DOI:
10.1039/C5RA03834K
(Communication)
RSC Adv., 2015,
5, 27862-27866
Electrochemical potential-responsive tetra(aniline) nanocapsules via self-assembly
Received
4th March 2015
, Accepted 13th March 2015
First published on 13th March 2015
Abstract
A simple and effective approach is developed to obtain electroactive TANI nanocapsules via self-assembly. The carboxylic acid doped TANI molecules can self-assemble into nanocapsules through a simple dialysis process. The average diameter of the nanocapsules can be tuned using hydrophilic dopant acids with different alkyl lengths. Furthermore, those electroactive nanocapsules can be disrupted by applying a reducing voltage at −0.1 V.
Introduction
Recently, polymeric vesicles and nanocapsules prepared by self-assembly techniques have attracted considerable attention in the applications of active ingredient encapsulation and release, ranging from delivery and release of drugs or bioactive agents,1–3 diagnostic imaging,4 nanoreactors,5,6 electrical energy storage,7 and so on. One of the most attractive advantages of polymer vesicles and nanocapsules is their stimuli-responsive properties, including pH-,8 thermo-,9 redox-,10 photo-response,11 etc. Among them, the redox-responsive disruption or reorganization of polymeric vesicles and nanocapsules can be achieved by either the addition of redox chemical additives12 or the application of electric potentials.13 Compared with the former, electrical stimulus is more favorable for application in industry and biological systems without changing the chemical composition, concentration, volume, or temperature.
Oligo(aniline)s, as the good model compounds for studying the optical, electronic, magnetic and structural properties of polyaniline (PANI),14,15 have drawn increasing academic interest in the construction of responsive polymer vesicles and nanocapsules. This interest mainly stems from the fact that the oligo(aniline)s retain the unique redox chemistry of PANI and possess the relatively high conductivity.16 At the same time, the well-defined chemical structure of oligo(aniline)s provides molecular engineering possibilities for design and synthesis of multiblock polymers or oligomers with oligo(aniline)s sub-units exhibiting unique electrochemical redox property.17
Among these oligo(aniline)s, phenyl–NH2 end-capped tetra(aniline) (Ph/NH2 TANI) is the shortest oligomer that can represent the emeraldine oxidation state. Park and co-workers18 reported electrical switching between vesicles and micelles using redox-responsive self-assembly of an amphiphile, TANI block with poly(ethylene glycol). In aqueous solution, the self-assembled vesicles could be burst to form pucklike micelles by an oxidizing voltage at 0.2 V, while the collapsed micelles could regenerate vesicles upon the application of a reducing voltage at −0.5 V. This interesting electrochemical potential-responsive behavior could be attributed to the changes in packing density of TANI units upon oxidation to the emeraldine base (EB) state from the leucoemeraldine base (LEB) state. Another kind of voltage-responsive vesicle obtained from a diblock polymer, TANI block with poly (N-isopropylacrylamide), could be destructed completely by applying an oxidizing voltage at 0.6 V.19 A TANI-containing triblock, TANI–PEO–TANI, molecular architecture was also developed by Yang etc.20 This rod–coil–rod triblock copolymer exhibited pH-dependent self-assembly behavior in water to yield vesicles with a low conductivity. Another triblock oligomer containing TANI and ethylene could also self-assemble into electroactive vesicle in THF.21
By far, reports on the electroactive vesicles and nanocapsules mainly use the self-assembly of multiblock polymers or oligomers. Unfortunately, the complex procedures for the synthesis of copolymer and the attachment of large structural (i.e., functionless) blocks diluting the electroactive functionality within the bulk material make it difficult to achieve its potential application requiring a high density of electroactivity.22 Besides, few articles focus on that the amphiphilic nature of doped TANI molecules can make it possible to self-assemble into electroactive nanocapsules. We show that electroactive liquid core–TANI shell microcapsules can be obtained via the self-assembly of carboxylic acid doped TANI molecules on the interface of oil–water droplet in our previous report.23 In this communication, according to the amphiphilic nature of doped Ph/NH2 TANI, we report that the carboxylic acid doped TANI molecules can self-assemble into the electrochemical nanocapsules directly through a simple dialysis process without the addition of the oil. The average size of nanocapsules is dependent on the length of alkyl chain in the doped acid. Also, the effect of voltage on the electroactive nanocapsules is investigated.
