) as compared to Bioral® as well as with the free CyA, and the levels were normalized as compared to that of the control group indicative of the antioxidant potential of vitamin E TPGS present as a functional excipient in the developed system.
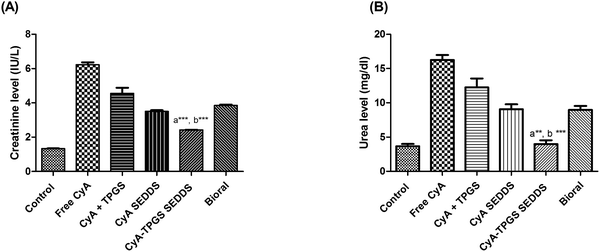 |
| Fig. 7 Effect of various formulations on the nephrotoxicity markers: (A) the creatinine level and (B) the BUN level. Each data point represents the mean ± S.E.M (n = 5). | |
4. Discussion
Self-nanoemulsifying drug delivery systems (SNEDDS) pose great potential in the co-encapsulation of multiple bioactive compounds owing to their high solubilization potential of oils. Solubility studies were extensively carried out to identify a suitable oily phase for the rationalized development of SNEDDS. Capmul MCM, being a mixed composition of lower order synthetic glycerides such as caprylic acids, preferably solubilized both the drug and functional excipient (antioxidant) in the highest amounts (Fig. 1). The implementation of synthetic analogues as compared to the natural origin pose advantages in terms of chemical uniformity and relatively lesser amounts of known impurities. Further, Cremophor RH 40 exhibited excellent solubilizing effects vis-à-vis good emulsification capabilities and hence was chosen as the preferred surfactant for further development of SNEDDS (Table 1). Subsequently, a co-surfactant usually improves upon the PDI of the SNEDDS formulation.25 The co-surfactant screening studies revealed the potential droplet stabilization effects of Labrafil 1944 CS (PEG-8-oleate/linoleate) for the developed formulation in contrast to ethylene glycol, ethanol, transcutol, Plurol Oleique, etc. and hence was selected for further study (Table 2). Drug loading of the bioactive compounds within the developed formulation was further optimized using pseudo ternary phase diagrams. The shaded region within the ternary diagrams represents the nanoemulsification region which yields the desired droplet size and PDI. A gradual decrease in the nanoemulsification region was noted from the blank formulation to the simultaneous co-loading of bioactive compounds reflecting the limited loading capacity of the system (Fig. 2). The results were in line with our previous experiences on the development and optimization of SNEDDS.24
Furthermore, the developed formulation exhibited superior stability in simulated gastrointestinal fluids (Table 3) which could be in part attributed to exhaustive optimization and in part inclusion of the multifunctional excipient, vitamin E TPGS, which can act as a solubilizer and stabilizer along with the therapeutic effects of antioxidants.32 In addition, the formulation was also found to be stable after long term standing and against temperature fluctuations in freeze thaw cycles (Tables 4 and 5).
A morphological evaluation of the reconstituted nanoemulsion using TEM analysis further revealed the spherical shape of the droplets and the droplet size being in good accordance with the dynamic light scattering results (Fig. 3). In vitro drug release studies of the developed formulation revealed some interesting aspects with respect to the influence of the release characteristics as a function of physicochemical properties. The drug release of the highly lipophilic drug, CyA was enhanced upon incorporation into the SNEDDS formulation. However, no statistically significant difference (p > 0.05) was noted among the drug release of CyA from the in-house CyA-SNEDDS and Bioral®. Interestingly, among Bioral® and the CyA-TPGS-SNEDDS, the drug release was relatively rapid in later cases suggesting a strong influence of the formulation components on the physicochemical performance of the system (Fig. 4). Furthermore it was also noted that drug release was affected as a function of a variety of factors such as droplet size, dilution media, physiochemical properties, localization of the drug within the droplet (either within the droplet or at oil–surfactant/water interface), etc. Interestingly, such balancing behavior of the SNEDDS could be correlated to their spontaneity in formation upon exposure to physiological media, which in actual fact depends upon the careful selection of surfactants and co-surfactants in appropriate concentrations, thereby highlighting the significance of an exhaustive optimization for the development of the SNEDDS compositions.
