DOI:
10.1039/C5RA00358J
(Review Article)
RSC Adv., 2015,
5, 26686-26705
Green chemistry approaches as sustainable alternatives to conventional strategies in the pharmaceutical industry
Received
8th January 2015
, Accepted 16th February 2015
First published on 19th February 2015
Abstract
Green chemistry is a rapidly developing field providing an avenue for the sustainable development of future science and technology. It offers enhanced chemical process economics, concomitant with a reduced environmental burden. It can be applied to design environmentally benign synthetic protocols to deliver life-saving medicines, while minimizing environmental impact. It is expected that chemists and chemical engineers should produce greener and more sustainable chemical processes for drug design and it is likely that this trend will continue to grow over the next few decades. This review summarizes environmentally benign protocols for the synthesis of some FDA (Food and Drug Administration) approved drugs which are in high volume demand coupled with their requirements of high chemical and optical purity utilizing the principles of green chemistry.
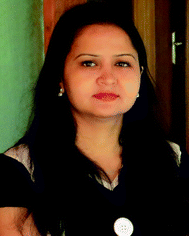 Princy Gupta | Princy Gupta, Ph.D obtained her Ph.D from the University of Jammu, Jammu, India with Prof. Satya Paul where she worked in the ambit of Green Chemistry using solid acids particularly carbon based solid acids and their applications in organic synthesis. She worked as a Lecturer on a contractual basis in the Department of Chemistry, University of Jammu for two academic years, where she taught Medicinal Chemistry and Organic Chemistry to post-graduate students. At present she is working as a Part-Time Lecturer in the Department of Chemistry, Guru Nanak Dev University, Amritsar. Her research interests include the development of novel solid acid catalysts and their use for the synthesis of drugs using Green Chemistry principles. |
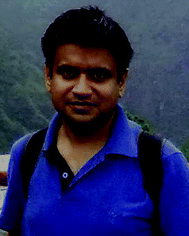 Aman Mahajan | Aman Mahajan Ph.D, obtained his Ph.D from Guru Nanak Dev University under the supervision of Prof. Kamaljit Singh where he explored the synthesis and structural characterization of hetarylazo disperse dyes based on diazo components and regioselective scaffold decoration of 3,4-dihydropyrimidin-2-(1H)-ones followed by postdoctoral experience at the University of CapeTown, South-Africa with Professor Kelly Chibale where he extensively worked on synthesis of drug-like molecules in collaboration with (a) AuTEK-Bimed Advanced Material Division, South Africa, (b) NRF, South Africa and (c) European union antimal project. In November 2011, he joined as a research scientist in the API department with Prof. M.P Mahajan (Director) at the Apeejay Stya Research foundation, Gurgaon, India and is involved extensively in developing economically viable/tangible cost-effective routes for known drugs (steroidal and non steroidal) which are either in great demand and/or too expensive. |
Introduction
The concepts of green chemistry and green engineering are not new in the pharmaceutical industry. In recent years, significant efforts have been invested to improve efficiency, reduce waste, and enhance quality and control in research and development (R & D). This is driven by the desire not only to reduce costs but also to increase the sustainability of the manufacturing processes. The pharmaceutical industry is the most profitable of the chemical industries and its growth increases by 5–6% every year.1a In the last decade, pharmaceutical industries1b–5 embraced green chemistry ideas to promote their environmental credentials and increase the efficiency of their manufacturing processes.6 It is known that the pharmaceutical industries produce more waste per kg of product than other chemical industries e.g. petrochemical, bulk and fine chemicals etc. A pharmaceutical industry produces approximately 25–100 kg of waste for every one kg of product for organic synthetic routes involving 6–8 steps.7a,b An additional problem with these industries are the new regulations for environmental pollution of water bodies. There is great need for pharmaceutical manufacturers to change methods into “greener” methods, to use less toxic reagents and solvents; and to minimize their effluents and solid waste.7c–e
1. Green chemistry metrics
In the past decade, there has been much discussion about the use of metrics to drive research communities towards more sustainable practices. In general, it is widely accepted that a good metric must be simple, easily measurable, clearly defined, objective and must ultimately drive the right behaviour. Several metrics have been proposed under this premise to encourage chemists and engineers to design greener, safer and more sustainable processes e.g. some of the mass-based metrics include process mass intensity (PMI), mass efficiency, reaction mass efficiency, E-factor, atom economy, space-time yield, amongst others. Three of the most common metrics that are used are the E-factor, process mass intensity (PMI) and atom economy.
2. Environmental factor (E-factor)
The simplest concepts are often the most effective, and this can certainly be said for the E-factor which was proposed by Sheldon in 1992.8a The E-factor is defined as the ratio of waste over the product.
It may however be difficult for the pharmaceutical industries to routinely use this metric in their operations. This is because there may be a lack of clarity depending on how ‘total waste’ is ultimately defined and accounted for and where the boundaries of the process are drawn. One may draw a boundary around the immediate process, around the facility or within the broader geographic region of the facility. Some examples of this lack of clarity in defining waste include: is waste that passes over the fence line the only waste considered? Is waste that is produced as a result of emissions treatment (e.g., acid gas scrubbing, pH adjustment in waste water treatment plants, etc.) included? Is waste that is produced as a result of energy use (heating or cooling reactions etc.) included? From an operational perspective, these kinds of questions complicate the routine use of this metric for individuals whose primary concern is to get new products on to the market in as short a period of time as possible.
3. Atom economy
The concept of atom economy was first proposed by Trost in 1991.8b At about the same time Sheldon proposed the very similar concept of atom utilisation8c but it is the atom economy name that has become widespread in use. Atom economy is simply defined as:
4. Process mass intensity (PMI)
Process mass intensity is defined as the total mass of materials used to produce a specified mass of product. Materials include reactants, reagents, solvents used for reaction and purification, and catalysts. Ideally this equals unity when no waste is produced and all materials are incorporated into the product.9a
It has been generally argued that using metrics such as E-factor would help drive the reduction of waste and this is certainly a good starting point, although not the best. After all, one can argue that the only difference between E-factor and PMI is one.