Experimental section
Synthesis of TANI
N-Phenyl-1,4-phenylenediamine (98%) was purchased from Aldrich chemical Co. Ltd. and other chemicals were from Tianli Chemical Reagent Co. Ltd. All reagents were used as received. The synthetic route of Ph/NH2 TANI in emeraldine base (EB) state (TANI-EB) has been reported in our previous literature.23 MALDI-TOF-MS m/z: [M + H]+ calcd for C24H20N4, 366.2; found: 366.14. IR (KBr): 3373 (s, νN–H), 3023 (m, νC–H), 1593 (s, νC
C of quinone rings), 1502 (s, νC
C of benzenoid rings), 1301 (s, νC–N), 815 (m, δC–H), 746 (m, δC–H).
Electroactive TANI nanocapsules via self-assembly
In a typical preparation process, 2.0 mg of fine TANI-EB powder was dispersed in 4.0 ml of glacial acetic acid (HAc) under ultrasonic irradiation for 5 min. Then the mixture was centrifuged at 4000 rpm for 5 min. The supernatant was collected and placed into a dialysis bag (3500 MW cutoff, Viskase). The sealed bag was suspended within a 1000 mL beaker containing deionized water under stirring to allow the acid to diffuse out of the dialysis bag until the pH of the outer deionized water stabilized at 7. The outer deionized water was renewed every 2 h for the first 10 h and then every 7 h for the remaining period of time.
Characterization
Matrix-assisted laser desorption ionization time-of-fight mass spectrometry analysis (MALDI-TOF MS) was performed on an Applied Biosystems 4700 Proteomics Analyzer. UV-Vis spectra were recorded on an Agilent 8453 instrument. FT-IR spectra of the dried samples were obtained on a Bruker, TENSOR37 infrared spectrometer with KBr pellets. Dynamic light scattering (DLS) and zeta potential measurements were carried out by using a Malvern Zetasize Nano ZS90 apparatus. SEM images were taken in JEOL JSM-6390A Field Emission Scanning Electron Microscope. The samples were prepared by casting a drop of the final solution on a silicon wafer. Transmission electron microscopy (TEM) was performed on a JEM model 2100 electron microscope. The samples were prepared by drop-casting 1 to 2 drops of the nanocapsules dispersion onto the carbon-coated copper grids and allowed to dry in air. Cyclic voltammetry (CV) was performed on a CHI 660D electrochemical work station with a conventional three electrode cell, using Ag/AgCl (3 M KCl) as the reference electrode and Pt foil as the counter electrode. The working electrode was prepared by simple dropping the final vesicles solution onto the surface of the FTO glass. The loading of TANI vesicles solution on the FTO glass was 0.3 ml cm−2. The FTO glass was obtained from Pilkington (Toledo, USA) (14 Ohm per square, thickness of 2.2 mm). CV investigations were carried out in 1.0 M H2SO4 solutions (degassed for 30 minutes with high purity N2) in the range from −0.2 to 1.0 V at a scan rate of 100 mV s−1.
Results and discussion
Electroactive TANI nanocapsules via self-assembly
As shown in Fig. 1, the as-synthesized TANI-EB powder with aggregated morphology (Fig. 1a) dissolved in HAc assembled into vesicles with average diameter of 250 nm by dialyzing the mixture against deionized water. The broken nanocapsules observed in SEM images (the arrows in Fig. 1b) and the strong contrast between the pale edge and the dark center in the TEM image (Fig. 1c) are evidence for their hollow nature. The average walls of nanocapsules are approximately 30 nm thick. Those nanocapsules collapse occurred in thinner shell area when exposed to high vacuum and energy during SEM and TEM characterization (Fig. 1b and c and 5b and c).24 Therefore, it seems that the size in SEM images is slightly larger than that of hydrodynamic radius determined from DLS (Fig. 1d).
