DOI:
10.1039/C5RA04712A
(Paper)
RSC Adv., 2015,
5, 35622-35630
Gas-breathing polymer film for constructing switchable ionic diodes†
Received
3rd March 2015
, Accepted 18th March 2015
First published on 18th March 2015
Abstract
Carbon dioxide (CO2), as one of the major byproducts of cellular metabolism, mediates many fundamental behaviors, and its detection is associated with the activation of protein ion channels expressed on olfactory sensory neurons. Inspired by this biological phenomenon, we developed an extraordinary gas-sensitive ion channel, reconfigurable ionic diode and bionic ion pump on the basis of the growth of a gas-responsive polymer film, which can be rendered hydrophilic with CO2 stimulus and hydrophobic with N2 stimulus. In particular, by alternatively purging CO2/N2 into the solutions which are placed on both sides of the channel embedded in the polymer membrane, the smart polymer-coated channel can be either closed or opened, which is independent of voltage polarity. Most intriguingly, under asymmetric stimulation with pH pairs (pH 6.5‖pH 9.0) or with gas inputs (CO2‖N2) on both sides of the channel, a change in voltage polarity can switch the channel between a closed status and open status, leading to the construction of a reconfigurable fluidic diode. Furthermore, by combination of gas inputs and voltage polarity, each of the gates of the channel can be individually manipulated, which is a very important prerequisite for the construction of a bionic ion pump that can be operated under physiologically mild conditions. It is envisaged that this new progress in the bionic pore/channel field will hold great prospects for the application of this kind of biomimetic device for energy storage, sample filtration and seawater desalinization.
Introduction
Carbon dioxide (CO2) has been widely involved in natural activities. The ecosystem has evolved a comprehensive set of CO2-responsive pathways to keep a balance. CO2, as one of the major byproducts of cellular metabolism, mediates many fundamental behaviors, and its detection is associated with the activation of protein ion channels expressed on the olfactory sensory neurons1–9 and transportation through biological gas channels.10,11 It will be intriguing to design an artificial gas-responsive ion channel, ionic diode and ion pump by mimicking the gas-responsive pathway that exists in living systems. Until now, no artificial gas-responsive ion channel, ionic diode and ion pump on the basis of a thin polymer film supported on track-etched membrane have been constructed, although many biomimetic pore/ionic systems have been built up on the basis of the immobilization of organic molecules, polymers, and biomolecules in the pore/channel.12–23
In recent years, abiotic devices that mimic the function and structure of biological counterparts have become alluring and have received increasing attention. Among these artificial designs, incessant endeavors have been devoted to imitating ion channels, which mainly comprise engineered protein ion channels,24 solid nanopores20,25–27 in an ultrathin membrane, asymmetric pores28,29 in a polymer membrane and glass nanopores.30,31 Heretofore, many bioinspired ion conducting channels have been designed based on an artificial nanopore with one gate, which can be switched on and off by various external stimuli such as voltage,32,33 temperature,34 light,35 ions,36 pressure,37 ATP38,39 and pH.40,41 More recently, the Jiang group invented a biomimetic ion pump based on a nanochannel with two gates.13 In that pioneering study, two different responsive polymer brushes were grafted onto each end of the ionic channel and each gate could be switched from on to off or vice versa independently/synchronously by pH stimuli.
Among those external stimuli, pH stimulus is very appealing and easily accessible, and has been applied to change the size, surface charge and wettability of the channels. However, many operation cycles that require repeated addition of acids and bases into the solution can degrade the switching capability of the artificial ion channel and ion pump due to the salt accumulation in the solution. While substituting gas stimuli for pH has several distinct advantages: (1) salt accumulation due to the repeated addition of various pH solutions, which can diminish the performance of the biomimetic device, can be eliminated; (2) drastic conditions such as strong acid and strong base can be replaced by much more milder operation conditions; (3) the whole system can be easily restored to its previous status even after many cycles of operation. Furthermore, another common feature of previously made external-stimuli responsive ion channels is that a responsive monolayer is grafted inside the channel.16,18–20 When the channel/pore is in a closed status, the leakage and switching efficiency is highly diameter-dependent. Insufficient growth of the responsive polymer leads to the failure and leakage of the artificial ion pump, whereas the overgrowth of responsive polymers will deteriorate the performance of the channel. It will be more encouraging for scientists to develop more intelligent materials and devices with leakage-free properties and a high switching efficiency.
In this work, we develop an ionic channel device with both entrances covered by a gas-breathing polymer film, which possess the properties of an artificial ion channel, ion pump and ionic diode. Each gate of this ionic channel can be manipulated individually or synchronously. Purging CO2 into a buffer solution (pH 7) on both sides of the channel membrane can render the polymer films positively charged and hydrophilic, leading to ion transport though the polymer films and to the “on” status of the channel. Whereas, the polymer films can be switched back to a hydrophobic status and the channel completely shuts down when the solutions on both sides of the channel membrane are purged with N2 or replaced with buffer solutions with a pH ≥ 7. Except for the synchronous manipulation of both polymer films on both sides of the channel membrane, each gate can also be individually operated by a combination of gas (or pH) and voltage inputs, which are very important prerequisites for the construction of a bionic ion pump. Most interestingly, under asymmetric stimulation with pH pairs (pH 6.5‖pH 9.0) or with gas inputs (CO2‖N2), the change in voltage polarity can switch the channel between a closed status and an open status, leading to the construction of a reconfigurable ionic diode. The alternative passing of CO2/N2 into the solution can allow each gate of the channel to be operated repeatedly for many cycles, which fully eliminates the salt accumulation and degradation of performance. The second advantage lies in that this approach prevents any leakage and lifts the strict requirement on the size of the channel as well. It is envisaged that this new progress in the bionic pore/channel field will hold great prospects for the application of this kind of biomimetic device for energy storage, sample filtration and seawater desalinization.