However, that difference of one is equivalent to the saleable product of a company; in other words, it is the factor that produces revenue for any business in the chemical and allied industries. For instance, the ideal state of PMI is when all the materials going into the process are integrated into the product, thus contributing actively to the generation of revenue (this corresponds to a PMI value of 1 and therefore an E-factor of zero). However, the waste from the manufacturing process is only a part of the equation. But in a broad sense it does not really matter which of these metrics one uses, historically waste and waste reduction have not come anywhere close to capturing management attention to the extent that the cost of high-value materials does.
Some work has also been performed since the late 1990s on the LCI/A Life Cycle Inventory and Assessment of pharmaceutical processes. LCI/A is a methodology that allows one to more precisely estimate the cumulative environmental impacts associated with manufacturing all the chemicals, materials and equipments used to make a product or deliver a service, thus providing a comprehensive view of the potential trade-offs in environmental impacts associated with a given process or product across the entire life cycle.9b–d,10 If one wants to measure the ‘greenness’ of a process, the ideal is to have at hand a variety of metrics that include LCI/A metrics to best represent the overall sustainability of a process or product. However, performing the LCI/A consumes significantly more time and effort than estimating PMI or even E-factor.
To truly integrate green chemistry and engineering into chemical processes, one has to look at the inputs instead of the output leading metrics that allow us to facilitate changes as the processes and routes are being designed and tested. In this context, mass and energy inputs (how much and what types) are the first line leading metrics for chemists and engineers designing chemical and pharmaceutical routes.
This review highlights synthetic protocols of some best selling FDA approved drugs that utilize green chemistry principles for their syntheses (Table 1).
Table 1 Drugs with their biological activities and advantages of green approaches over conventional approaches
S. no. |
Drug |
Activity |
Conventional approaches |
Green approach |
1 |
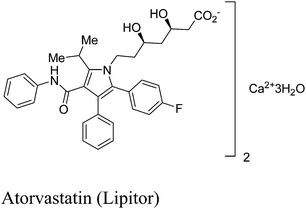 |
Cholesterol-lowering |
Use of cyanide; extensive by-products; low yield |
Biocatalytic route; E-factor = 5.8, if water is not included and 18 if water is included |
2 |
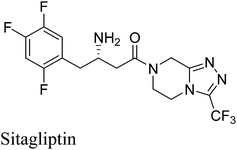 |
Antidiabetic |
Poor atom economy and large number of steps; use of expensive rhodium based catalyst; low stereocontrol in the asymmetric hydrogenation step |
Biocatalytic route; only three step synthesis; use of transaminase enzyme in place of rhodium; reduction in waste by 19% |
3 |
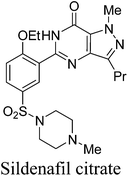 |
For the treatment of male erectile dysfunction |
Use of tin chloride (environment polluter); toxic hydrogen peroxide, oxalyl chloride and thionyl chloride as solvent |
Convergent and clean; catalytic hydrogenation in place of with tin chloride; stoichiometric amount of thionyl chloride; KOBut in tBuOH in place of hydrogen peroxide |
4 |
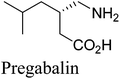 |
To treat epilepsy, neuropathic pain, anxiety and social phobia |
Overall yield was only 20%; high process mass intensity; high manufacturing costs |
Biocatalytic route; E-factor reduced from 86 to 17; over 10 million gallons of alcoholic organic solvents and nearly 2000 metric tons of raw materials (mandelic acid, CNDE, Ni) were eliminated annually |
5 |
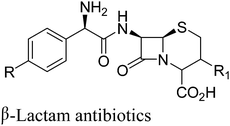 |
Antibiotic |
Use of toxic silylating agent, phosphorus pentachloride and dichloromethane |
Biocatalytic route, use of water; raw material efficiency and E-factor improved |
6 |
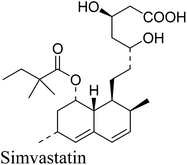 |
Cholesterol-lowering and for the treatment of dyslipidemia, cardiovascular diseases |
Laborious, expensive |
Biocatalytic route, single step; high yield >99% |
7 |
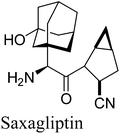 |
DPP-IV inhibitor |
Use of toxic potassium cyanide |
Biocatalytic route; reduction in number of steps from five to one; eliminating the use of cyanide and the expensive chiral reagent; use of water as solvent |
8 |
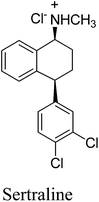 |
For depression as well as dependency and other anxiety-related disorders |
Four steps to establish the skeleton of the racemic tetralone; Friedel–Crafts acylation required excess AlCl3 and carbon disulphide; use of TiCl4 which produce hazardous by-products and solid wastes |
Selective catalytic reduction with Pd/CaCO3; double overall product yield; eliminated the need to use, distill and recover four solvents methylene chloride, tetrahydrofuran, toluene, and hexane; reduction in solvent usage from 250 to 25 litres per kilogram of sertraline |
9 |
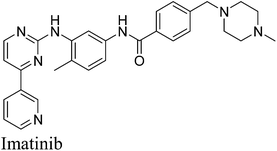 |
Anticancer |
Low yield |
High yield and convenient microwave synthesis |
10 |
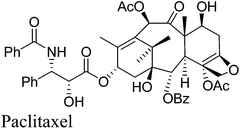 |
Anticancer |
Complex requiring 11 chemical transformations and 7 isolations; require 13 solvents along with 13 organic reagents and other materials |
Plant cell fermentation process; elimination of the eleven chemical transformations and a large amount of hazardous solvents and wastes |
11 |
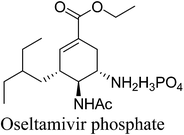 |
Anti-influenzatic |
Involves solvent extraction processes; use of thionyl chloride |
Single-step, higher yielding ionic liquid strategy |
12 |
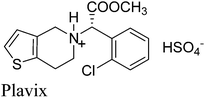 |
Antithrombotic agent |
Low yield; high raw material cost and environmental impact |
Only four steps; 98.3% ee and 88% total yield |
13 |
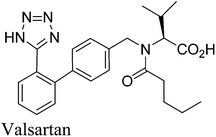 |
Anti-hypertensive |
Use of expensive boronic acid substrates in the cross-coupling step; use of halogenated solvents |
Involves heterogeneous Suzuki–Miyaura coupling step |
Case studies: green approaches in the pharmaceutical industry
Incorporating green chemistry into the synthesis of active pharmaceutical ingredients (APIs) and intermediates is of ongoing importance to the pharmaceutical industry. Solvent reduction and replacement, using heterogeneous catalysis and biocatalysis are some of the tools used to optimize select API syntheses. Moreover, academic researchers have seen benefits of using ionic liquids and microwave synthesis, which will hopefully lead to pharmaceutical companies adopting this strategy in the near future.