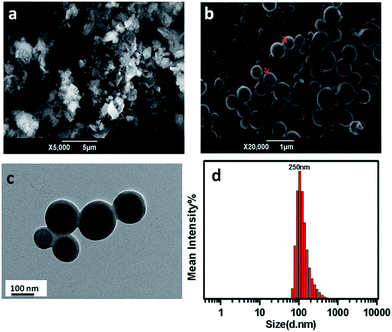 |
| Fig. 1 Scanning electron microscope (SEM) images of the as-synthesized TANI-EB powder (a) and the obtained nanocapsules (b), TEM image of the obtained nanocapsules (c) and particle size distribution (d) of the final TANI nanocapsules. | |
In Fig. 2, the CV curve obtained from the bare FTO glass electrode exhibits no obvious peaks, while the CV curve of the obtained nanocapsules shows two stronger redox processes. There are two reduction peaks at 0.51 V and 0.21 V vs. Ag/AgCl, which are attributed to the transition from pernigraniline (PA) oxidation state to EB state and EB state to LEB state, respectively.25 This indicates that the obtained nanocapsules exhibit the unique redox activity which is similar to that expected for simple TANI materials.16
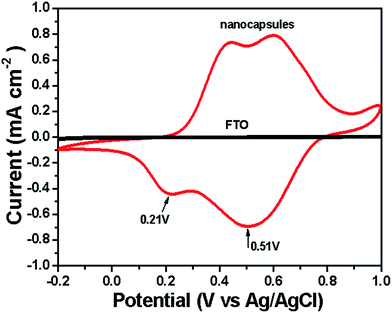 |
| Fig. 2 Cyclic voltammetry of bare FTO glass and the final TANI nanocapsules in 1.0 M H2SO4. | |
Proposed formation mechanism of the electroactive TANI nanocapsules
A possible formation mechanism of the electroactive nanocapsules is shown in Fig. 3. TANI-EB is soluble in HAc and can be doped in this environment at the same time. Some of the formed HAc-doped TANI precipitated from solution, which was removed by centrifugation. The remaining homogeneous solution (HAc-doped TANI dissolved in the excess HAc, Fig. 3A) was placed in the dialysis bag. During the dialysis process, the excess HAc was replaced with water, resulting in the formation of nanocapsules (Fig. 3B). The driven forces for the self-assembly can be divided into three parts. Firstly, due to its hydrophilic –COOH groups from HAc and its hydrophobic conjugated backbone of TANI, the HAc-doped TANI can form micelle.26,27 Under the slow exchange of HAc with the water, the hydrophilic environment promotes aggregation among the hydrophobic conjugated backbone to produce self-assembly, whereas the hydrophilic –COOH groups are solvated by the hydrophilic solvent. The resulting aggregated regions of the TANI conjugated backbone are possibly configured at the core of the self-assembled structure. Secondly, driven by π–π stacking interactions, those hydrophobic conjugated backbones interact with each other to self-assemble into the shell of nanocapsules (Fig. 3C).23,28–30 It can be seen from Fig. 4A, the πB–πQ absorption of the final nanocapsules is red shifted by Δλ = 16 nm compared with that of as-synthesized TANI, indicating the existence of strong π–π stacking interaction.21,23 Besides, the electrostatic interactions introduced by the dopants can also serve as one of the important driving forces for the self-assembly.31 As shown in Fig. 4B, a strong peak attributed to the stretching vibrations of the carbonyl group is located at 1662 cm−1 in the pure HAc-doped TANI, which is shifted to 1708 cm−1 in the final nanocapsules.32 This indicates that the carboxylic groups exist in the final TANI molecules. Thus, the carboxylic ions act as dopant counter ions that reside near the positively charged nitrogen atoms during the dialysis process, leading to the formation of electrostatic interactions. When the dialysis finished, the TANI in emeraldine salt (ES) state was dedoped into EB state (Fig. 3D), as the UV-Vis and FT-IR characterization of the final nanocapsules shown in Fig. 4. Interesting, the carbonyl groups still exist in the molecular structure of final nanocapsules. Combined with the shift in the C
O stretching frequency in FT-IR spectra, we deduce that the double bond of the carbonyl groups are in conjugation with π-bonded system of TANI.33