Experimental section
Fabrication of the channel
PET (polyethylene terephthalate) membranes (diameter = 3 cm, thickness = 12 μm), that had been irradiated with heavy ions of 2.2 GeV kinetic energy to create single track and multi tracks through the membrane, were obtained from GSI, Darmstadt, Germany and are referred to as “tracked” membranes. NaOH, HCOOH, Na2CO3 and KCl were purchased from Beijing Chemical Reagent Company (Beijing, China). 2A1 was purchased from Dow Corning. All of the chemicals were at least analytical grade. To obtain a conical-shaped pore, each side of the PET membrane was treated with UV light (4 mW cm−2) for one hour to allow the activation of the polymer foil. The polymer membrane was sandwiched between two half compartments, each of which contained etching solution (6 M NaOH), and the etching temperature was maintained at 40 °C. The whole etching process was controlled by monitoring the current through the membrane; a one volt potential difference was applied. The current remained at zero as long as the pore did not break through the membrane. The current after the breakthrough was monitored via a picoammeter/voltage source (Keithley 6487, Keithley Instruments, Cleveland, OH), and when the desired current was reached, the etching process was stopped by replacing the etching solution with a stopping solution (1 M HCOOH plus surfactant 2A1) to neutralize the etching solution in the pore. After the first step of the etching, the membranes were thoroughly washed with purified water and dried under a nitrogen flow. The membrane after the first step of etching was immersed into a vial containing 2 M NaOH at 65 °C for 10 minutes. The diameters of the conical-shaped channel were obtained by measuring the corresponding diameters of the multi-track membrane etched under the same experimental conditions.
Polymer film growth via surface-initiated ATRP
Nascent membranes after chemical etching were immersed in an aqueous solution containing 1% w branched PEI (MW: 25
000) for 8 hours at room temperature, and then the samples were washed with ethanol, followed by nitrogen flow drying. The surface immobilization of the ATRP initiator was adapted from previously published procedures.42 Briefly, 2-bromopropionyl bromide (10 mM) was coupled via an amide linkage to the PEI coated membrane surface in a buffer solution (pH 10, 32 mM Na2CO3). The reaction mixture was cooled in an ice bath, and bubbled with N2 for 30 minutes and lasted for 24 h under N2 protection. Reagents for polymer brush growth including H2O, methanol, 2-(diethylamino)ethyl methacrylate, CuBr and N,N,N′,N′,N′′-pentamethyldiethylenetriamine (PMDETA) were purchased from JK Chemical (Beijing, China) and used without further purification. The polymerization reaction took place for 30 minutes under N2 protection. After the polymerization, the PET film was washed with methanol and H2O several times to remove the redundant CuBr and the other reagents. Then the PET film was dried in a N2 flow and placed under vacuum for subsequent characterization.
Current-versus-voltage measurements (I–V curves)
The prepared PET film with an asymmetrical pore was sandwiched between two half compartments, each filled with conductive electrolyte, in order to measure the I–V curves. The current though the conical-shaped pore was measured with a Keithley 6487 picoammeter (Keithley Instruments, Cleveland, OH) and Ag/AgCl electrodes in a custom-designed electrolyte cell. Transmembrane potentials from −1 V to +1 V were scanned to obtain each I–V curve. All of the experiments were carried out at room temperature. Buffer solutions (0.1 M PBS, 0.1 M KCl) at pH 3.0, 5.0, 7.0 and 9.0 were used for stimulation. For gas stimulation, after purging of the solution with CO2/N2 for the desired time, a buffer solution (0.1 M PBS, 0.1 M KCl) at pH 7 was used to open/close the gate, and each gate of the asymmetric pore could be independently operated.
Results and discussion
Endowing artificial pores with switching features responsive to external stimuli has been the scholastic pursuit over the past several years. Until now, several approaches based on pH,40,41 metal ion,36 voltage32,33 and ligand43 stimuli have been used to mediate the open/closed status of the pore. In this study, a gas-responsive polymer film has been adopted to cover both entrances of the ionic channel. Fig. 1A illustrates the reversible switching process accomplished via alternatively purging CO2 and N2 into the buffer solutions close to the track-etched polymer film. In the presence of CO2, the pH value of the buffer solution drops, leading to the protonation and extension of the polymer and subsequent “on” status of the polymer film (Fig. 1B). Purging the buffer solution with N2 displaces the CO2 and leads to an increase in the pH value, which provokes the deprotonation of the polymer film and drives the polymer film toward hydrophobic and a collapsed status. The whole modification process is presented in the supporting information (Fig. S1 in the ESI†). Additional XPS and NMR characterizations have also proved each modification step (Fig. S2 and S3 in the ESI†).