1. Atorvastatin (Lipitor)
Atorvastatin calcium (Fig. 1) is the active ingredient of Lipitor, the first drug in the world with annual sales to exceed $10 billion. It is a cholesterol-lowering drug that blocks the synthesis of cholesterol in the liver.11a This drug is an example of a competitive HMG-CoA-reductase inhibitor belonging to the 7-substituted 3,5-dihydroxyheptanoic acid family.
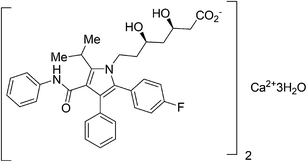 |
| Fig. 1 Structure of atorvastatin (Lipitor). | |
The key chiral building block in all the commercialized syntheses of atorvastatin is ethyl (R)-4-cyano-3-hydroxybutyrate 1 (Scheme 1). The initial routes used for the industrial production of 1 in all these processes11b–f involves reaction of an ethyl 3-hydroxy-4-halobutyrate with a cyanide ion in alkaline solution at high temperature (Scheme 2). What is more, these processes ultimately substitute cyanide for halide under heated alkaline conditions forming extensive by-products and require a challenging high-vacuum fractional distillation to purify the final product, lowering the yield even further. To address these issues for a practical and economical process, in 2010, S. K. Ma12 and co-workers developed a green, two-step, three-enzyme process for the synthesis of 1 (Scheme 3) in the manufacture of atorvastatin. The first step involves the biocatalytic reduction of ethyl-4-chloroacetoacetate 2 using a ketoreductase (KRED) in combination with glucose and a NADP-dependent glucose dehydrogenase (GDH) for cofactor regeneration. The (S) ethyl-4-chloro-3-hydroxybutyrate product 3 is obtained in 96% isolated yield and >99.5% ee. In the second step, a halohydrin dehalogenase (HHDH) is employed to catalyse the replacement of the chloro substituent with cyano by reaction with HCN at neutral pH and ambient temperature. This enabled the economical and environmentally attractive production of the key hydroxynitrile intermediate. The overall process has an E-factor (kg waste per kg product) of 5.8 when process water is not included, and 18 if included.
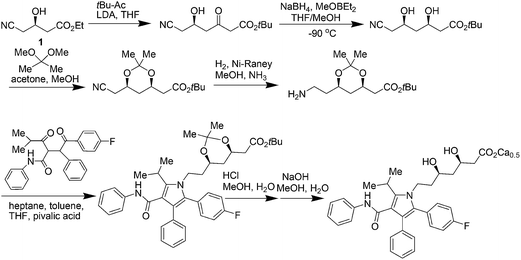 |
| Scheme 1 Commercialized synthetic routes for atorvastatin from ethyl (R)-4-cyano-3-hydroxybutyrate. | |
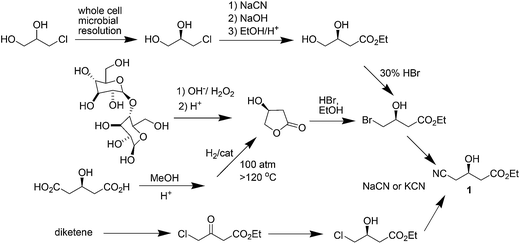 |
| Scheme 2 Synthetic routes for ethyl (R)-4-cyano-3-hydroxybutyrate. | |
 |
| Scheme 3 Enzymatic synthesis of atorvastatin. | |
2. Sitagliptin
Sitagliptin (Fig. 2) a selective, potent dipeptidyl peptidase-IV (DPP-IV) inhibitor, is the active ingredient in JANUVIA and JANUMET approved for the treatment of type 2 diabetes by FDA.13–16 The initial process chemistry route towards sitagliptin (1st generation process) was given by Hansen and co-workers in 2005.17a The first generation process was inefficient due to the poor atom economy of the reaction and large number of steps.17a However, Hansen et al.17b in 2009 reported a more efficient synthesis of sitagliptin14,16 (2nd generation process) which involves asymmetric hydrogenation of an enamine at high pressure [250 pounds per square inch (psi)] using a rhodium-based chiral catalyst (Scheme 4A). The Merck researchers later developed a ruthenium-catalyzed asymmetric direct reductive amination methodology for preparing unprotected β-amino amides from β-keto amides.17c This enables to set the chiral amine center in 9 with complete stereocontrol directly from 7 (Scheme 4B). Even though the transformation 7–8 is embedded in the one-pot sequence in the 2nd generation process, it still represents a unit operation for the process. This is eliminated in the direct reductive amination of 7–9, which represents an improvement over the 2nd generation process (Scheme 4B). The second generation synthesis shortened the steps required and almost halved the amount of waste produced; however there is still room for improvement because of the inherent drawbacks of utilizing a transition metal mediated hydrogenation step which necessitated the use of specialized high-pressure equipment and a process for the complete removal of the transition metal from the product stream both of which were significant cost drivers. Moreover, in the Rh-catalyzed process, the relatively low stereocontrol in the asymmetric hydrogenation step (95% ee) necessitated the incorporation of an additional crystallization to obtain optically pure 10.
