DOI:
10.1039/C5RA01507C
(Paper)
RSC Adv., 2015,
5, 48769-48778
Nutrimetabolomic analysis provides new insights into spermine-induced ileum-system alterations for suckling rats
Received
26th January 2015
, Accepted 20th May 2015
First published on 20th May 2015
Abstract
This study aimed to investigate the effects of spermine supplementation on the ileum metabolism of suckling rats. Rats were intragastrically administered with 0.2 μmol g−1 body weight of either spermine or saline for 3 or 7 days. The ileum samples taken 24 h after the last spermine ingestion were analyzed by NMR-based metabolomics. The results showed that the villus width, surface area, and crypt depth of the rat ileum were increased by 18.0%, 33.3%, 15.7%, respectively, and the specific activities of sucrase and maltase in the rat ileum were also significantly increased by 789.4%, 44.9%, respectively, but the activity of lactase and alkaline phosphatase were decreased by 49.9%, 35.5%, respectively (spermine group relative to control group) and the villus width, surface area, and crypt depth of the rat ileum were increased by 15.1%, 33.3%, 26.3%, respectively [duration extension of spermine (7 days) relative to 3 days] (P < 0.05). Spermine also increased ileum choline, amino acids, inosine, lactate, myo-inositol, scyllo-inositol, and trimethylamine-N-oxide levels and decreased the level of lysine (P < 0.05). The ileum levels of α-glucose, aspartate, creatine, D-galactose, formate, glutathione, glycerolphosphocholine, lipid, and tyrosine were also significantly affected by spermine supplementation (P < 0.05). Collectively, spermine ingestion induces the morphological and common systemic metabolic modifications characterizing the ileum postnatal maturation suggesting the important role of spermine. The metabolic variations could be attributed to functional variations in lipid metabolism, energy metabolism, amino acid metabolism, gut microbial activity, osmoregulation, and oxidative protection.
Introduction
Spermine is a ubiquitous cellular component that plays important roles in cell physiological processes, such as maintenance of the structure and stability of cellular macromolecules (e.g., nucleic acids), gene expression, modulation of kinase activities, protein synthesis, control of ion channel activities, and protection from oxidative damage.1 Spermine is also necessary to facilitate functioning and renewal of the gut epithelium. Spermine can alleviate intestinal dysfunction and improve immune function in animals.2–5 For instance, suckling rodents that orally ingest spermine exhibit structural and biochemical alterations in the small intestine, which are characteristic of the natural postnatal intestinal maturation process that is observed at weaning. This type of maturation is reflected by morphological, enzymatic, and physiological modulations.6 Furthermore, spermine-induced intestinal maturation is a two-step event. In the first step, which occurs fast and transiently, immature enterocytes found in the tip of the villus are eliminated. In the second step, which occurs longer than the first step, immature cells are replaced with adult-type enterocytes.7 In a time-course analysis of biochemical and histological modifications occurring after single dose of spermine is ingested, spermine remarkably changes mucosal integrity without disrupting the epithelium from 4 h to 10 h after supplementation is administered.8 Mucosal function is also impaired by reduced lactase- and maltase-specific activities because of cell loss by apoptosis.9,10 Intestinal weight is also significantly reduced by spermine.9 Between 30 and 40 h after spermine is administered, intestinal weight and maltase-specific activities are recovered, and sucrase-specific activities can be found in the jejunum and the ileum.9 Histological studies have shown that the mucosa completely regenerates 48 h after spermine is administered. Large supranuclear vacuoles disappear from the ileum.8 Spermine-induced maturation is also reversible when administration is terminated 2 days to 3 days after the end of treatment. Furthermore, sucrase- and maltase-specific activities revert to a typical “immature” status when spermine treatment is extended (0 days to 4 days) after spermine-induced maturation occurs; at the same time, mucosal weight and lactase-specific activities remain at typical “mature” levels.7 Spermine can indirectly induce postnatal intestinal development through secondary effectors such as corticoids and insulin, gastrointestinal hormones, or a cytokine-dependent mechanism.11–13 However, intestinal health effects and exact action mechanisms of spermine supplementation remain largely unknown.
Nevertheless, many studies on spermine supplementation have been performed by measuring and comparing single or several biochemical marker(s), which do not sufficiently reflect the overall metabolic status of animal or human gastrointestinal tract. These parameters are pre-selected on the basis of some general and expected hypotheses and then verified. Thus, a robust technique that can simultaneously quantify and identify a large number (hundreds to thousands) of molecules is necessary. Nutrimetabolomics offers a novel strategy to determine changes in metabolic endpoints of physiological regulatory processes of an organism after specific nutritional interventions are administered. However, few studies have focused on the response of animal or human intestine biological systems to spermine supplementation. This experiment aimed to investigate the effect of spermine administration on the ileum compositions of rats by using an explorative metabolomic approach with 1H NMR spectroscopy and chemometrics.
Materials and methods
Animal experiment and sample collection
The experimental protocol was approved by the Animal Care and Use Committee of the Animal Nutrition Institute of Sichuan Agricultural University and was conducted according to the Guide for the Care and Use of Laboratory Animals of the National Research Council. A total of forty rats were divided into four groups (ten rats per group) in this study. Four groups are control-3 days (group 1), and control-7 days (group 2), spermine-3 days (group 3), spermine-7 days (group 4). Ten litters from each of the four male pups were used. Mothers were fed ad libitum with food and drinking water. Only male rats were used in the experiments to avoid excessive body weight (BW) differences (females are usually smaller than males) and the possible influence of these differences in hormonal status. The day of birth was designated as 0 day. The age of the pups at the beginning of the experiment was 8 days. In each litter, two animals received intragastric administration of 0.2 μmol per g BW of spermine (Sigma Chemical Co., St. Louis, M.O., USA; spermine was dissolved in physiological saline). The remaining pups from the same litter were used as control animals in the same way and received vehicle (physiological saline). Treatment was repeated daily until the animals were sacrificed 3 or 7 days after the beginning of the treatment. At this time point, the pups were 11 or 15 days old. The ileum was then harvested. A small piece from the ileum was prepared for histological analysis. The remaining pieces of the ileum sample were stored at −80 °C for NMR spectroscopic and enzymatic analyses. The dosage for this study was selected on the basis of a previous experiment.7,14,15 The rats were maintained at 24 ± 1 °C with a 12
:
12 hour light-to-dark cycle and from 50% to 70% humidity throughout the study. Clinical observations were achieved during the experimental period.