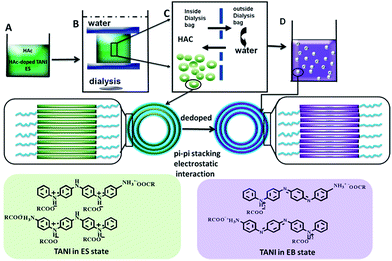 |
| Fig. 3 Schematic of electroactive TANI nanocapsules formation by the self-assembly process of the amphiphilic HAc-doped TANI molecules. | |
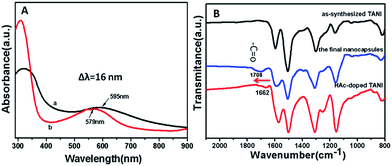 |
| Fig. 4 (A) UV-Vis spectra of (a) the final nanocapsules, and (b) as-synthesized TANI dissolved in dimethyl sulfoxide without the solvatochromism effect on the πB–πQ absorption peak: in the UV-Vis spectra of final nanocapsules, the main characteristic bands located at 317 nm and 591 nm are ascribed to π–π* transitions of the benzene ring and the benzenoid to quinid (πB–πQ) excitonic transition in TANI-EB, respectively;21 (B) FT-IR spectra of the as-synthesized TANI-EB powder, the final nanocapsules and pure HAc-doped TANI: the main peaks in the FT-IR spectra of the final nanocapsules located at 1593 and 1502 cm−1 are corresponded to C C stretching vibrations of quinone and benzene ring in TANI-EB, respectively.21 | |
Size control of the nanocapsules
The diameter of the aggregates assembled nanocapsules can be readily tuned using different alkane length of carboxylic acid which is related to hydrophobicity of the dopant aliphatic chain. The average diameter of nanocapsules increases with the increase in the length of hydrophilic dopant acid (Fig. 5). The average diameter of nanocapsules obtained using acetic acid is around 250 nm. When propionic acid was used, nanocapsules with an average diameter of 325 nm were well dispersed in solution. The average diameter of nanocapsules furthermore increased to 483 nm when butyric acid was the dopants. This interesting phenomenon further supports the mechanism proposed above.
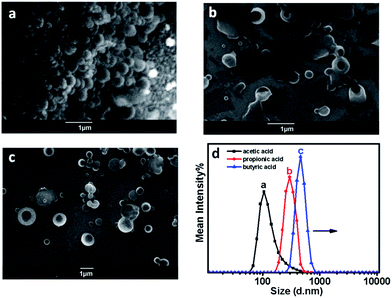 |
| Fig. 5 SEM images and particle size distributions of nanocapsules prepared using acetic acid (a), propionic acid (b) and butyric acid (c) as dopants. | |
Electrochemical potential-responsive property of TANI nanocapsules
The electrochemical-responsive behavior of TANI nanocapsules were further investigated in both NaCl and H2SO4 aqueous solution. The final TANI nanocapsules were casted on the surface of FTO glass as the working electrode, Ag/AgCl as the reference electrode and Pt foil as the counter electrode. Vred (−0.1 V) was the static potential applied to the aqueous for 4 h. As we can see from the SEM images in Fig. 6, the nanocapsules being electrochemically reduced in both NaCl and H2SO4 aqueous were destroyed and partially collapsed to form disk aggregates (Fig. 6C and D). Literature report indicates that the conductivity of corresponding electrolytes system, i.e., the individual ions, plays a curial role in electrochemical response.34 It means that the doping of H+ makes TANI more electroactive than Na+, resulting in the differences of CV curves obtained in two different electrolytes. Moreover, TANI exhibits unique reversibly oxidation–reduction chemistry property in acid solution.14,16 Therefore, there are two redox pairs in the CV curve of the obtained nanocapsules in H2SO4 solution but one pair in NaCl electrolyte. Interestingly, a new irreversible oxidation peak (0.098 V in NaCl aqueous and 0.257 V in H2SO4 aqueous) appears in both CV curves obtained using two different electrolytes, which might due to the reduction of TANI nanocapsules. However, this new band could not be attributed to the transition from LEB state to EB state because there are still two pairs of redox peaks in H2SO4 aqueous (Fig. 6B). Unfortunately, there are no reports on this peak in the electrochemistry of oligo(aniline)s, and the possible mechanism is under investigation.