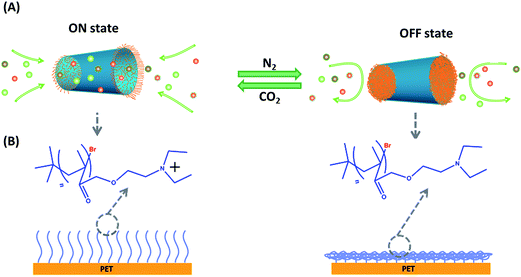 |
| Fig. 1 (A) Cartoon illustration of the “on” status and “off” status of a cylindrical channel covered by polymer films under symmetric stimulation with gas inputs. (B) The charged status due to the protonation of the polymer film and the collapsed status due to the deprotonation of the polymer film. | |
To prove the successful growth of a gas-responsive polymer film on the channel membrane, SEM images were acquired (Fig. 2), including the cross section, and the top and bottom sides of the channel. Continuous polymer films fully covered both sides of the channel membrane after 1 hour of growth (Fig. 2B and C) and the thickness of the polymer film was measured to be 1.6 μm (Fig. 2F). It is clear that domains with various sizes exist in the polymer film and have a much larger size than the channel diameter (200 nm). From the cross section image (Fig. 2E), the polymer film growth predominantly happened on the faces of the channel membrane and the inside of the channel was left empty without polymer growth. To the best of our knowledge, growth of a gas-responsive polymer film on a channel membrane has not yet been reported.
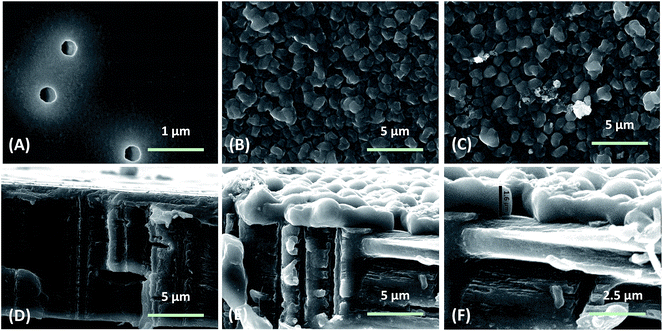 |
| Fig. 2 SEM images of cylindrical channels with a diameter of 200 nm before and after polymer film growth. A side view of the track-etched membrane (A), top and bottom views (B and C) of a cylindrical channel embedded in the PET membrane after polymer film growth, and cross sections of the cylindrical channel before (D) and after (E and F) polymer film growth. The thickness was measured to be around 1.6 μm. | |
The gas-responsive polymer film on the track-etched membrane exhibited repetitive switching between hydrophobicity and hydrophilicity (Fig. 3). Contact angle measurements (Fig. 3) illustrate that the contact angle of the water droplet on the nanopore surface showed a contact angle of 16.17° after the membrane grafted with a polymer film was immersed in a pH 5 buffer. Whereas, after the membrane was immersed in a pH 7 buffer and air dried, the contact angle increased to 54.71° (Fig. 3B). The contact angle could be further increased if a pH 9 solution was used. This result clearly manifested that protonation/deprotonation of the polymer film on the membrane can lead to a change in the hydrophilicity/hydrophobicity of the membrane surface. Instead of employing buffer solutions adjusted to certain pH values with NaOH, buffer solutions reversibly purged with CO2/N2 can also efficiently switch the membrane status between a big contact angle (57.50°) and a small contact angle (20.69°) (Fig. 3A).
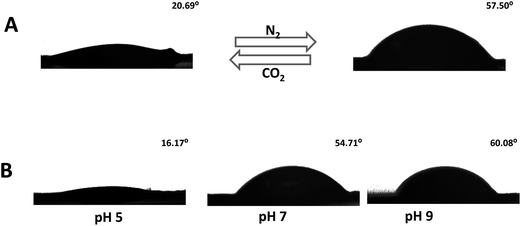 |
| Fig. 3 Contact angle measurements of the polymer film (PDEAEMA: poly 2-(diethylamino)ethyl methacrylate) grafted onto the channel membrane. (A) Measurements were carried out after the polymer film was immersed in neutral electrolyte solutions purged with CO2 and N2 for 30 minutes, respectively and was nitrogen dried. (B) Measurements were carried out after the polymer film was immersed in each electrolyte solution (pH 5, 7 and 9). 0.1 M PBS containing 0.1 M KCl was applied as the electrolyte solution. | |
A previous study19 proposed that toggling between hydrophobicity and hydrophilicity can play an extremely important role in the opening/closing of the channel on the basis of the pH-tuned polymer brush immobilized in the channel. Unambiguously, it has been clarified that conformation variation is a negligible factor which contributes to ion transport through this kind of channel. In contradiction to the immobilization of the polymer brush inside the channel, the present study entails only the growth of a gas-responsive polymer film on both sides of the track-etched membrane, which can fully prevent the leakage of the channel embedded in the membrane once the channel is switched to “off” status. Notably, several previous nanochannels with responsive properties are majorly related to the conformation switching of the monolayer immobilized inside the nanochannel surface. Obviously, this is not the same as our case. As shown in Fig. S4 (ESI†), in the presence of different pH values (5, 7 and 9), the morphology of the polymer film characterized using SEM did not show any distinct difference and the channel membrane was consistently covered by a continuous polymer film, which means that the conformation switching is not the important factor contributing to ion transport through the channel. Furthermore, the thickness of the polymer film was up to 1.6 μm, which was several times larger than the diameter of the channel embedded in the PET membrane.