Ileum morphology study
The ileum samples were flushed with physiological saline and stored in 10% neutral buffered formalin. The preserved intestinal segments were prepared using standard paraffin-embedding techniques. Cross-sections at 5 μm thickness of each intestinal segment were stained with haemotoxylin and eosin.
A total of 10 intact, well-oriented crypt-villus units were selected in triplicate for each intestinal cross-section. Villus height was measured from the tip of the villi to the villus crypt junction, and crypt depth was defined as the depth of the invagination between adjacent villi. Morphological indices were determined using image processing and analysis system (Image Pro Plus, Media Cybernetics, Bethesda, MD, USA).
Ileum protein content and analysis of disaccharidases
The protein content of the homogenates was measured by Bradford's method.16 Sucrase, lactase, and maltase activities were assayed according to Dahlqvist (1968).17
Sample preparation and NMR spectroscopy
Ileum tissues (approximately 60 mg) were extracted with 0.6 mL of pre-cooled aqueous methanol (methanol–water = 2
:
1) by using a tissue-lyser (Next Advance Bullet Blender, America) for 30 s. The homogenate mixture was then sonicated in an ice bath to further break the cells for 3 min. The supernatant was obtained after the mixture was centrifuged at 14
500×g for 10 min at 4 °C. The whole extraction was repeated twice, and the three supernatants were combined and lyophilized after methanol was removed in vacuo. The extract was then reconstituted into 600 μL of phosphate buffer (0.1 M NaH2PO4/K2HPO4, pH 7.4, 50% v/v D2O) containing 5.0 mM 2,2-dimethyl-2-silapentane-5-sulfonate-d6 (DSS) as a chemical shift reference (δ0.00 ppm). A total of 550 μL of this solution was transferred to 5 mm NMR tubes after centrifugation was performed (at 12
000×g for 10 min at 4 °C). The proton NMR spectra of the ileum samples were obtained at 300 K on a Bruker Avance II 600 MHz spectrometer (Bruker Biospin, Rheinstetten, Germany) operating at a 1H frequency of 600.13 MHz with a broadband-observe probe. A standard water-suppressed one-dimensional NMR spectrum was obtained for ileum by using the first increment of the gradient-selected NOESY pulse sequence (recycle delay-90°-t1-90°-tm-90°-acquire data) with a recycle delay of 2 s, t1 of 3 μs, mixing time (tm) of 100 ms, and 90° pulse length of 13.70 μs. A total of 128 transients were collected into 49
178 data points with a spectral width of 9590 Hz and an acquisition time of 3.42 s. Metabolite assignments were usually obtained by considering chemical shifts, coupling constants, and relative intensities as in previous reports and additional 1H–1H correlation spectroscopy and 1H–1H total correlation spectroscopy were acquired for selected samples (data not shown).
NMR spectroscopic processes and analysis
All of the NMR spectra were processed employing Mestrenova 8.1.2 software (Mestrelab Research S.L., Spain). Free induction decays were multiplied by an exponential window function with a 1 Hz line-broadening factor prior to Fourier transformation was performed. The NMR spectra were then manually phase- and baseline-corrected with the chemical shift referenced to the peak of DSS at δ0.00. The ileum spectral region of δ0.7 to δ9.5 was then integrated into regions with an equal width of 0.002 ppm by using Mestrenova 8.1.2 software (Mestrelab Research S.L., Spain). Methanol signals, together with the regions containing H2O signals, were carefully excluded to obtain only the endogenous metabolite changes that were induced by treatment. This treatment helps avoid any contributions of methanol and H2O to intergroup differentiations. The discarded regions included δ4.54 to δ5.24 for H2O and δ1.15 to δ1.20 and δ3.56 to δ3.63 for methanol. The integrals of the remaining bins were normalized to the total sum of all of the integral regions for each spectrum prior to pattern recognition analysis.
Multivariate data analysis was carried out on normalized NMR data sets with the software package SIMCA-P+ (version 11.0, Umetrics, Sweden). Principal component analysis (PCA) was achieved using the mean-centered NMR data to generate an overview. Results were viewed in the form of score plots, where each point represented an individual sample, and loading plots, where each coordinate represented one NMR spectral region. Projection to latent structure-discriminant analysis (PLS-DA) and orthogonal projection to latent structure-discriminant analysis (OPLS-DA) were conducted using NMR data (scaled to unit variance) as the X-matrix and class information as the Y-matrix.18 The quality of the model was validated by the parameters R2X, representing the total explained variation, and Q2 representing model predictability. The models were evaluated using a seven-fold cross validation method and a permutation test.19,20 To interpret our results, we used the OPLS-DA models as shown by coefficient-coded loading plots. The loadings were backtransformed in Excel (Microsoft, USA) and plotted with color-coded absolute coefficient values (|r|) of variables in MATLAB (version 7.1, The Mathworks Inc., Natwick, USA).19 The coefficient plot demonstrated the significance of variables (resonances) that contributed to the differentiation of classes of interest in which red was considered more significant than blue. In the current study, appropriate correlation coefficients were used as cutoff values (depending on the number of animals used for each group) to determine the statistical significance on the basis of the discrimination significance (P < 0.05). These coefficients were determined according to Pearson's product-moment correlation coefficient.19
Statistical analysis
Ileum morphology and enzymatic parameters of suckling rats were analyzed statistically by two-way ANOVA by using the general linear model procedure of SPSS 17.0 software (SPSS Inc., Chicago, IL). Model main effects included spermine levels (0, 0.2 μmol per g BW) and treatment time levels (3, 7 days). Data were presented as mean ± SEM. The level of significance used was P < 0.05.
Results
Morphology and enzymatic parameters
The villus width, surface area, and crypt depth of rat ileum were increased by 18.0%, 33.3%, 15.7%, respectively (spermine group relative to control group) and the villus width, surface area, and crypt depth of rat ileum were increased by 15.1%, 33.3%, 26.3%, respectively [duration extension of spermine (7 days) relative to 3 days] (P < 0.05; Table 1 and Fig. 1). The specific activities of sucrase and maltase in rat ileum were also significantly increased by 789.4%, 44.9%, respectively, but the activity of lactase and alkaline phosphatase were decreased by 49.9%, 35.5%, respectively (spermine group relative to control group) (P < 0.05; Table 2). The activity of lactase, sucrase, maltase and alkaline phosphatase in rat ileum were not affected by duration extension of spermine (7 days) relative to 3 days (P > 0.05; Table 2).