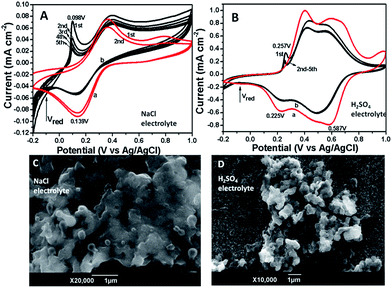 |
| Fig. 6 CV curves of final TANI nanocapsules before (a) and after (b) being electrochemically reduced at −0.1 V for 4 h in 0.5 M NaCl (A) and 1.0 M H2SO4 (B) electrolyte; SEM images of TANI nanocapsules after being electrochemically reduced at −0.1 V for 4 h in 0.5 M NaCl (C) and 1.0 M H2SO4 (D). | |
Conclusions
In summary, novel electroactive TANI nanocapsules can be obtained via self-assembly during a simple dialysis process. The amphiphilic feature, π-conjugation and electrostatic interactions of carboxylic acid doped TANI molecules make them self-assemble into nanocapsules for slow exchange of carboxylic acid with the water. The morphology of the obtained nanocapsules can be controlled by the alkyl length of carboxylic acid. In particular, without using any chemical additives, the electrochemical potential response makes nanocapsules a potential carrier for the burst release. Besides, this new strategy for electroactive nanocapsules via self-assembly may be applied to other conducting oligomers like oligo(pyrrole)s.
Acknowledgements
The authors gratefully acknowledge the financial supports from the National Natural Science Foundation of China (Grant no. 21307098) and the Fundamental Research Funds for the Central Universities of China (2011JDGZ15).
Notes and references
- D. E. Discher and F. Ahmed, Annu. Rev. Biomed. Eng., 2006, 8, 323 CrossRef CAS PubMed.
- F. Ahmed, R. I. Pakunlu, A. Brannan, F. S. Bates, T. Minko and D. E. Discher, J. Controlled Release, 2006, 116, 150 CrossRef CAS PubMed.
- D. A. Christian, S. Cai, D. M. Bowen, Y. Kim, J. D. Pajerowski and D. E. Discher, Eur. J. Pharm. Biopharm., 2009, 71, 463 CrossRef CAS PubMed.
- R. J. Hickey, A. S. Haynes, J. M. Kikkawa and S. J. Park, J. Am. Chem. Soc., 2011, 133, 1517 CrossRef CAS PubMed.
- P. Broz, S. Driamov, J. Ziegler, N. Ben-Haim, S. Marsch, W. Meier and P. Hunziker, Nano Lett., 2006, 6, 2349 CrossRef CAS PubMed.
- K. T. Kim, J. J. L. M. Cornelissen, R. J. M. Nolte and J. C. M. van Hest, Adv. Mater., 2009, 21, 2787 CrossRef CAS.
- J. C. Kelly, M. Pepin, D. L. Huber, B. C. Bunker and M. E. Roberts, Adv. Mater., 2012, 24, 886 CrossRef CAS PubMed.
- S. Yu, T. Azzam, I. Rouiller and A. Eisenberg, J. Am. Chem. Soc., 2009, 131, 10557 CrossRef CAS PubMed.
- Y. Li, B. Lokitz and C. L. McCormick, Angew. Chem., Int. Ed., 2006, 45, 5792 CrossRef CAS PubMed.
- S. Cerritelli, D. Velluto and J. A. Hubbell, Biomacromolecules, 2007, 8, 1966 CrossRef CAS PubMed.