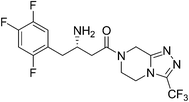 |
| Fig. 2 Structure of sitagliptin. | |
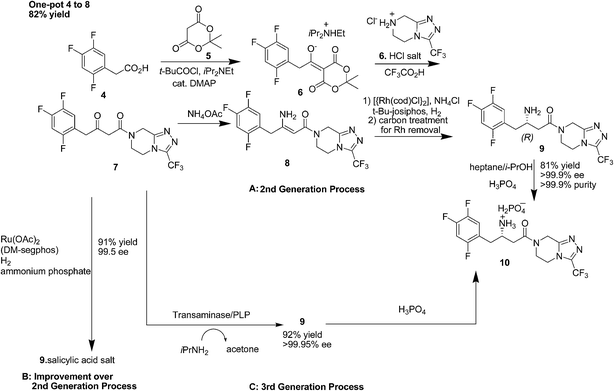 |
| Scheme 4 (A) Second generation process for sitagliptin using rhodium based catalyst. (B) Second generation process for sitagliptin using ruthenium based catalyst. (C) Third generation process for sitagliptin using transaminase biocatalyst. | |
In 2010, Savile et al.18 reported the third and current generation synthesis (Scheme 4C) of Januvia using a transaminase biocatalyst instead of a rhodium metal resulting in fewer steps, a better yield, and a more pure final product. The third generation's environmentally friendly route was made possible by the production of a transaminase biocatalyst devised by Merck and Codexis.19 Starting with the β-keto amide, which was isolated in the second generation (Scheme 4A) to create an improvement to the second generation synthesis, this same isolated molecule could now be treated with the newly created transaminase catalyst. The transaminase product that was formed still had to be crystallized by H3PO4 acid to produce the sitagliptin phosphate, but overall this synthesis was greener than that of the synthesis seen in the second generation. Additionally, the third generation reduced the overall waste from the second generation by 19%. With the biocatalyst, all previously stated drawbacks seen in the second generation were eliminated with the third generation. Specifically, the third generation eliminated the need for the high pressure hydrogenation device or heavy metals. Without the device or the metal,18,19 the manufacturing cost of the drug was able to decrease as well.
With each subsequent generation, the evolution of sitagliptin's synthesis has led to a “greener” and more effective synthesis. The third and final generation of sitagliptin fulfills eight of the twelve principles of sustainable chemistry. The first principle of green chemistry involves waste prevention and the third generation reduces the amount of superfluous waste and completely eliminates the need for aqueous waste streams. Limiting the amount of waste per product weight is crucial in keeping drug cost and environmental impact to a minimum. Moreover, the third generation conducts less hazardous chemical syntheses and these syntheses are performed under constant temperature and pressure. Additionally, the third generation allows for nearly perfect optical and chemical purity using an engineered biocatalyst to replace the heavy metal rhodium catalyst. The need for high-pressure hydrogenation devices was also eliminated. The third generation only requires three steps and increased the overall yield significantly, which fulfils the eighth principle of reducing extraneous derivatives.
3. Sildenafil citrate (Viagra)
Sildenafil citrate (Viagra) (Fig. 3) is the first oral treatment for the male erectile dysfunction, an illness that affects million men and their partners world wide. This drug works by inhibiting the phosphodiesterase enzyme (PDE5) found in the human corpus cavernosum which regulates the level of cyclic guanosine monophosphate (cGMP) by its conversion to guanosine monophosphate (GMP). The inhibition of PDE5 leads to higher levels of cGMP which in turn leads to improved smooth muscle relaxation, increased blood flow, and hence an improved erection.20a,b
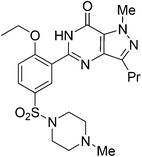 |
| Fig. 3 Structure of sildenafil citrate (Viagra). | |
The first medicinal chemistry route developed for its synthesis20c–f is outlined in Scheme 5. This method was not suitable for large scale manufacturing and the following changes were made in the later reported optimised medicinal chemistry route to improve the environmental performance of the synthesis:
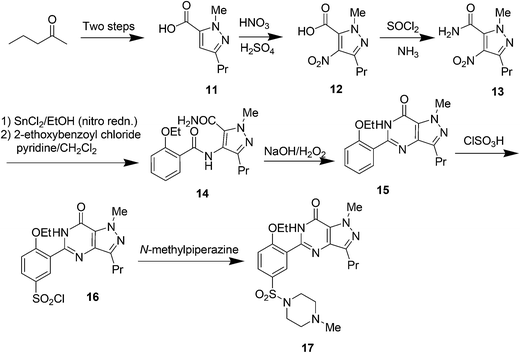 |
| Scheme 5 Medicinal chemistry route developed for the synthesis of sildenafil citrate. | |
• Replacement of tin chloride which is a major environment polluter with catalytic hydrogenation reduction.
• The use of stoichiometric quantities of thionyl chloride as solvent, rather than thionyl chloride as solvent in the preparation of compound 13.
• KOBut in tBuOH was used to cyclize 14–15 with 100% isolated yield with no impurities in place of hydrogen peroxide which causes burns on contact with skin and can cause fire especially in contact with organic materials.
• The use of thionyl chloride rather than oxalyl chloride for the preparation of 2-ethoxybenzoyl chloride. This change gave an improvement in worker safety by avoiding carbon monoxide emissions.
The synthesis of Viagra was later redesigned to introduce convergency and clean cyclization reaction at the final step resulting in the formation of very clean product (Scheme 6).21 Firstly, amine 18 is prepared from 12 as mentioned earlier in the optimized synthesis and 20 is prepared from 2-ethoxybenzoic acid, 19. 2-Ethoxybenzoic acid is chlorosulfonated using chlorosulfonic acid along with 1 mol of thionyl chloride to ensure that the intermediate sulfonic acid is converted to the sulfonyl chloride followed by aqueous quench to give water wet sulfonyl chloride. For simplicity and efficiency, the sulfonyl chloride is then again suspended in water and reacted with N-methylpiperazine to give sulfonamide (20). Hence, no organic solvent is used for the preparation of the sulfonamide. Reaction of 20 with 18 was then done with N,N′-carbonyldiimidazole (CDI) to get coupled product 21 despite being expensive because it provided clean, robust chemistry and high quality product; allowed all three reactions (hydrogenation, activation and acylation) to be combined; enabled a single solvent to be employed (ethyl acetate), allowing simple solvent recovery with low energy use; avoided VOC emissions (EtCl) from the interaction of either thionyl chloride or oxalyl chloride with ethyl acetate and high chemical yield (90%) over three chemical steps (subsequently optimised to 96%) which (21) is then cyclized with t-BuOH and t-BuOK. The overall yield of sildenafil citrate had risen to 75%, and the high yields late in the synthesis minimised the environmental impact of the earlier steps.