In addition to the XPS and NMR characterization of the polymer film growth, each step in the modification process was confirmed and characterized with a current-versus-voltage curve (I–V curve). In this study, we present I–V curves for one cylindrical channel with a diameter of 200 nm and another conical-shaped channel with a tip diameter of 130 nm. The cylindrical channel in the PET membrane was fabricated under the same conditions as those in Fig. 2. The I–V curve (black curve in Fig. 4A) of the single cylindrical channel without any modification showed a linear shape without any rectification, which is measured in 100 mM PBS solution containing 1 M KCl at pH 3. Further tandem modification with branched PEI and an initiator did not exhibit much of a difference in the current-versus-voltage curves. Compared to the cylindrical channel, the asymmetric channel after PEI adsorption (red curve, Fig. 4C) presented an upward curve with strong rectification. For both the cylindrical and asymmetric channel, the polymer film growth fully blocked ion transport through the channel when measured in a pH 7 solution of 0.1 M KCl (curves in dark cyan, Fig. 4A and C).
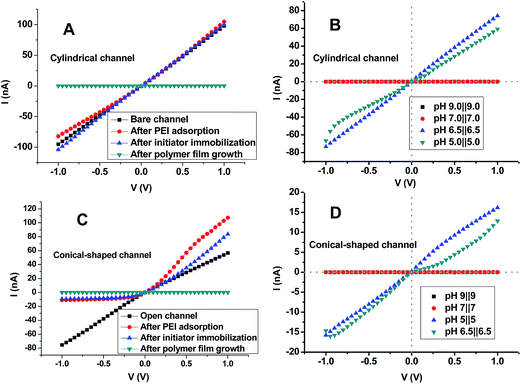 |
| Fig. 4 (A) I–V characterization of each modification step for the cylindrical channel with a diameter of 200 nm. (B) pH-dependent switching between the “on” status and “off” status of the cylindrical channel covered by a polymer film. (C) I–V characterization of each modification step for the conical-shaped channel with a tip diameter of 130 nm and a base diameter of 957 nm. (D) pH-dependent switching between the “on” status and “off” status of the conical-shaped channel covered by a polymer film. The electrolyte solutions for the experimental measurements in (A) and (C) are 0.1 M PBS containing 1 M KCl at pH 3; the buffer solutions for the experimental measurements in (B) and (D) are 0.1 M PBS containing 0.1 M KCl at pH 7. | |
Both the cylindrical and asymmetric channel covered with a gas-responsive polymer film can be switched on/off by using pH stimuli or gas inputs (Fig. 4B and D). Ion channel behaviors common to biological nanopores can be faithfully reproduced: when the pH value is above 7, the channel can be fully blocked in symmetric pH 7‖7 and 9‖9 conditions; when the pH value is decreased towards 6.5, the channel can be restored to an “on” status in symmetric pH 6.5‖6.5 and pH 5‖5 conditions. If the on/off current ratio was defined as the ratio of the current measured at pH 6.5‖6.5 to the current measured at pH 9‖9, the on/off current ratio corresponding to 1 V was calculated to be 34
418 for the cylindrical channel with a diameter of 200 nm. Furthermore, the current corresponding to the “off” status measured at 1 V was only 2.1 pA, which was defined as the leaking current. To the best of our knowledge, this is the biggest on/off current ratio and the lowest leakage current. As for the asymmetric channel with a tip diameter of 130 nm and a base diameter of 957 nm, the on/off current ratio and the leaking current were 6736 and 1.9 pA, respectively, which are calculated from the data in Fig. 4D. This is to say, the geometry of the channel was not a critical factor for determining the switching efficiency. The quality of the gas-responsive polymer film itself could play a crucial rule. The pH-adjusted ion transport through the channel, both sides of which were covered with a gas-responsive polymer film, was highly consistent with the pH-dependent switching between hydrophobicity and hydrophilicity.
Features belonging to an ionic diode can be exactly reproduced by this channel system symmetrically functionalized with the same gas-responsive polymer films. It has to be noted that previous ionic diodes based on a channel were almost accomplished via asymmetric functionalization with differently charged ligands or polymer brushes.13,14,16,44,45 In the current study, symmetric growth of the same gas-responsive film was executed on both sides of the channel. As shown in Fig. 5A, the upward curve with a nonlinear shape was divided into two parts including part I and part II. The corresponding cartoons about the ion transportation are also illustrated on the right hand side. When a pH 6.5 buffer solution was placed on the left side of the channel and the same solution but at pH 9 was placed on the right side of the channel, the voltage polarity decided the status of the channel. When a positive voltage was applied on the right side of the channel (part II in Fig. 5A), the slope of the I–V curve corresponded to an “on” status, which was exactly the same as the case when both sides of the channel were placed in buffer solutions at pH 6.5 (Fig. 4B). When a negative voltage was applied (part I), the channel was completely shut down. The “on” status and “off” status of the channel was exchangeable by swapping the position of the buffer solutions as shown in Fig. 5B.