- X. Liu and M. Jiang, Angew. Chem., Int. Ed., 2006, 45, 3846 CrossRef CAS PubMed.
- J. Z. Du and R. K. O'Reilly, Soft Matter, 2009, 5, 3544 RSC.
- A. C. Feng and J. Y. Yuan, Macromol. Rapid Commun., 2014, 35, 767 CrossRef CAS PubMed.
- Z. Shao, P. Rannou, S. Sadki, N. Fey, D. M. Lindsay and C. F. J. Faul, Chem.–Eur. J., 2011, 17, 12512 CrossRef CAS PubMed.
- Z. Wei, T. Laitinen, B. Smarsly, O. Ikkala and C. F. J. Faul, Angew. Chem., Int. Ed., 2005, 44, 751 CrossRef CAS PubMed.
- Y. Wang, H. D. Tran, X. F. Duan and R. B. Kaner, J. Am. Chem. Soc., 2010, 132, 10365 CrossRef CAS PubMed.
- C. U. Udeh, N. Fey and C. F. J. Faul, J. Mater. Chem., 2011, 21, 18137 RSC.
- H. Kim, S. M. Jeong and J. W. Park, J. Am. Chem. Soc., 2011, 133, 5206 CrossRef CAS PubMed.
- Y. P. Wu, S. W. Liu, Y. C. Tao, C. P. Ma, Y. Zhang, J. R. Xu and Y. Wei, ACS Appl. Mater. Interfaces, 2014, 6, 1470 CAS.
- Z. Yang, X. Wang, Y. Yang, Y. Liao, Y. Wei and X. Xie, Langmuir, 2010, 26, 9386 CrossRef CAS PubMed.
- C. U. Udeh, P. Rannou, B. P. Brown, J. O. Thomas and C. F. J. Faul, J. Mater. Chem. C, 2013, 1, 6428 RSC.
- Z. Wei and C. F. J. Faul, Macromol. Rapid Commun., 2008, 29, 280 CrossRef CAS.
- W. Lv, J. T. Feng, W. Yan and C. F. J. Faul, J. Mater. Chem. B, 2014, 2, 4720 RSC.
- A. Loxley and B. Vincent, J. Colloid Interface Sci., 1998, 208, 49 CrossRef CAS PubMed.
- Y. N. Xia, J. M. Wiesinger, A. G. Macdiarmid and A. J. Epstein, Chem. Mater., 1995, 7, 443 CrossRef CAS.
- Z. X. Wei, Z. M. Zhang and M. X. Wan, Langmuir, 2002, 18, 917 CrossRef CAS.
- L. J. Zhang and M. X. Wan, Adv. Funct. Mater., 2003, 13, 815 CrossRef CAS.
- Y. Yan, R. Wang, X. H. Qiu and Z. X. Wei, J. Am. Chem. Soc., 2010, 132, 12006 CrossRef CAS PubMed.
- Y. Zhao, E. Tomsik, J. Wang, Z. Moravkova, A. Zhigunov, J. Stejskal and M. Trchova, Chem.–Asian J., 2013, 8, 129 CrossRef CAS PubMed.
- Y. Zhao, J. Stejskal and J. Wang, Nanoscale, 2013, 5, 2620 RSC.
- Y. Wang, J. Liu, H. D. Tran, M. Mecklenburg, X. N. Guan, A. Z. Stieg, B. C. Regan, D. C. Martin and R. B. Kaner, J. Am. Chem. Soc., 2012, 134, 9251 CrossRef CAS PubMed.
- D. W. Hatchett, M. Josowicz and J. Janata, J. Phys. Chem. B, 1999, 103, 10992 CrossRef CAS.
- I. Šeděnková, M. Trchová and J. Stejskal, Polym. Degrad. Stab., 2008, 93, 2147 CrossRef PubMed.
- S. Chaudhari, Y. Sharma, P. S. Archana, R. Jose, S. Ramakrishna, S. Mhaisalkar and M. Srinivasan, J. Appl. Polym. Sci., 2013, 129, 1660 CrossRef CAS.
|
This journal is © The Royal Society of Chemistry 2015 |