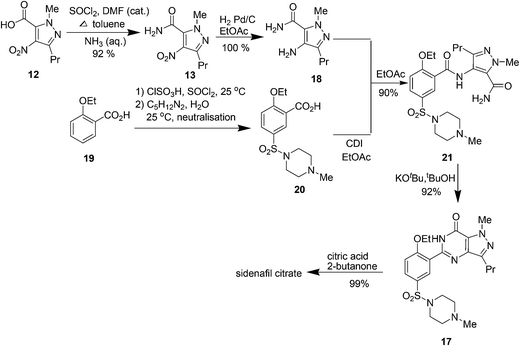 |
| Scheme 6 Convergent synthesis of sildenafil citrate. | |
4. Pregabalin (Lyrica)
(S)-(+)-3-Aminomethyl-5-methylhexanoic acid (pregabalin) (Fig. 4) is a lipophilic GABA (γ-aminobutyric acid) analogue that was developed for the treatment of several central nervous system disorders including epilepsy, neuropathic pain, anxiety and social phobia.22,23a
 |
| Fig. 4 Structure of pregabalin (Lyrica). | |
The first generation manufacturing process23b (Scheme 7) for the synthesis of pregabalin began with the Knoevenagel condensation of isovaleraldehyde and diethyl malonate, followed by cyanation to give the key intermediate 22, which was established as the regulatory starting material. Compound 22 was then converted to racemic 3-aminomethyl-5-methylhexanoic acid in a three-step sequence that included hydrolysis, reduction, and decarboxylation, all performed in one pot with a single isolation step. The crude racemic pregabalin was then resolved using (S)-(+)-mandelic acid in a three-step crystallization process and the overall yield of the process was only about 20%. This chemical process suffers from high process mass intensity (raw material input/API output) and high manufacturing costs because the undesired γ-amino acid enantiomer could not be recycled.23b
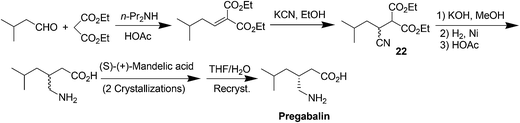 |
| Scheme 7 First generation manufacturing process for pregabalin involving Knoevenagel condensation. | |
To address the green chemistry and cost issues, both chemocatalysis and biocatalysis have been applied to develop more efficient second-generation routes.24–27 Among them, the greenest one is a biocatalytic method which involves a lipolase-catalyzed resolution of a cyano diester (CNDE) to produce the desired (S)-mono acid enantiomer in high resolution yields (45%) and enantioselectivity (98% ee) which was subsequently transformed to pregabalin upon decarboxylation, hydrolysis and hydrogenation (Scheme 8).27 The enzymatic step has an excellent volumetric activity of >500 g per L per d. Since the undesired (R)-enantiomer could be readily racemized to CNDE, the yield of the process was improved to 40% after one recycling doubling the yield of the chemical route. Moreover, all three GMP (Good Manufacturing Practice) steps after CNDE were conducted in water and intermediates were telescoped. As a result, the biocatalytic process is significantly greener than the chemical route with the E-factor being reduced from 86 to 17.27 It was projected that over 10 million gallons of alcoholic organic solvents and nearly 2000 metric tons of raw materials (mandelic acid, CNDE, Ni) were eliminated annually by adopting this biocatalytic route for pregabalin.
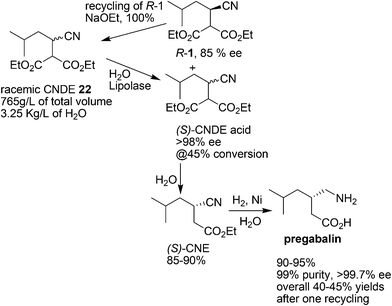 |
| Scheme 8 Biocatalytic route for the synthesis of pregabalin. | |
5. β-Lactam antibiotics (ampicillin, amoxicillin, cefaclor, cephalexin and cefadroxil)
β-Lactam antibiotics are a broad class of antibiotics consisting of antibiotic agents that contains a β-lactam ring in their molecular structure. This includes ampicillin, amoxicillin, cefaclor, cephalexin and cefadroxil. Initially, they were prepared from penicillin G, a fermentation product derived from non-ribosomal peptide synthase (NRPS) by chemical deacylation where the carboxy group of the penicillin G was first protected by silylation, followed by selective deacylation via the imidoyl chloride and removal of the protecting group.28 The method uses stoichiometric amounts of the silylating agent and a large amount of hazardous chemicals and solvents such as phosphorus pentachloride and dichloromethane.
In contrast, Bruggink and co-workers29 gave a biocatalytic route for the synthesis of β-lactam antibiotics using penicillin G acylase. It involves enzymatic deacylation of penicillin G by penicillin G acylase in water at room temperature requiring no protection and deprotection of other functional groups (8th principle of green chemistry, Scheme 9). Under kinetic control, an immobilized penicillin aclyase was able to catalyze the acylation of 6-APA (6-aminopenicillanic acid) and 7-ADCA (7-aminodesacetoxycephalosporanic acid) with either D-phenylglycine methyl ester, D-phenylglycine amides or their para hydroxylated analogs to produce a wide range of semi-synthetic β-lactam antibiotics such as ampicillin, amoxicillin, cefaclor, cephalexin and cefadroxil (Scheme 9).29,30 Raw material efficiency and E-factor were significantly improved in biotransformation route as compared to first generation chemical process.
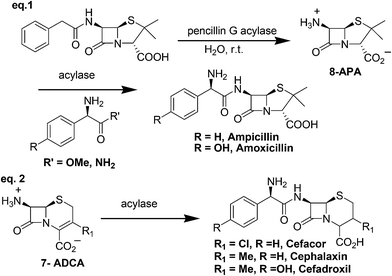 |
| Scheme 9 Biocatalytic route for the synthesis of β-lactam antibiotics. | |
6. Simvastatin
Simvastatin (Fig. 5) is a cholesterol-lowering drug marketed by Merck as Zocor®. The primary uses of simvastatin are for the treatment of dyslipidemia and the prevention of cardiovascular disease.31a It is a semisynthetic compound derived from the natural product lovastatin which is a secondary metabolite produced by the filamentous fungus Aspergillus terreus.31b–e
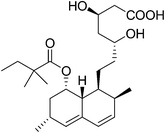 |
| Fig. 5 Structure of simvastatin. | |
Initially, two semisynthetic routes reported by Hoffman et al.32 (in 1986) and Auskin et al.33 (in 1991), were widely used to synthesize simvastatin starting from lovastatin (Scheme 10). One commonly adapted process32 starts with the hydrolysis of lovastatin to yield the key intermediate monacolin J, followed by the lactonization of the acid to protect the C11 hydroxyl group and trimethylsilylation protection of the C13 hydroxyl. The protected monacolin J was then subjected to acylation by α-dimethylbutyryl chloride to yield the protected form of simvastatin, which was subsequently deprotected to yield simvastatin. Both multistep processes shown in Scheme 10 are laborious and are nearly five times more expensive than lovastatin. In 2007, Yi Tang and his group34 reported a whole-cell biocatalytic process (Scheme 11) that is able to convert monacolin J to simvastatin in a highly efficient manner. By using a novel thioester as the acyl donor (LovD) that selectively transfers the 2-methylbutyryl side chain to the C8 alcohol of monacolin J sodium or ammonium salt it was possible to achieve >99% conversion of simvastatin from monacolin J in a single step. The fermentation process can be easily scaled up to produce an industrial-scale yield of simvastatin.