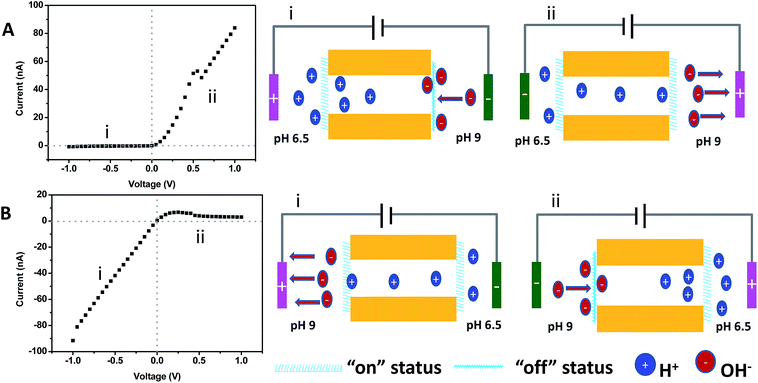 |
| Fig. 5 A switchable ionic diode pair (A and B) based on the same cylindrical channel covered by gas-responsive polymer films. Each curve is divided into part I and part II. The corresponding cartoons illustrate the ion transport mechanisms under pH stimulation and voltage polarity. When the OH− ions accumulate around the polymer film, this gate is shut down due to deprotonation and hydrophobicity; when the OH− ions decrease and H+ ions dominate around the polymer film, the gate opens up due to protonation and hydrophilicity. | |
Why did voltage polarity have such a significant impact on the ion transport through the channel when the channel with entrances covered by the same polymer film was stimulated under asymmetric pH conditions? To better understand this phenomenon, we have to understand the movement of ions under the applied voltage. When a negative voltage (part I in Fig. 5A) was applied in the chamber containing the buffer solution at pH 9, the negative ions such as OH− in the same chamber moved towards the polymer films and the concentration of OH− around the film increased, leading to closure of the polymer film due to deprotonation and hydrophobicity. When a positive voltage (part II in Fig. 5B) was applied in the chamber containing the buffer solution at pH 9, the negative ions such as OH− in the same chamber moved away from the polymer films and the concentration of OH− around the film decreased. Although the H+ ions in another chamber containing an electrolyte solution at pH 6.5 moved away from the polymer film adjacent to the chamber which contained the electrode applying the positive voltage, its concentration still overwhelms that of OH−, leading to the “on” status of the polymer film due to protonation of the polymer film and hydrophilicity. This similar mechanism can also be applied to explain the downward curve observed in Fig. 5B. Now, it is also very clear that by combining stimuli such as pH and gas with voltage polarity, each one of the two gates can be selectively shut down or opened up, which is the important prerequisite for construction of an ion pump with alternative gates and occluded states.
Once again, the features of this type of diode device which can be explained by the above mechanism can also be realized by an asymmetric channel with entrances covered by the same polymer film (Fig. S5 and S6, ESI†). Most interestingly, this kind of ionic device is not only pH-actuatable but is also highly CO2/N2-stimulable. By utilizing an asymmetric channel as a gas-responsive prototype, we carried out a series of experiments to challenge this kind of channel device with gas stimuli. Upon purging the buffer solution (0.1 M PBS, pH 7, 0.1 M KCl) with CO2, the pH value changed to nearly 5; by passing N2 into the solution, the pH value of 7 was recovered. Once the tip and base side solutions of the conical-shaped channel were purged with CO2 and N2, respectively, the I–V curve demonstrated extremely high rectification behavior and an unprecedented on/off current ratio with an upward nonlinear shape (Fig. 6A). One unique feature of this channel device is that the current rectification can be fully inversed. Upon purging N2 and CO2 into the tip and base side solutions of the same device, the downward I–V curve with excellent high rectification and on/off current ratio was obtained (Fig. 6B). The rectification inversion and current direction were highly reversible, which was only dependent on the gas pair (CO2‖N2 or N2‖CO2). Additionally, as shown in Fig. 6C and D, more than 5 switching cycles between the “on” status and “off” status have been presented in this study under the alternative stimulation with symmetric gas pairs (N2‖N2 and CO2‖CO2).
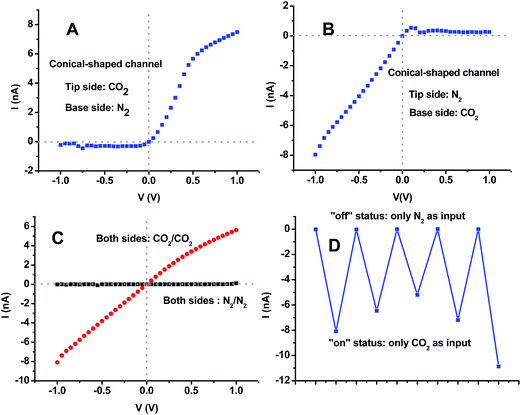 |
| Fig. 6 Green gas (CO2 and N2) breathing ionic diode (A and B) and ion channel (C). In case (A), the electrolyte solution on the tip side of the conical-shaped channel was purged with CO2 for 20 minutes and the base side electrolyte solution was purged with N2 for 20 minutes. In case (B), the electrolyte solution on the tip side of the conical-shaped channel was purged with N2 for 20 minutes and the electrolyte solution on the base side was purged with CO2 for 20 minutes. In case (C), electrolyte solutions on both sides were purged with CO2 or N2. (D) Repetitive switching between the “on” status and “off” status upon alternative purging with CO2 and N2: each point value was measured by applying a −1 V voltage. | |
In this paragraph, we would like to clarify that under the asymmetric stimulation, the competition between H+ and OH− plays a dominant role in the status of the polymer film. By using a conical-shaped channel as a prototype, we compared three different pH pairs (pH 5‖7, pH 6.5‖7, and pH 6.5‖9). As shown in Fig. S7,† only pH 6.5‖9 among the three pH pairs offered the opportunity to observe the ionic diode behavior: the “on” and “off” statuses are determined by voltage polarity. Fig. S7A and B† conclusively affirmed that H+ won and only the “on” status was present, which was indicated by the curved shape of the I–V curve. Therefore, the combination of voltage polarity and the appropriate pH pair is crucial for the opening and closing of each polymer film gate.