Table 1 Effects of spermine and its duration extension on ileum morphology of suckling ratsa
Groups |
Spermine (μmol per g BW) |
Time extension of spermine (days) |
Villus height (μm) |
Villus width (μm) |
Crypt depth (μm) |
Villus height/crypt depth |
Villus surface area (mm2) |
Data represent the mean ± SEM. Different superscripts (b–d) indicate significant difference (P < 0.05) between groups (group 1, group 2, group 3, group 4), between spermine (0, 0.2 μmol per g BW, main effects), and between time extension of spermine (3, 7 day, main effects) for one of all parameters (villus height, villus width, crypt depth, villus width/crypt depth, villus surface area), respectively. |
1 |
0 |
3 |
172.02 ± 10.90 |
49.27 ± 3.47b |
50.62 ± 3.46b |
3.44 ± 0.19b |
0.02 ± 0.00b |
2 |
0 |
7 |
191.87 ± 5.56 |
58.85 ± 1.39c |
67.27 ± 3.02cd |
2.67 ± 0.08c |
0.03 ± 0.00c |
3 |
0.2 |
3 |
186.77 ± 16.58 |
60.31 ± 3.33c |
61.76 ± 2.89c |
3.02 ± 0.22 bc |
0.04 ± 0.00c |
4 |
0.2 |
7 |
202.71 ± 9.18 |
67.30 ± 2.49c |
74.65 ± 4.34d |
2.75 ± 0.14c |
0.04 ± 0.00c |
![[thin space (1/6-em)]](https://www.rsc.org/images/entities/char_2009.gif) |
Main effects |
Spermine |
0 |
|
181.94 ± 8.34 |
54.06 ± 1.98b |
58.94 ± 2.46b |
3.05 ± 0.12 |
0.03 ± 0.00b |
0.2 |
|
194.74 ± 8.06 |
63.81 ± 1.98c |
68.21 ± 2.46c |
2.89 ± 0.12 |
0.04 ± 0.00c |
Time extension of spermine |
|
3 |
179.40 ± 8.06 |
54.79 ± 1.98b |
56.19 ± 2.46b |
3.23 ± 0.12b |
0.03 ± 0.00b |
|
7 |
197.29 ± 8.34 |
63.08 ± 1.98c |
70.96 ± 2.46c |
2.71 ± 0.12c |
0.04 ± 0.00c |
![[thin space (1/6-em)]](https://www.rsc.org/images/entities/char_2009.gif) |
P value |
Spermine |
|
|
0.28 |
0.00 |
0.01 |
0.34 |
0.00 |
Time extension of spermine |
|
|
0.14 |
0.01 |
0.00 |
0.01 |
0.01 |
Spermine × time extension of spermine |
|
|
0.87 |
0.65 |
0.59 |
0.15 |
0.63 |
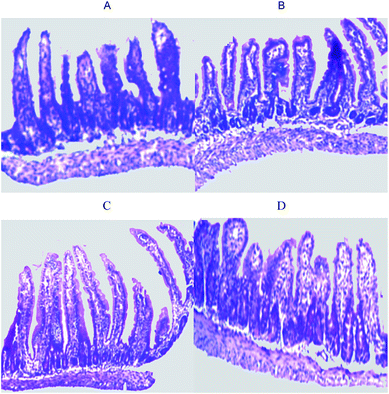 |
| Fig. 1 Ileum histomorphology of suckling rats. A, control-3 days (group 1); B, control-7 days (group 2); C, spermine-3 days (group 3); D, spermine-7 days (group 4). | |
Table 2 Effects of spermine and its duration extension on ileum enzymatic parameters of suckling ratsa
Groups |
Spermine (μmol per g BW) |
Time extension of spermine (days) |
Protein content (mg per g tissue) |
Lactase activity (U per mg protein) |
Sucrase activity (U per mg protein) |
Maltase activity (U per mg protein) |
Alkaline phosphatase activity (U per g protein) |
Data represent the mean ± SEM. Different superscripts (b and c) indicate significant difference (P < 0.05) between groups (group 1, group 2, group 3, group 4), between spermine (0, 0.2 μmol per g BW, main effects), and between time extension of spermine (3, 7 day, main effects) for one of all parameters (protein content, lactase activity, sucrase activity, maltase activity, alkaline phosphatase activity), respectively. |
1 |
0 |
3 |
72.24 ± 3.38 |
107.49 ± 9.62b |
4.30 ± 2.09b |
101.45 ± 17.08 bc |
2865.53 ± 234.27b |
2 |
0 |
7 |
76.14 ± 1.84 |
121.92 ± 8.96b |
2.10 ± 0.43b |
77.47 ± 5.67b |
2748.84 ± 383.59b |
3 |
0.2 |
3 |
71.45 ± 3.96 |
59.53 ± 7.43c |
32.49 ± 5.14c |
141.98 ± 13.27c |
2219.41 ± 279.40 bc |
4 |
0.2 |
7 |
73.61 ± 2.27 |
55.40 ± 4.48c |
24.43 ± 6.63c |
117.34 ± 16.41 bc |
1399.30 ± 212.06c |
![[thin space (1/6-em)]](https://www.rsc.org/images/entities/char_2009.gif) |
Main effects |
Spermine |
0 |
|
74.19 ± 2.11 |
114.70 ± 5.61b |
3.20 ± 3.11b |
89.46 ± 9.81b |
2807.19 ± 207.94b |
0.2 |
|
72.53 ± 2.11 |
57.46 ± 5.81c |
28.46 ± 3.11c |
129.66 ± 9.81c |
1809.36 ± 200.89c |
Time extension of spermine |
|
3 |
71.85 ± 2.11 |
83.51 ± 5.61 |
18.40 ± 3.22 |
121.72 ± 9.81 |
2542.47 ± 207.94 |
|
7 |
74.88 ± 2.11 |
88.66 ± 5.81 |
13.27 ± 3.01 |
97.41 ± 9.81 |
2074.07 ± 200.89 |
![[thin space (1/6-em)]](https://www.rsc.org/images/entities/char_2009.gif) |
P value |
Spermine |
|
|
0.58 |
0.00 |
0.00 |
0.01 |
0.00 |
Time extension of spermine |
|
|
0.32 |
0.53 |
0.26 |
0.09 |
0.12 |
Spermine × time extension of spermine |
|
|
0.77 |
0.26 |
0.51 |
0.98 |
0.23 |
1H NMR spectra of ileum sample
Fig. 2 shows the typical 1H NMR spectra of rat ileum from the control group and the spermine-treated group. A total of 43 metabolites were assigned for the ileum and listed in Table 3. The spectra of the ileum samples included resonances from glucose, lactate, lipids, choline metabolites, pyrimidines, and amino acids.