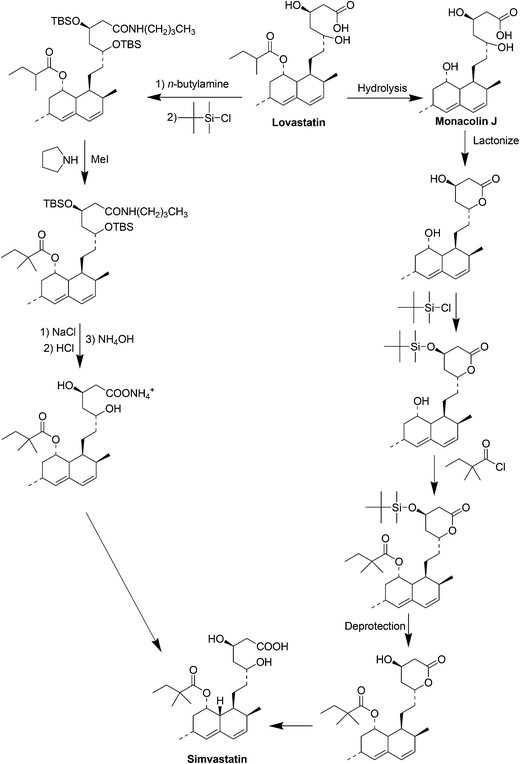 |
| Scheme 10 Semisynthetic routes reported for simvastatin starting from lovastatin. | |
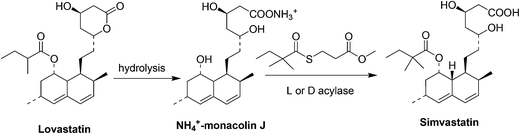 |
| Scheme 11 Biocatalytic route for the synthesis of simvastatin. | |
Codexis licensed this process from UCLA (University of California, Los Angeles) subsequently optimized the enzyme and the chemical process for commercial manufacture, carried out nine iterations of in vitro evolution, creating 216 libraries, screening 61
779 variants to develop a LovD variant with improved activity, in-process stability and tolerance to product inhibition.34 The approximately 1000-fold improved enzyme and the new process pushed the reaction to completion at high substrate loading and minimized the amounts of acyl donor and of solvents for extraction and product separation.
7. Saxagliptin (Onglyza)
Saxagliptin35a (Fig. 6), a dipeptidyl peptidase-IV (DPP-IV) inhibitor's early development was solely by Bristol-Myers Squibb; in 2007 AstraZeneca joined with Bristol-Myers Squibb to co-develop the final compound and collaborate on the marketing of the drug. In June 2008, it was announced that Onglyza would be the trade name under which saxagliptin will be marketed.35b AstraZeneca took full control of the franchise last year in a $4.3bn (£2.6bn) deal.
 |
| Fig. 6 Structure of saxagliptin (Onglyza). | |
Saxagliptin requires (S)-N-BOC-3-hydroxyadamantylglycine 25 as an intermediate for its synthesis and this compound was originally prepared using an asymmetric Strecker amino acid synthesis which involves the use of highly toxic potassium cyanide (Scheme 12).35a By using a modified phenylalanine dehydrogenase, the Bristol-Myers Squibb (BMS) enzyme technology group36 successfully set the chiral center in 24 by an enzymatic reductive amination of keto acid 23 (Scheme 13), reducing the number of steps from five to only one by redesigning the process chemistry, eliminating the need for cyanide and the expensive chiral reagent, (R)-(−)-2-phenylglycinol, poor oxidation using potassium permanganate in the final step, and this reductive amination used water as solvent.
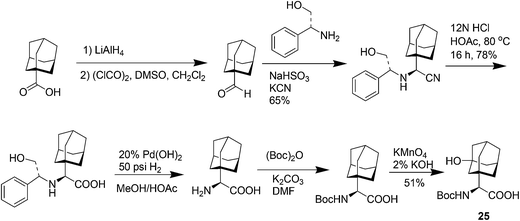 |
| Scheme 12 Synthesis of saxagliptin involving asymmetric Strecker reaction. | |
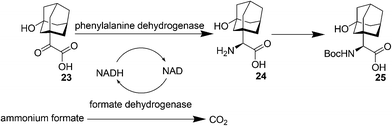 |
| Scheme 13 Enzymatic synthesis of saxagliptin. | |
8. Sertraline (Zoloft)
Sertraline hydrochloride (Fig. 7) is an inhibitor of synaptosomal serotonin uptake, an important pharmaceutical agent for the treatment of depression as well as dependency and other anxiety-related disorders.37a–c
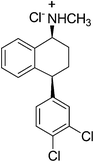 |
| Fig. 7 Structure of sertraline (Zoloft). | |
The original synthesis of sertraline37c involved Stobbe reaction of benzophenone with diethylsuccinate to yield a mono acid (Scheme 14) followed by hydrolysis and decarboxylation under strongly acidic conditions producing butenoic acid which was hydrogenated over a palladium catalyst to the desired 4,4-diarylbutanoic acid 29. Subsequent Friedel–Crafts acylation and cyclization yielded the key racemic tetralone intermediate 30. Condensation of tetralone with methylamine in the presence of titanium tetrachloride followed by catalytic reduction of the imine gave a mixture of cis and trans amines 31. The cis form was purified as its HCl salt by fractional crystallization and was subsequently resolved with d-(−)mandelic acid to produce the desired (−)-(1S, 4S)-sertraline 32. There are several drawbacks of this method, firstly, it required four steps to establish the skeleton of the racemic tetralone, certainly a shorter route existed for this relatively simple compound. Secondly, the Friedel–Crafts acylation required excess AlCl3 and was carried out in the hazardous solvent carbon disulfide. Finally, classic resolution using a chiral salt was required in the final step, requiring significant resources for carrying along the unwanted stereoisomer throughout the entire synthetic sequence. In 2004, Taber et al.37d reported a green process for the synthesis of sertraline (Scheme 15). This process (referred to as the “combined” process) offers substantial pollution prevention benefits including improved safety and material handling, reduced energy and water use, and doubled overall product yield. Specifically, a three-step sequence in the original manufacturing process was streamlined to a single step in the new sertraline process. The condensation reaction between 4-(3,4-dichlorophenyl)-3,4-dihydro-1(2H)-naphthalone, sertraline tetralone (30), and monomethylamine was carried out in ethanol without the need for classical dehydrating agent, such as TiCl4 or more novel approaches such as molecular sieves, both of which produce hazardous by products and solid wastes. The low solubility of the imine 33 in this type of solvent is exploited such that the reaction equilibrium favorably enhances the imine formation. Furthermore, an improved and highly selective catalytic reduction of 33 with Pd/CaCO3 catalyst in ethanol as the reaction solvent followed by the resolution of the racemic cis isomer with d-(−)-mandelic acid results in a more efficient telescoped commercial process to (1S-cis)-4-(3,4-dichlorophenol)-1,2,3,4-tetrahydro-N-methyl-1-naphthalenamine mandelate, sertraline mandelate (32). The process also eliminated the need to use, distilled and recover four solvents (methylene chloride, tetrahydrofuran, toluene, and hexane) and resulted in a reduction in solvent usage from 250 to 25 litres per kilogram of sertraline.