Conclusions
In summary, we describe a new ionic diode with both entrances covered by the same gas-responsive polymer films. Under symmetric stimulation with pH pairs (5‖5, 6.5‖6.5, 7‖7 and 9‖9) or with gas pairs (CO2‖CO2 and N2‖N2), features common to an ion channel can be faithfully reproduced including an “on” status and “off” status. Under asymmetric stimulation with gas pairs (CO2‖N2, N2‖CO2), an ionic diode based on gas-responsive polymer films supported on a channel membrane can be constructed with an unprecedented high rectification ratio of more than several thousands and the smallest leaking current at around several pA. Most importantly, the diameter and geometry of the channel are no longer the critical determining factors that influence the switching efficiency and contribute to current leakage, whereas the quality of the ionic device is more reliant on the polymer films. Except for the construction of an artificial ion channel and ionic diode, this new device can also be applied to build an ion pump since both gates of this device can be individually manipulated by a combination of gas pairs (or pH pairs) and voltage polarity. Therefore, the device developed herein holds great promise in further applications such as in the energy field, for sample filtration and seawater desalinization.
Acknowledgements
This work was supported by the National Natural Science Foundation of China (no. 21275137 and 21190040) and the Fundamental Research Funds of Shandong University (2015TB005).
References
- L. Sun, H. Wang, J. Hu, J. Han, H. Matsunami and M. Luo, Guanylyl cyclase-D in the olfactory CO2 neurons is activated by bicarbonate, Proc. Natl. Acad. Sci. U. S. A., 2009, 106, 2041–2046 CrossRef CAS PubMed.
- X. Wang, M. Zhong, Q. Liu, S. M. Aly, C. Wu and J. Wen, Molecular characterization of the carbon dioxide receptor in the oriental latrine fly, Chrysomya megacephala (Diptera: Calliphoridae), Parasitol. Res., 2013, 112, 2763–2771 CrossRef PubMed.
- J. Su, L. Yang, X. Zhang, A. Rojas, Y. Shi and C. Jiang, High CO2 chemosensitivity versus wide sensing spectrum: a paradoxical problem and its solutions in cultured brainstem neurons, J. Physiol., 2007, 578, 831–841 CrossRef CAS PubMed.
- K. Sharabi, C. Charar, N. Friedman, I. Mizrahi, A. Zaslaver, J. I. Sznajder and Y. Gruenbaum, The Response to High CO2 Levels Requires the Neuropeptide Secretion Component HID-1 to Promote Pumping Inhibition, PLoS Genet., 2014, 10, e1004529 Search PubMed.
- S. Lahiri and R. E. Forster, CO(2)/H(+) sensing: peripheral and central chemoreception, Int. J. Biochem. Cell Biol., 2003, 35, 1413–1435 CrossRef CAS.
- J. Y. Kwon, A. Dahanukar, L. A. Weiss and J. R. Carlson, The molecular basis of CO2 reception in Drosophila, Proc. Natl. Acad. Sci. U. S. A., 2007, 104, 3574–3578 CrossRef CAS PubMed.
- W. D. Jones, P. Cayirlioglu, I. G. Kadow and L. B. Vosshall, Two chemosensory receptors together mediate carbon dioxide detection in Drosophila, Nature, 2007, 445, 86–90 CrossRef CAS PubMed.
- F. Badsha, P. Kain, S. Prabhakar, S. Sundaram, R. Padinjat, V. Rodrigues and G. Hasan, Mutants in Drosophila TRPC Channels Reduce Olfactory Sensitivity to Carbon Dioxide, PLoS One, 2012, 7, e49848 CAS.
- S. Arioli, P. Roncada, A. M. Salzano, F. Deriu, S. Corona, S. Guglielmetti, L. Bonizzi, A. Scaloni and D. Mora, The relevance of carbon dioxide metabolism in Streptococcus thermophilus, Microbiology, 2009, 155, 1953–1965 CrossRef CAS PubMed.
- R. R. Geyer, R. Musa-Aziz, X. Qin and W. F. Boron, Relative CO2/NH3 selectivities of mammalian aquaporins 0-9, Am. J. Physiol.: Cell Physiol., 2013, 304, C985–C994 CrossRef PubMed.