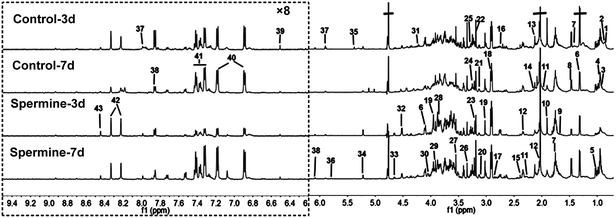 |
| Fig. 2 Representative one-dimensional 1H NMR spectra of ileum samples from control (control-3 days and control-7 days) and spermine-treated (0.2 μmol per g BW; spermine-3 days and spermine-7 days) groups. The region of δ6.2 to δ9.5 was magnified eight times compared with the corresponding region of δ0.7 to δ6.2 for clarity. A total of 43 metabolites were unambiguously assigned. The chemical shifts and peak multiplicities of these metabolites are listed in Table 3. | |
Table 3 1H NMR data of metabolites in rat ileum
Keys |
Metabolites |
Moieties |
δ1H (ppm) and multiplicity |
LDL, low density lipoprotein; VLDL, low density lipoprotein; TMAO, trimethylamine-N-oxide; s, singlet; d, doublet; t, triplet; q, quartet; dd, doublet of doublets; m, multiplet. |
1 |
LDLa |
CH3(CH2)n |
0.86(t) |
2 |
VLDLa |
CH3CH2CH2C![[double bond, length as m-dash]](https://www.rsc.org/images/entities/char_e001.gif) |
0.88(t) |
3 |
Isoleucine |
αCH, βCH, βCH3, γCH2, γCH3, δCH3 |
3.68(d), 1.99(m), 1.26(m), 1.47(m), 0.93(t), 1.02(d) |
4 |
Leucine |
αCH, βCH2, γCH, δCH3 |
3.73(t), 1.96(m), 1.72(m), 0.96(d), 0.97(d) |
5 |
Valine |
αCH, βCH, γCH3 |
3.62(d), 2.28(m), 1.05(d), 1.0(d) |
6 |
Lactate |
αCH, βCH3 |
4.13(q), 1.33(d) |
7 |
Lysine |
αCH, βCH2, γCH2, εCH2 |
3.76(t), 1.92(m), 1.74(m), 1.49(m), 3.01(t) |
8 |
Alanine |
αCH, βCH3 |
3.77(q), 1.49(d) |
9 |
Arginine |
αCH, βCH2, γCH2, εCH2 |
3.76(t), 1.89(m), 1.63(m), 3.23(t) |
10 |
Acetate |
CH3 |
1.92(s) |
11 |
Lipids (triglycerides and fatty acids) |
(CH2)n, CH2CH2CO, CH2C C, C CCH2C C |
1.28(m), 2.23(m), 2.01(m), 1.8(m), 2.76(m) |
12 |
Glutamate |
αCH, βCH2, γCH2 |
3.75(m), 2.12(m), 2.35(m) |
13 |
Methionine |
αCH, βCH2, γCH2, S–CH3 |
3.87(t), 2.16(m), 2.6(dd), 2.14(s) |
14 |
Glutamine |
αCH, βCH2, γCH2 |
3.78(m), 2.14(m), 2.46(m) |
15 |
Oxaloacetate |
CH2 |
2.38(s) |
16 |
Aspartic acid |
αCH, βCH2 |
3.89(m), 2.79(m) |
17 |
Asparagine |
αCH, βCH2 |
3.99(m), 2.86(m) |
18 |
Glutathione |
CH2, S–CH2, N–CH, CH, CH3 |
2.17(m), 2.55(m), 2.95(m), 3.83(m), 4.56(m), 0.89(m) |
19 |
Creatine |
CH3, CH2 |
3.03(s), 3.93(s) |
20 |
Cysteine |
βCH2 |
3.13(m) |
21 |
Ethanolamine |
CH2 |
3.15(t) |
22 |
Choline |
OCH2, NCH2, N(CH3)3 |
4.05(t), 3.51(t), 3.20(s) |
23 |
Glycerolphosphocholine |
CH3, βCH2, αCH2 |
3.22(s), 3.69(t), 4.32(t) |
24 |
Taurine |
–CH2–S, –CH2–NH2 |
3.26(t), 3.4(t) |
25 |
TMAOa |
CH3 |
3.27(s) |
26 |
scyllo-Inositol |
CH |
3.35(s) |
27 |
Glycine |
CH2 |
3.55(s) |
28 |
Serine |
αCH, βCH2 |
3.84(m), 3.96(m) |
29 |
Betaine |
CH3, CH2 |
3.27(s), 3.9(s) |
30 |
myo-Inositol |
1,3-CH, 2-CH, 5-CH, 4,6-CH |
3.60(dd), 4.06(t), 3.30(t), 3.63(t) |
31 |
Threonine |
αCH, βCH, γCH3 |
3.58(d), 4.25(m), 1.32(d) |
32 |
D-Galactose |
1-CH |
4.58(d) |
33 |
β-Glucose |
1-CH, 2-CH, 3-CH, 4-CH, 5-CH, 6-CH |
4.65(d), 3.25(dd), 3.49(t), 3.41(dd), 3.46(m), 3.73(dd), 3.90(dd) |
34 |
α-Glucose |
1-CH, 2-CH, 3-CH, 4-CH, 5-CH, 6-CH |
5.24(d), 3.54(dd), 3.71(dd), 3.42(dd), 3.84(m), 3.78(m) |
35 |
Unsaturated lipids |
CH–CH2C , –CH CH– |
5.19(m), 5.31(m) |
36 |
Uracil |
CH |
5.78(d), 7.52(d) |
37 |
Cytosine |
CH |
5.86(d), 7.84(d) |
38 |
Isocytosine |
CH |
6.03(d), 7.82(d) |
39 |
Fumarate |
CH |
6.52(s) |
40 |
Tyrosine |
2,6-CH, 3,5-CH |
7.16(dd), 6.87(d) |
41 |
Phenylalanine |
2,6-CH, 3,5-CH, 4-CH |
7.33(m), 7.40(m), 7.35(m) |
42 |
Inosine |
2-CH, 8-CH, 4-CH, 5-CH,CH2 |
8.34(s), 6.1(d), 8.24(s), 4.44(t), 4.28(q), 3.92(dd) |
43 |
Formate |
CH |
8.45(s) |
Multivariate data analysis of NMR data
PCA was initially carried out on ileum spectral data. For the treatment groups, two principal components were calculated, in which 39.7% and 29.4% of the variables were explained by PC1 and PC2, respectively. The PCA results (Fig. 3A) demonstrated that separation was not observed in the metabolic ileum profiles of rats from spermine-3 days, spermine-7 days, control-3 days, and control-7 days groups. The ileum spectra of spermine-treated and control groups were also subjected to PLS-DA (Fig. 3B). The score plots clearly highlighted four clusters corresponding to the four groups. The metabolic changes in the ileum of the rats from spermine-treated and control groups were also analyzed by OPLS-DA. The corresponding coefficient analysis showed that spermine significantly increased the ileum levels of choline, glutamate, glutamine, lactate, methionine, myo-inositol, scyllo-inositol, taurine, threonine, and trimethylamine-N-oxide, but decreased the level of lysine. The ileum levels of α-glucose, aspartate, creatine, D-galactose, formate, glutathione, glycerolphosphocholine, lipid, and tyrosine were significantly affected by spermine ingestion (P < 0.05, Table 4, Fig. 4A and B). Furthermore, extended spermine administration significantly increased α-glucose, aspartate, choline, creatine, D-galactose, glutamate, glycine, lactate, lysine, fumaric acid, myo-inositol, serine, trimethylamine-N-oxide, and decreased glutathione and methionine levels in the ileum of the spermine group (P < 0.05, Table 5 and Fig. 4C).