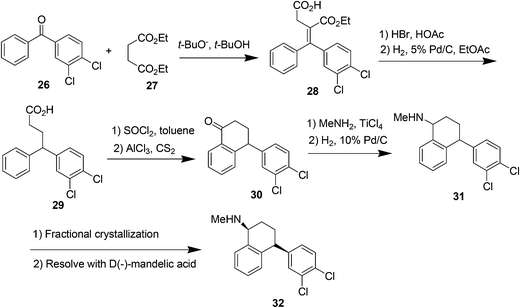 |
| Scheme 14 Synthesis of sertraline involving Stobbe reaction. | |
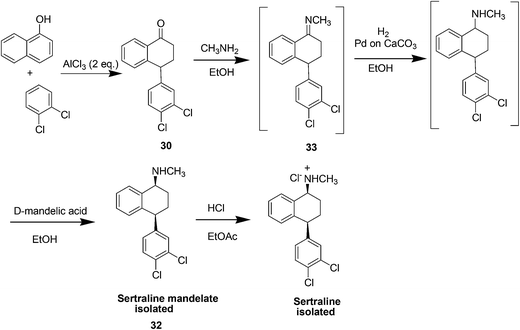 |
| Scheme 15 Green (combined) process for the synthesis of sertraline. | |
9. Imatinib (Gleevec)
Imatinib (Gleevec, Fig. 8) is a potent and selective inhibitor of BCR-ABL and c-kit oncogenic tyrosine kinase, approved by the Food and Drug Administration for the chemotherapy of chronic myeloid leukemia and gastrointestinal stromal tumor.38,39 Imatinib has become a paradigm for molecular targeted cancer therapy and acquired soon the status of a blockbuster drug owing to its outstanding therapeutic efficacy and low toxicity profile.40 Unfortunately, in many patients with advanced diseases a harmful drug resistance frequently develops after an initial positive response to imatinib.41 Therefore, the development of an efficient synthetic method for the synthesis of imatinib may pave the way to build focused libraries of new, more potent and selective BCR-ABL inhibitors possibly active against the most common life-threatening resistant mutants.
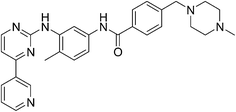 |
| Fig. 8 Structure of imatinib (Gleevec). | |
The first synthetic pathway for imatinib was described by Zimmermann and co-workers in 1993.42,43 In 2012, Kompella44 and co-workers reported an efficient and economic process for the production of imatinib with 99.99% purity and 50% overall yield from four steps by considering Zimmermann and co-workers' route as the basis. The various steps involved in the protocol are:
• Reacting 2-methyl-5-nitroaniline with cyanamide in the presence of hydrochloric acid followed by neutralization to obtain 1-(2-methyl-5-nitrophenyl)guanidine (34).
• Reacting 3-(dimethylamino)-1-(3-pyridinyl)-prop-2-en-1-one (35) with 34 to obtain N-(5-nitro-2-methylphenyl)-4-(3-pyridinyl)-2-pyridineamine (36).
• Reducing 36 in the presence of Raney nickel to obtain N-(5-amino-2-methylphenyl)-4-(3-pyridinyl)-2-pyrimidineamine (37).
• Condensing 37 with 4-(4-methylpiperazino)methyl benzoyl chloride dihydrochloride (38) in the presence of an inorganic base to give imatinib, 39 (Scheme 16).
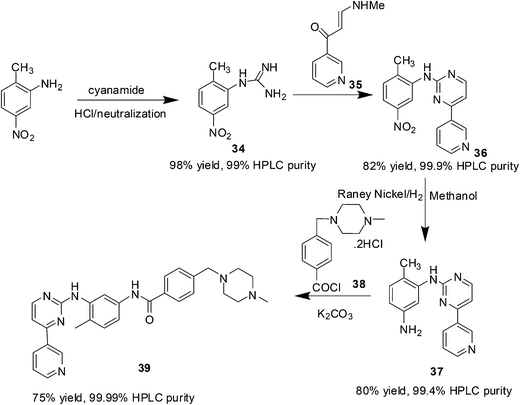 |
| Scheme 16 Efficient and economic synthetic route for imatinib. | |
10. Paclitaxel
Paclitaxel (Fig. 9) has been investigated as having significant anticancer activity against various tumors and is currently prescribed worldwide to treat the most aggressive forms of ovarian, lung and breast cancer as well as AIDS-related Kaposi's sarcoma.45–49
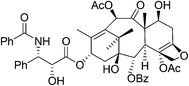 |
| Fig. 9 Structure of paclitaxel. | |
It is a complex diterpenoid alkaloid originally isolated from the bark of the pacific yew tree Taxus brevifolia with a yield of 0.014%.45,50 In addition, isolating paclitaxel required stripping the bark from the yew trees killing them in the process. Yews take 200 years to mature and are part of a sensitive ecosystem. The complexity of the paclitaxel molecule makes commercial production by chemical synthesis from simple compounds impractical.