In vivo pharmacokinetic studies were further carried out to assess the delivery potential of the developed formulation. From the results it was quite evident that CyA (free base) is poorly absorbed owing to its higher lipophilicity (Table 6). Interestingly, the co-administration of vitamin E TPGS along with CyA did result in a marginal bioavailability enhancement (Fig. 5). However, a remarkable appreciation in bioavailability was noted only for the developed formulation which could be attributed to the promising role of the co-encapsulation strategy in the simultaneous delivery of therapeutics with opposite physicochemical properties. The results were in line with our previous results.33,34 The key reasons attributed to the bioavailability enhancement includes the high solubilization potential of the SNEDDS, P-gp inhibition effects of vitamin E TPGS, alternate absorption pathway and superior encapsulation of CyA within the nanoemulsion droplets.35
The safety profile of the developed formulation was further assessed in vitro as a function of CyA induced ROS generation within HEK cell lines. CyA induced distress in kidney cell lines is well reported in the literature and hence was adopted as a marker to predict the nephrotoxicity potential of the developed formulations.36 Clinically, acute doses of CyA leads to the transient reduction in the glomerular filtration rate while chronic administration is reported to induce renal impairment and fibrosis.7 These detrimental effects have been primarily mediated by series of mediators including ROS.37 Cell culture studies revealed the remarkable potential of vitamin E TPGS in combating the CyA induced intracellular ROS in the developed formulation (CyA TPGS SNEDDS) in contrast to the plain drug combinations and the CyA SNEDDS (Fig. 6). The results reveal the complexities associated with the potent molecule CyA wherein a concomitant approach of bioavailability enhancement and toxicity reduction is desirable. The developed formulation surpasses the clinical relevance as compared to that of the clinical formulation, Bioral™, wherein only the bioavailability enhancement has been addressed.
The potential of the developed formulation was further established at the pre-clinical level by assessing the levels of the nephrotoxicity biochemical markers in animals treated with various CyA formulations. The results revealed a significant reduction in these parameters in the case of the CyA-TPGS-SNEDDS as compared to the marketed formulation (Bioral®) as well as with the free CyA (Fig. 7). The observed advantages with the developed formulation could be attributed to the potent antioxidant of vitamin E TPGS, playing a critical role in arachidonic acid metabolism mediated by processes such as prostaglandin formation, affecting the release of arachidonic acid and alterations in the lipoxygenase activities.
5. Conclusions
The developed formulation poses great potential in increasing the oral bioavailability and reducing the toxicity of CyA induced nephrotoxicity. Furthermore, the said formulation also offers unique advantages such as high industrial adaptability and relatively lower scalability challenges. A solid dosage form of the said formulation could be a further line of action for a remarkable improvement in the pharmaceutical stability of the dosage form. Furthermore, multiple dose kinetics could be investigated for deeper insights into the delivery potential of the formulation.
Acknowledgements
The authors are thankful to the Director, NIPER for financial support, necessary infrastructure and facilities. A.K.J. and K.T. are grateful to the Council of Scientific and Industrial Research (CSIR), GoI, New Delhi, for providing research fellowships. The technical support rendered by Mr Dinesh Singh and Rahul Mahajan is also duly acknowledged.
Notes and references
- C. E. Griffiths and J. J. Voorhees, J. Invest. Dermatol., 1990, 95, 53S–55S Search PubMed.
- L. Djekic and M. Primorac, in Colloids in drug delivery, ed. M. Fanun, CRC Press, Boca Raton, FL, USA, 2010, pp. 245–270 Search PubMed.
- S. Jain, A. Mittal, A. K. Jain, R. R. Mahajan and D. Singh, Curr. Nanosci., 2010, 6, 422–431 CrossRef CAS.
- M. E. Burton, L. Shaw, J. J. Schentag and W. Evans, Applied pharmacokinetics & pharmacodynamics: principles of therapeutic drug monitoring, Lippincott Williams & Wilkins, Baltimore, Maryland, USA, 2006 Search PubMed.
- S. Takahara, N. Ichimaru, Y. Kojima, Y. Namba, K. Toki, Y. Shi, K. Yoshimura, K. Matsumiya, N. Nonomura and A. Okuyama, Transplant. Proc., 2004, 36, S456–S460 CrossRef PubMed.
- M. Chadimová, M. Bohdanecká, O. Schück, R. Petrásek and V. Reitschlägerová, Cesk. Patol., 1995, 31, 99–103 Search PubMed.
- P. Barany, P. Stenvinkel, A. Ottosson-Seeberger, A. Alvestrand, J. Morrow, J. J. Roberts 2nd and A. K. Salahudeen, Nephrol., Dial., Transplant., 2001, 16, 580–584 CrossRef CAS PubMed.
- D. Ankola, A. Battisti, R. Solaro and M. N. V. R. Kumar, J. R. Soc., Interface, 2010, 7, S475–S481 CrossRef CAS PubMed.
- J. Italia, P. Datta, D. Ankola and M. Kumar, J. Biomed. Nanotechnol., 2008, 4, 304–312 CrossRef CAS PubMed.
- J. Italia, D. Bhatt, V. Bhardwaj, K. Tikoo and M. Kumar, J. Controlled Release, 2007, 119, 197–206 CrossRef CAS PubMed.
- R. Müller, S. Runge, V. Ravelli, W. Mehnert, A. Thünemann and E. Souto, Int. J. Pharm., 2006, 317, 82–89 CrossRef PubMed.