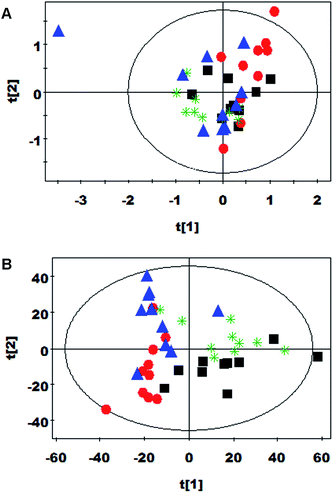 |
| Fig. 3 PCA score plots (R2X = 0.941, Q2 = 0.773; (A)) and PLS-DA score plots (R2X = 0.264, R2Y = 0.35, Q2 = 0.0799; (B)) on the basis of the 1H NMR spectra of 24 h ileum samples from spermine-treated [spermine-3 days (red circles) and spermine-7 days (blue triangles)] and control [control-3 days (black squares) and control-7 days (green stars)] groups. | |
Table 4 OPLS-DA coefficients obtained from the NMR data of ileum metabolites from the (A) control group and (B) spermine-treated groups
Metabolite |
B (vs. A) OPLS-DA coefficient (r)a |
3 days |
7 days |
Metabolite keys were shown in Table 3; correlation coefficients were calculated from OPLS-DA results with positive and negative signs indicating positive and negative correlation in the concentrations, respectively. The correlation coefficient of |r| > 0.666 (3 days) or 0.632 (7 days) represented the cutoff value. “−” means the correlation coefficient |r| is less than 0.666 (3 days) or 0.632 (7 days). |
α-Glucose (34) |
−0.669 |
0.7 |
Aspartic acid (16) |
−0.756 |
0.678 |
Betaine (29) |
— |
0.687 |
Choline (22) |
0.908 |
0.794 |
Creatine (19) |
−0.683 |
— |
D-Galactose (32) |
−0.682 |
— |
Formate (43) |
0.778 |
— |
Cytosine (37) |
— |
0.673 |
Glutamate (12) |
0.863 |
0.771 |
Glutamine (14) |
0.786 |
0.658 |
Glutathione (18) |
0.691 |
— |
Glycerolphosphocholine (23) |
— |
0.703 |
Isocytosine (38) |
0.721 |
0.714 |
Inosine (42) |
0.724 |
0.802 |
Lactate (6) |
0.85 |
0.809 |
Lipid (11) |
0.792 |
— |
Lysine (7) |
−0.771 |
−0.643 |
Methionine (13) |
0.761 |
0.655 |
myo-Inositol (30) |
0.768 |
0.729 |
scyllo-Inositol (26) |
0.832 |
0.795 |
Taurine (24) |
0.9 |
0.822 |
Threonine (31) |
0.687 |
0.769 |
TMAO (25) |
0.834 |
0.763 |
Tyrosine (40) |
−0.694 |
— |
Uracil (36) |
0.831 |
— |
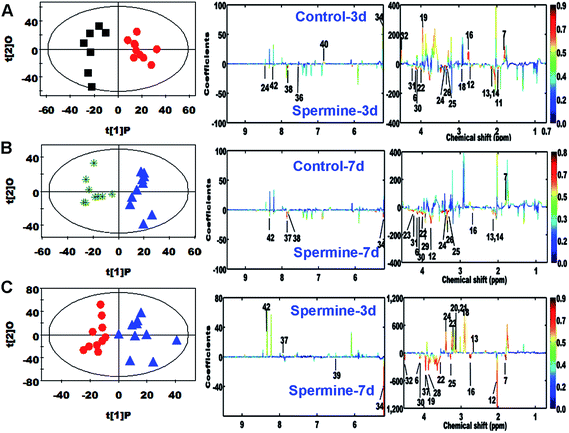 |
| Fig. 4 OPLS-DA scores plots (left panel) and corresponding coefficient loading plots (right panel) obtained from 1H NMR spectra of 24 h ileum samples from spermine-treated [spermine-3 days (red circles) and spermine-7 days (blue triangles)] and control [control-3 days (black squares) and control-7 days (green stars)] groups ((A), R2X = 0.303, Q2 = 0.529; (B), R2X = 0.214, Q2 = 0.479; (C), R2X = 0.357, Q2 = 0.114). Two samples from the control-3 days group and one sample from the control-7 days group were excluded because they lay outside the Hotelling' T2 ellipse on the score plot. Color scale in the coefficient plot shows the significance of metabolite variation between spermine-treated (spermine-3 days and spermine-7 days) and control (control-3 days and control-7 days) groups. | |
Table 5 OPLS-DA coefficients obtained from the NMR data of ileum metabolites from the (A) 3 day group and (B) 7 day-treated groups
Metabolite |
B (vs. A) OPLS-DA coefficient (r)a |
Control |
Spermine |
Metabolite keys were shown in Table 3; correlation coefficients were calculated from OPLS-DA results with positive and negative signs indicating positive and negative correlation in the concentrations, respectively. The correlation coefficient of |r| > 0.602 represented the cutoff value. “−” means the correlation coefficient |r| is less than 0.602. |
Acetate (10) |
0.651 |
— |
α-Glucose (34) |
0.875 |
0.764 |
Aspartic acid (16) |
0.617 |
0.865 |
Choline (22) |
0.697 |
0.734 |
Creatine (19) |
0.788 |
0.763 |
Cysteine (20) |
— |
−0.733 |
Cytosine (37) |
— |
−0.628 |
Inosine (42) |
— |
−0.653 |
D-Galactose (32) |
0.677 |
0.787 |
Ethanolamine (21) |
— |
−0.66 |
Fumarate (39) |
— |
0.672 |
Glutamate (12) |
0.778 |
0.731 |
Glutathione (18) |
— |
−0.639 |
Glycine (22) |
0.62 |
0.787 |
Glycerolphosphocholine (23) |
−0.672 |
−0.709 |
Lactate (6) |
0.769 |
0.746 |
Lysine (7) |
0.862 |
0.881 |
Methionine (13) |
— |
−0.787 |
myo-Inositol (30) |
— |
0.639 |
Serine (28) |
0.829 |
0.723 |
Taurine (24) |
— |
−0.738 |
TMAO (25) |
0.884 |
0.798 |
Uracil (36) |
−0.687 |
— |
Discussion
Effects of spermine and its duration extension on ileum development maturation