- R. R. Geyer, R. Musa-Aziz, G. Enkavi, P. Mahinthichaichan, E. Tajkhorshid and W. F. Boron, Movement of NH3 through the human urea transporter B: a new gas channel, Am. J. Physiol. Ren. Physiol., 2013, 304, F1447–F1457 CrossRef CAS PubMed.
- N. Quoc Hung, M. Ali, R. Neumann and W. Ensinger, Saccharide/glycoprotein recognition inside synthetic ion channels modified with boronic acid, Sens. Actuators, B, 2012, 162, 216–222 CrossRef PubMed.
- H. Zhang, X. Hou, L. Zeng, F. Yang, L. Li, D. Yan, Y. Tian and L. Jiang, Bioinspired Artificial Single Ion Pump, J. Am. Chem. Soc., 2013, 135, 16102–16110 CrossRef CAS PubMed.
- W. Guo, Y. Tian and L. Jiang, Asymmetric Ion Transport through Ion-Channel-Mimetic Solid-State Nanopores, Acc. Chem. Res., 2013, 46, 2834–2846 CrossRef CAS PubMed.
- Z. Meng, H. Bao, J. Wang, C. Jiang, M. Zhang, J. Zhai and L. Jiang, Artificial Ion Channels Regulating Light-Induced Ionic Currents in Photoelectrical Conversion Systems, Adv. Mater., 2014, 26, 2329–2334 CrossRef CAS PubMed.
- X. Hou, W. Guo and L. Jiang, Biomimetic smart nanopores and nanochannels, Chem. Soc. Rev., 2011, 40, 2385–2401 RSC.
- M. Ali, B. Schiedt, R. Neumann and W. Ensinger, Biosensing with Functionalized Single Asymmetric Polymer Nanochannels, Macromol. Biosci., 2010, 10, 28–32 CrossRef CAS PubMed.
- S. N. Mubarak Ali, R. Patricio, A. Ishtiaq, H. N. Quoc, S. M. Ljiljana Fruk and W. Ensinge, Optical Gating of Photosensitive Synthetic Ion Channels, Adv. Funct. Mater., 2012, 22, 390–396 CrossRef PubMed.
- B. Yameen, M. Ali, R. Neumann, W. Ensinger, W. Knoll and O. Azzaroni, Synthetic Proton-Gated Ion Channels via Single Solid-State Nanochannels Modified with Responsive Polymer Brushes, Nano Lett., 2009, 9, 2788–2793 CrossRef CAS PubMed.
- W. Ruoshan, V. Gatterdam, R. Wieneke, R. Tampe and U. Rant, Stochastic sensing of proteins with receptor-modified solid-state nanopores, Nat. Nanotechnol., 2012, 7, 257–263 CrossRef PubMed.
- C. Han, H. Su, Z. Sun, L. Wen, D. Tian, K. Xu, J. Hu, A. Wang, H. Li and L. Jiang, Biomimetic Ion Nanochannelsas a Highly Selective Sequential Sensor for Zinc Ions Followed by Phosphate Anions, Chem.–Eur. J., 2013, 19, 9388–9395 CrossRef CAS PubMed.
- B. Yameen, M. Ali, M. Alvarez, R. Neumann, W. Ensinger, W. Knoll and O. Azzaroni, A facile route for the preparation of azide-terminated polymers. “Clicking” polyelectrolyte brushes on planar surfaces and nanochannels, Polym. Chem., 2010, 1, 183–192 RSC.
- M. Ali, S. Nasir, N. Quoc Hung, J. K. Sahoo, M. N. Tahir, W. Tremel and W. Ensinger, Metal Ion Affinity-based Biomolecular Recognition and Conjugation inside Synthetic Polymer Nanopores Modified with Iron-Terpyridine Complexes, J. Am. Chem. Soc., 2011, 133, 17307–17314 CrossRef CAS PubMed.
- J. E. Reiner, A. Balijepalli, J. W. F. Robertson, J. Campbell, J. Suehle and J. J. Kasianowicz, Disease Detection and Management via Single Nanopore-Based Sensors, Chem. Rev., 2012, 112, 6431–6451 CrossRef CAS PubMed.
- M. Puster, J. A. Rodriguez-Manzo, A. Balan and M. Drndic, Toward Sensitive Graphene Nanoribbon-Nanopore Devices by Preventing Electron Beam-Induced Damage, ACS Nano, 2013, 7, 11283–11289 CrossRef CAS PubMed.
- S. Liu, B. Lu, Q. Zhao, J. Li, T. Gao, Y. Chen, Y. Zhang, Z. Liu, Z. Fan, F. Yang, L. You and D. Yu, Boron Nitride Nanopores: Highly Sensitive DNA Single-Molecule Detectors, Adv. Mater., 2013, 25, 4549–4554 CrossRef CAS PubMed.
- Z. Guo, J. Wang, Y. Hu and E. Wang, Application of Biomimetic Nanopore Fabricated in Self-Supported Membrane in Analytical Chemistry, Progr. Chem., 2011, 23, 2103–2112 CAS.
- A. Mara, Z. Siwy, C. Trautmann, J. Wan and F. Kamme, An Asymmetric Polymer Nanopore for Single Molecule Detection, Nano Lett., 2004, 4, 497–501 CrossRef CAS.