- J. Dai, X. Wang, Q. Zhang, M. Meng, X. Zhang and W. Lü, Acta Pharmacol. Sin., 2004, 39, 1023–1027 CAS.
- M. El-Shabouri, Int. J. Pharm., 2002, 249, 101–108 CrossRef CAS.
- J. Lai, Y. Lu, Z. Yin, F. Hu and W. Wu, Int. J. Nanomed., 2010, 5, 13–23 CAS.
- X. Wang, J. Dai, Q. Zhang, T. Zhang and G. Xia, Acta Pharmacol. Sin., 2004, 39, 463 CAS.
- M. Carmen Varela, M. Guzmán, J. Molpeceres, M. del Rosario Aberturas, D. Rodríguez-Puyol and M. Rodríguez-Puyol, Eur. J. Pharm. Sci., 2001, 12, 471–478 CrossRef.
- Q. Zhang, G. Yie, Y. Li, Q. Yang and T. Nagai, Int. J. Pharm., 2000, 200, 153–159 CrossRef CAS.
- L. A. Lexis, R. G. Fassett and J. S. Coombes, Basic Clin. Pharmacol. Toxicol., 2006, 98, 68–73 CrossRef CAS PubMed.
- H. S. Nagaraja, S. J. Ravinder, C. Srikumar, P. Thanikachalam, L. Nagarajah and B. K. Anupama, J. Chin. Clin. Med., 2009, 4, 26–31 CAS.
- N. Tirkey, G. Kaur, G. Vij and K. Chopra, BMC Pharmacol., 2005, 5, 15 CrossRef PubMed.
- J. R. Fischer, K. R. Harkin and L. C. Freeman, Vet. Therapeut., 2002, 3, 465–473 Search PubMed.
- T. C. L. Z. Benet and M. F. Hebert, Clin. Pharmacol. Ther., 1996, 59, 297–303 CrossRef.
- K. Sonaje, J. Italia, G. Sharma, V. Bhardwaj, K. Tikoo and M. N. V. R. Kumar, Pharm. Res., 2007, 24, 899–908 CrossRef CAS PubMed.
- N. K. Swarnakar, K. Thanki and S. Jain, RSC Adv., 2013, 3, 14671–14685 RSC.
- A. K. Jain, K. Thanki and S. Jain, Pharm. Res., 2014, 31, 923–945 CrossRef CAS PubMed.
- A. A. Date and M. S. Nagarsenker, Int. J. Pharm., 2007, 329, 166–172 CrossRef CAS PubMed.
- N. K. Swarnakar, K. Thanki and S. Jain, Pharm. Res., 2014, 31, 1219–1238 CrossRef CAS PubMed.
- S. Jain, P. U. Valvi, N. K. Swarnakar and K. Thanki, Mol. Pharmaceutics, 2012, 9, 2542–2553 CrossRef CAS PubMed.
- S. Jain, D. Kumar, N. K. Swarnakar and K. Thanki, Biomaterials, 2012, 33, 6758–6768 CrossRef CAS PubMed.
- M. Das, R. Jain, A. K. Agrawal, K. Thanki and S. Jain, Bioconjugate Chem., 2014, 25, 501–509 CrossRef CAS PubMed.
- D. Pozzi, V. Colapicchioni, G. Caracciolo, S. Piovesana, A. L. Capriotti, S. Palchetti, S. De Grossi, A. Riccioli, H. Amenitsch and A. Lagana, Nanoscale, 2014, 6, 2782–2792 RSC.
- W. Cho, M. S. Kim, J. S. Kim, J. Park, H. J. Park, K. H. Cha, J. S. Park and S. J. Hwang, Int. J. Nanomed., 2013, 8, 1673–1682 Search PubMed.
- A. K. Jain, K. Thanki and S. Jain, Pharm. Res., 2014, 31, 946–958 CrossRef CAS PubMed.
- A. K. Jain, K. Thanki and S. Jain, Mol. Pharmaceutics, 2013, 10, 3459–3474 CrossRef CAS PubMed.
- K. Thanki, R. P. Gangwal, A. T. Sangamwar and S. Jain, J. Controlled Release, 2013, 170, 15–40 CrossRef CAS PubMed.
- F. Lamoureux, E. Mestre, M. Essig, F. L. Sauvage, P. Marquet and L. N. Gastinel, J. Proteomics, 2011, 75, 677–694 CrossRef CAS PubMed.
- N. D. Vaziri, Z. Ni, Y. P. Zhang, E. P. Ruzics, P. Maleki and Y. Ding, Kidney Int., 1998, 54, 482–491 CrossRef CAS PubMed.
Footnotes |
† This work is a part of Indian Patent Application no. 762/DEL/2013 filed on March 15, 2013. |
‡ Electronic supplementary information (ESI) available. See DOI: 10.1039/c5ra04762e |
|
This journal is © The Royal Society of Chemistry 2015 |