Spermine is essential for the cell growth and proliferation, and function of normal cells. It is involved in DNA, RNA and protein synthesis. There is evidence to suggest that it is intimately engaged in the control of cell survival. Therefore, every cell in animal body requires spermine for its proper function. Spermine and its duration extension significantly increased the villus width, surface area, and crypt depth of rat ileum. Spermine also significantly increased the specific activities of sucrase and maltase but decreased the activity of lactase and alkaline phosphatase in rat ileum. These results suggested that spermine ingestion can improve intestinal development maturation. This finding also agrees with that of a previous study that spermine administration can enhance intestinal maturation.2,4–7 The different alterations of enzymatic activity can exert discriminatory influence on ileum metabolism because enzyme can modulate nutrition metabolic flux of animals. Then we discuss influence of spermine and its duration extension on ileum nutrition metabolism.
Effects of spermine on ileum nutrition metabolism
Spermine can induce alterations in lipid metabolism (Fig. 5). Spermine can be transported into the mitochondrial membrane by an electrophoretic mechanism.21 Interestingly, spermine supplementation in the present study increased the concentrations of glycerolphosphocholine (GPC) and choline. GPC and choline are essential constituents for the structural integrity of the cell membrane. These elements have important functions in cell metabolism and signaling processes.22,23 This finding agrees with the result of our previous study in which spermine can alter cell membrane metabolism in weaned rats.15,24 GPC can be synthesized with choline in mammalian and bacterial cells.25 Accordingly, the increase in ileum choline concentration was in relation with the increase in circulating levels of GPC in spermine-supplemented rats. GPC functions as an inhibitor of lysophospholipase, which catalyzes the hydrolysis of physiologically important lysophospholipid substrates (including lysophosphatidic acid and sphingosine 1-phosphate). These lipids modulate cellular signaling and nutrient metabolism.26 The ileum concentration of myo-inositol was increased in response to spermine supplementation. This carbocyclic polyol offers the structural basis for many secondary messengers (containing inositol phosphates, phosphatidylinositol, and phosphatidylinositol phosphate) in eukaryotic cells.27 Inositol is also associated with regulating intracellular calcium (Ca) concentrations, insulin signal transduction, gene expression, and fatty acid oxidation.27,28 This result is in accordance with that of a previous study, in which spermine increases electrogenic Ca2+ uptake and enhances mitochondrial Ca2+ retention.29 GPC also play important roles in lipid cholesterol transport and metabolism.23 Furthermore, spermine supplementation can enhance ileum lipid concentration of suckling rats. In summary, spermine ingestion can change lipid metabolism.
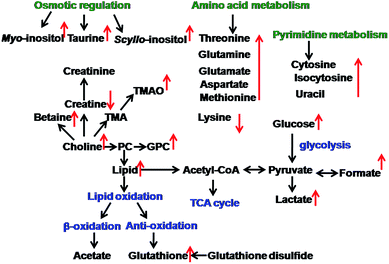 |
| Fig. 5 Spermine-induced changes in the metabolic pathway. , up-regulated; , down-regulated. PC, phosphorylcholine; GPC, glycerolphosphocholine; TCA cycle, tricarboxylic acid cycle. TMA, trimethylamine; TMAO, trimethylamine-N-oxide. | |
Spermine can change intestinal osmotic pressure (Fig. 5). Intestinal epithelial cells are subjected to hypertonic environments when digested foods move into the gastrointestinal tract. Therefore, osmotic regulation is necessary to maintain osmotic equilibrium in the gastrointestinal tract between a cell and its external environment. Such regulation is performed by maintaining a high intracellular content of osmolytes. An increase in myo-inositol and scyllo-inositol levels could be linked to specific osmoreregulatory functions to balance luminal and intracellular milieus.30–32 Taurine is a well-characterized muscular tissue component with essential regulatory functions in osmosis, as well as muscular contractility and excitability.33 Therefore, taurine is directly related to the energy state of intestinal cells.34 The ileum also actively reabsorbs 90% to 95% of all the luminal tauro-conjugated bile acids,35 which account for high taurine level in ileum tissues in the current study. Collectively, spermine can alter the osmotic pressure in the ileum.
Spermine can also change energy metabolism (Fig. 5). In the current study, spermine increased the lactate level in the ileum. Lactate is the end product of compounds in response to energy metabolism. Increased lactate level is associated with increased anaerobic glycolysis. An increased ileum lactate level also implies that carbohydrate and energy metabolism are modified. Furthermore, spermine can affect the glucose level in the ileum. In our previous study, spermine altered the plasma glucose level of weaned rats.15 Thus, spermine can alter energy metabolism.