- Z. Siwy, E. Heins, C. C. Harrell, P. Kohli and C. R. Martin, Conical-Nanotube Ion-Current Rectifiers:
The Role of Surface Charge, J. Am. Chem. Soc., 2004, 126, 10850–10851 CrossRef CAS PubMed. - B. Vilozny, P. Actis, R. A. Seger, Q. Vallmajo-Martin and N. Pourmand, Reversible Cation Response with a Protein-Modified Nanopipette, Anal. Chem., 2011, 83, 6121–6126 CrossRef CAS PubMed.
- S. Ding, C. Gao and L.-Q. Gu, Capturing Single Molecules of Immunoglobulin and Ricin with an Aptamer-Encoded Glass Nanopore, Anal. Chem., 2009, 81, 6649–6655 CrossRef CAS PubMed.
- M. R. Powell, L. Cleary, M. Davenport, K. J. Shea and Z. S. Siwy, Electric-field-induced
wetting and dewetting in single hydrophobic nanopores, Nat. Nanotechnol., 2011, 6, 798–802 CrossRef CAS PubMed.
- S. F. Buchsbaum, N. Gael, S. Howorka and Z. S. Siwy, DNA-Modified Polymer Pores Allow pH- and Voltage-Gated Control of Channel Flux, J. Am. Chem. Soc., 2014, 136, 9902–9905 CrossRef CAS PubMed.
- L.-X. Zhang, S.-L. Cai, Y.-B. Zheng, X.-H. Cao and Y.-Q. Li, Smart Homopolymer Modification to Single Glass Conical Nanopore Channels: Dual-Stimuli-Actuated Highly Efficient Ion Gating, Adv. Funct. Mater., 2011, 21, 2103–2107 CrossRef CAS PubMed.
- G. Wang, A. K. Bohaty, I. Zharov and H. S. White, Photon gated transport at the glass nanopore electrode, J. Am. Chem. Soc., 2006, 128, 13553–13558 CrossRef CAS PubMed.
- X. Hou, W. Guo, F. Xia, F.-Q. Nie, H. Dong, Y. Tian, L. Wen, L. Wang, L. Cao, Y. Yang, J. Xue, Y. Song, Y. Wang, D. Liu and L. Jiang, A Biomimetic Potassium Responsive Nanochannel: G-Quadruplex DNA Conformational Switching in a Synthetic Nanopore, J. Am. Chem. Soc., 2009, 131, 7800–7805 CrossRef CAS PubMed.
- W.-J. Lan, D. A. Holden and H. S. White, Pressure-Dependent Ion Current Rectification in Conical-Shaped Glass Nanopores, J. Am. Chem. Soc., 2011, 133, 13300–13303 CrossRef CAS PubMed.
- N. Liu, Y. Jiang, Y. Zhou, F. Xia, W. Guo and L. Jiang, Two-Way Nanopore Sensing of Sequence-Specific Oligonucleotides and Small-Molecule Targets in Complex Matrices Using Integrated DNA Supersandwich Structures, Angew. Chem., Int. Ed. Engl., 2013, 52, 2007–2011 CrossRef CAS PubMed.
- Y. Jiang, N. Liu, W. Guo, F. Xia and L. Jiang, Highly-Efficient Gating of Solid-State Nanochannels by DNA Supersandwich Structure Containing ATP Aptamers: A Nanofluidic IMPLICATION Logic Device, J. Am. Chem. Soc., 2012, 134, 15395–15401 CrossRef CAS PubMed.
- L. Cheng-Yong, M. Feng-Xiang, W. Zeng-Qiang, G. Hong-Li, S. Wen-Ting, W. Kang and X. Xing-Hua, Solution-pH-modulated rectification of ionic current in highly ordered nanochannel arrays patterned with chemical functional groups at designed positions, Adv. Funct. Mater., 2013, 23, 3836–3844 CrossRef PubMed.
- F. Xia, W. Guo, Y. Mao, X. Hou, J. Xue, H. Xia, L. Wang, Y. Song, H. Ji, O. Qi, Y. Wang and L. Jiang, Gating of single synthetic nanopores by proton-driven DNA molecular motors, J. Am. Chem. Soc., 2008, 130, 8345–8350 CrossRef CAS PubMed.
- S. Kumar, X. Tong, Y. L. Dory, M. Lepage and Y. Zhao, A CO2-switchable polymer brush for reversible capture and release of proteins, Chem. Commun., 2013, 49, 90–92 RSC.
- N. Sa and L. A. Baker, Rectification of Nanopores at Surfaces, J. Am. Chem. Soc., 2011, 133, 10398–10401 CrossRef CAS PubMed.
- E. B. Kalman, O. Sudre, I. Vlassiouk and Z. S. Siwy, Control of ionic transport through gated single conical nanopores, Anal. Bioanal. Chem., 2009, 394, 413–419 CrossRef CAS PubMed.
- G. Nguyen, I. Vlassiouk and Z. S. Siwy, Comparison of bipolar and unipolar ionic diodes, Nanotechnology, 2010, 21, 265301 CrossRef PubMed.
Footnote |
† Electronic supplementary information (ESI) available. See DOI: 10.1039/c5ra04712a |
|
This journal is © The Royal Society of Chemistry 2015 |