Spermine exposure can affect amino acid metabolism (Fig. 5). Amino acids are necessary to generate protein and energy in intestinal mucosal cells. Relative increases in the levels of amino acids were found in the ileum tissues of the spermine group compared with the control group. Increased threonine level in the spermine group suggested that threonine, which is an essential constituent of intestinal mucin synthesis36 and key element in protecting the mucosa from autodigestion by gastric juice,37 can contribute to the synthesis of intestinal mucins; as a result, intestinal function is improved because mucins are necessary to lubricate tissues and modulate water and electrolyte absorption, as well as protect the epithelium from mechanical and chemical stress.38 Therefore, spermine is vital in maintaining gut barrier integrity. In support of this view, ileum glutamine can be significantly increased by spermine supplementation. Glutamine can activate signaling pathways to increase protein synthesis. Glutamine is an important fuel for the mucosal cells of the small intestine and plays a crucial role in maintaining the function and integrity of the intestine.39 Hence, glutamine is particularly essential for the growth, development, and function of the intestine. Ileum glutamate and aspartate were also significantly increased. Glutamate is the preferential source of mucosal glutathione synthesis in animals. Aspartate is also catabolized as fuel used by intestinal enterocytes for rapid division, epithelial cell repair, nutrient transport, and protein turnover.40 Taken together, spermine ingestion can enhance intestinal amino acid metabolism.
Spermine exposure can promote antioxidative ability (Fig. 5). In the current study, glutathione, which is related to oxidative defense, was clearly increased in the ileum after spermine supplementation was administered. Alterations in glutathione levels can be considered as biomarkers of antioxidant capacity.41,42 As one of the most important defense barriers against diet-derived mutagens, carcinogens, and oxidants, the optimal development of an antioxidative capacity during growth is crucial for health.43,44 Increased glutathione levels are consistent with enhanced oxidative defense in the spermine group of a previous in vitro study.45
Spermine exposure can alter gut microbiota metabolism (Fig. 5). Spermine significantly increased trimethylamine-N-oxide level in the rat ileum. Trimethylamine (TMA) may correspond to microbial activity modification as dietary methyl components, such as carnitine and choline, are degraded to TMA by gut bacterial species.46 TMA is bound to lipoproteins after transluminal transport occurs and the liver is reached via blood circulation in which TMA is oxidized into trimethylamine-N-oxide.47
Time extension effect of spermine administration on ileum nutrition metabolism
Extended spermine administration can alter energy metabolism. The time extension-treated group exhibited a significant increase in plasma glucose levels compared with the control group. Glucose is a major energy substrate that plays a role in animal growth and development. Increased lactate concentration was also found in the ileum of the time extension-treated group. An increased ileum lactate level also implies that carbohydrate and energy metabolisms are altered. In addition, creatine was increased by extended spermine administration. Creatine provides energy to muscles in vertebrates in the form of stored creatine phosphate. The creatine levels in animals are synthesized de novo in the liver by amino acids, such as arginine, glycine, and methionine. Thus, extended spermine administration can affect energy metabolism.
Extended spermine administration can also change amino acid metabolism. Cells require amino acids to produce protein and generate energy in the ileum mucosa. In the current study, aspartate, glutamate, glycine, lysine, and serine levels were significantly increased. Enteral glutamate is the preferential source for mucosal glutathione synthesis in animals.48 Glycine is interconverted to serine to provide a mechanism for activated one-carbon group transfer.48 Serine and aspartate are also catabolized as fuels by intestinal enterocytes for rapid division, epithelial cell repair, nutrient transport, and protein turnover.48 Considering this observation, we found that extended spermine administration generally showed an altered gut morphology with increased villus height and surface area to compensate for a less efficient nutrient usage. As a result, energy requirements for cell division and growth are augmented in the intestine. Thus, extended spermine administration can alter amino acid metabolism.
Conclusion
Our results suggested that spermine ingestion induces morphological and common systemic metabolic processes modifications characterizing the ileum postnatal maturation of suckling rats. Metabolic variations could be attributed to the functional variations in lipid metabolism, energy metabolism, amino acid metabolism, gut microbial activity, osmoregulation, and oxidative protection, which have important implications in nutritional research in infants. These findings provided new essential information on the effects of spermine ingestion and showed the large potential of a nutrimetabolomic strategy in a mammalian system. To our knowledge, this in vivo study is the first to report on the response of animal intestinal biological systems from spermine supplementation. However, further research should be conducted to clarify the mechanisms of the effects of spermine on other types of tissue intermediary metabolism.
Acknowledgements
We also thank all the study participants at our laboratory for their ongoing assistance. This work was funded by the National Natural Science Foundation of China (no: 31301986), Research Foundation of Education Bureau of Sichuan Province (no: 11ZB061) and Specific Research Supporting Program for Discipline Construction in Sichuan Agricultural University.
References
- A. E. Pegg, IUBMB Life, 2014, 66, 8–18 CrossRef CAS PubMed.
- C. Dufour, G. Dandrifosse, P. Forget, F. Vermesse, N. Romain and P. Lepoint, Gastroenterology, 1988, 95, 112–116 CAS.
- J. C. ter Steege, W. A. Buurman and P. P. Forget, J. Pediatr. Gastroenterol. Nutr., 1977, 25, 332–340 CrossRef PubMed.
- Z. B. Cheng, D. F. Li, J. J. Xing, X. Y. Guo and Z. J. Li, Anim. Sci., 2006, 82, 621–626 CrossRef CAS.
- P. Kang, M. Wang, Y. Hou, Y. Yin, B. Ding, H. Zhu, Y. Liu, Y. Qiu, D. Yi, L. Wang and J. Gong, J. Anim. Vet. Adv., 2012, 11, 2782–2787 CrossRef CAS.
- O. Peulen, C. Pirlet, M. Klimek, G. Goffinet and G. Dandrifosse, Arch. Physiol. Biochem., 1998, 106, 46–55 CrossRef CAS PubMed.
- P. Deloyer, O. Peulen and G. Dandrifosse, Exp. Physiol., 2005, 90, 901–908 CrossRef CAS PubMed.
- M. Kaouass, P. Deloyer, I. Wery and G. Dandrifosse, Dig. Dis. Sci., 1996, 41, 1434–1444 CrossRef CAS.
- I. Wéry, P. Deloyer and G. Dandrifosse, Arch. Physiol. Biochem., 1996, 104, 163–172 CrossRef PubMed.
- O. Peulen, G. Denis, M. P. Defresne and G. Dandrifosse, Dig. Dis. Sci., 2001, 46, 2490–2498 CrossRef CAS.
- J. P. Buts, N. De Keyser, J. Kolanovsky and F. Vann Hoof, Pediatr. Res., 1990, 27, 161–164 CrossRef CAS PubMed.
- A. Mahmood and R. Torres-Pinedo, Pediatr. Res., 1985, 19, 899–902 CrossRef CAS PubMed.
- O. Peulen and G. Dandrifosse, J. Pediatr. Gastroenterol. Nutr., 2004, 38, 524–532 CrossRef CAS PubMed.
- E. Harada, Y. Hashimoto and B. Syuto, Comp. Biochem. Physiol., Part A: Mol. Integr. Physiol., 1994, 109, 667–673 CrossRef CAS.
- G. M. Liu, T. T. Fang, T. Yan, G. Jia, H. Zhao, Z. Q. Huang, X. L. Chen, J. Wang and B. Xue, J. Agric. Food Chem., 2014, 62, 9035–9042 CrossRef CAS PubMed.
- M. M. Bradford, Anal. Biochem., 1976, 72, 248–254 CrossRef CAS.
- A. Dahlqvist, Anal. Biochem., 1968, 22, 99–107 CrossRef CAS.
- J. Trygg and S. Wold, J. Chemom., 2002, 16, 119–128 CrossRef CAS PubMed.
- O. Cloarec, M. E. Dumas, J. Trygg, A. Craig, R. H. Barton, J. C. Lindon, J. K. Nicholson and E. Holmes, Anal. Chem., 2005, 77, 517–526 CrossRef CAS PubMed.
- F. Lindgren, B. Hansen, W. Karcher, M. SjÖstrÖm and L. Eriksson, J. Chemom., 1996, 10, 521–532 CrossRef CAS.
- S. Grancara, P. Martinis, S. Manente, A. N. García-Argáez, G. Tempera, M. Bragadin, L. D. Via, E. Agostinelli and A. Toninello, Amino Acids, 2014, 46, 671–679 CrossRef CAS PubMed.
- J. Klein, J. Neural Transm., 2000, 107, 1027–1063 CrossRef CAS.
- S. H. Zeisel and J. K. Blusztajn, Annu. Rev. Nutr., 1994, 14, 269–296 CrossRef CAS PubMed.
- G. M. Liu, T. T. Fang, T. Yan, G. Jia, H. Zhao, X. Chen, C. M. Wu and J. Wang, RSC Adv., 2014, 4, 56766–56778 RSC.
- C. T. Burt and H. Ribolow, Comp. Biochem. Physiol., Part B: Biochem. Mol. Biol., 1994, 108, 11–20 CrossRef CAS.
- A. Tokumura, J. Cell. Biochem., 2004, 92, 869–881 CrossRef CAS PubMed.
- G. Di Paolo and P. De Camilli, Nature, 2006, 443, 651–657 CrossRef CAS PubMed.
- R. A. Siddiqui, K. A. Harvey and G. P. Zaloga, J. Nutr. Biochem., 2008, 19, 417–437 CrossRef CAS PubMed.
- H. Rottenberg and M. Marbach, Biochim. Biophys. Acta, 1990, 1016, 77–86 CrossRef CAS.
- G. Guan, P. Dai and I. Shechter, Arch. Biochem. Biophys., 2003, 417, 251–259 CrossRef CAS.
- B. Holub, Annu. Rev. Nutr., 1986, 6, 563–597 CrossRef CAS PubMed.
- P. H. Yancey, M. E. Clark, S. C. Hand, R. D. Bowlus and G. N. Somero, Science, 1982, 217, 1214–1222 CAS.
- R. J. Huxtable, Physiol. Rev., 1992, 72, 101–163 CAS.
- U. Flogel, T. Niendorf, N. Serkowa, A. Brand, J. Henke and D. Leibfritz, Neurochem. Res., 1995, 20, 793–802 CrossRef CAS.
- M. H. Floch, Dig. Liver Dis., 2002, 34, S54–S57 CrossRef.
- M. Faure, D. Moennoz, F. Montigon, C. Mettraux, D. Breuille and O. Ballevre, J. Nutr., 2005, 135, 486–491 CAS.
- A. Allen, G. Flemstrom, A. Garner and E. Kivilaakso, Physiol. Rev., 1993, 73, 823–857 CAS.
- C. S. Potten, Philos. Trans. R. Soc., B, 1998, 353, 821–830 CrossRef CAS PubMed.
- G. Wu, S. A. Meier and D. A. Knabe, J. Nutr., 1996, 126, 2578–2584 CAS.
- H. G. Windmueller and A. E. Spaeth, J. Biol. Chem., 1980, 255, 107–112 CAS.
- J. Wang, L. Chen, P. Li, X. Li, H. Zhou, F. Wang, D. Li, Y. Yin and G. Wu, J. Nutr., 2008, 138, 1025–1032 CrossRef CAS PubMed.
- X. Wang, D. Ou, J. Yin, G. Wu and J. Wang, Amino Acids, 2009, 37, 209–218 CrossRef CAS PubMed.
- Z. Tang, Y. Yin, Y. Zhang, R. Huang, Z. Sun, T. Li, W. Chu, X. Kong, L. Li, M. Geng and Q. Tu, Br. J. Nutr., 2009, 101, 998–1005 CrossRef CAS PubMed.
- G. Wu, F. W. Bazer, T. A. Davis, S. W. Kim, P. Li, J. M. Rhoads, M. C. Satterfield, S. B. Smith, T. E. Spencer and Y. Yin, Amino Acids, 2009, 37, 153–168 CrossRef CAS PubMed.
- J. E. Rider, A. Hacker, C. A. Mackintosh, A. E. Pegg, P. M. Woster and R. A. Casero Jr, Amino Acids, 2007, 33, 231–240 CrossRef CAS PubMed.
- B. A. Seibel and P. J. Walsh, J. Exp. Biol., 2002, 205, 297–306 CAS.
- J. L. Smith, J. S. Wishnok and W. M. Deen, Toxicol. Appl. Pharmacol., 1994, 125, 296–308 CrossRef CAS PubMed.
- G. Wu, J. Nutr., 1998, 128, 1249–1252 CAS.
|
This journal is © The Royal Society of Chemistry 2015 |