As a result, a semisynthetic process was developed starting from 10-deacetylbaccatin III a more abundant taxoid biosynthesized from isoprenyl diphosphate and farnesyl diphosphate in the needles of the European yew tree Taxus baccata51 which could be isolated with a yield of 0.1% without harm to the trees. The semisynthetic process is complex requiring 11 chemical transformations and 7 isolations and also presents environmental concerns requiring 13 solvents along with 13 organic reagents and other materials. Consequently, an alternative process to paclitaxel was developed using Taxus cell fermentation and extraction from culture medium, followed by recrystallization. Starting from the two isoprenoid precursors, isoprenyl diphosphate and farnesyl diphosphate, geranylgeranyl pyrophosphate synthetase catalyzes the coupling to give geranylgeranyl pyrophosphate 40 which is then cyclized and then converted to baccatin III 41 through a series of enzymatic transformations including hydroxylation, acylation, oxidation and generation of the oxetane ring. The side chain in 42 was installed by enzymatic transfer of phenylisoserine (Scheme 17).52,53 To achieve a high titer of paclitaxel production, cell cultures from various species of Taxus and different elicitors to induce paclitaxel production have been examined.54 The plant cell fermentation process led to an elimination of the eleven chemical transformations and a large amount of hazardous solvents and wastes, which came with the semi-synthesis route.55
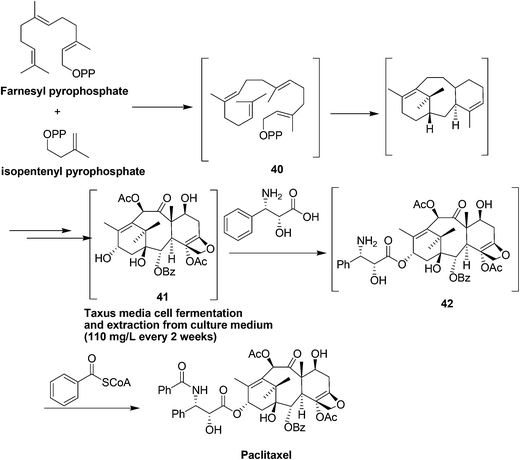 |
| Scheme 17 Enzymatic process for the synthesis of paclitaxel. | |
11. Oseltamivir phosphate
Tamiflu™ (oseltamivir phosphate, Fig. 10) is well known for the treatment and prevention of influenza.56,57 Extraction of active ingredients from plant material is mainly performed using solvent extraction processes; however this is often associated with the dangers of handling large volumes of volatile and combustible solvents, human risk and safety issues and also with poor extraction efficiency that can be the bottleneck in a drug's production.58 This is particularly true for shikimic acid ((3R,4S,5R)-3,4,5-trihydroxycyclohex-1-ene-1-carboxylic acid), the major starting material for the production of Tamiflu™. Although many alternative fermentation processes for shikimic acid have been developed, the production of oseltamivir phosphate was still dependent on the isolation of shikimic acid 43 from Chinese star anise seeds (Illicium verum) and the isolated yield was as low (about 3–7%). The initial esterification step in the synthesis of Tamiflu was typically achieved using stoichiometric amounts of toxic and corrosive thionyl chloride for the generation of anhydrous hydrochloric acid as catalyst. The toxicity of thionyl chloride as well as the formation of greenhouse gases after hydrolization raises serious safety and environmental concerns. In 2008, Roche co-workers reported process for the direct formation of ketal intermediate 45 without thionyl chloride by using the preformed reagent 3,3-diethoxypentane (Scheme 18).59,60 However, it should be noted that this optimized procedure requires the use of pure shikimic acid in rather high and consistent quality. In 2011, Rassmann and co-workers61 present an ionic liquid strategy for the reactive dissolution of star anise seeds in the presence of Bronsted-acidic ILs as solvent and catalyst for the formation of shikimic acid ethyl ester 44 and for the in situ formation of ketal ester 45 to develop an improved process for the synthesis of oseltamivir phosphate (Tamiflu™) (Scheme 19). This novel strategy provides a single-step, higher yielding and environmentally benign strategy for the manufacturing of the anti-influenza drug Tamiflu™.
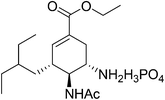 |
| Fig. 10 Structure of oseltamivir phosphate. | |
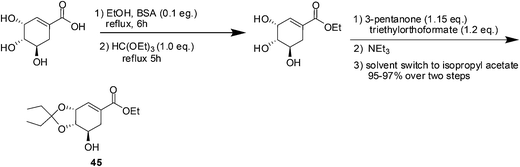 |
| Scheme 18 Synthesis of ketal ester (intermediate in the synthesis of oseltamivir phosphate) without using thionyl chloride. | |
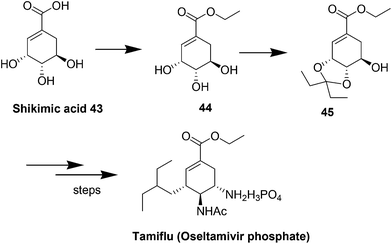 |
| Scheme 19 Improved synthesis of oseltamivir phosphate involving ionic-liquid strategy. | |
12. Plavix
(S)-(+)-Clopidogrel bisulfate (Plavix, Fig. 11) is an orally active inhibitor of platelet aggregation now marketed as an antithrombotic agent which was licensed by Sanofi in 1986 and works by preventing harmful blood clots.62 It is an adenosine diphosphate (ADP) receptor antagonist indicated for the reduction of atherosclerotic events including myocardial infarction, ischemic stroke, and vascular death in patients with atherosclerosis.
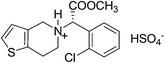 |
| Fig. 11 Structure of plavix. | |
Initially, there were mainly two kinds of syntheses of clopidogrel. One was from the derivatives of R-halogenphenyl acetate by alkylation with 4,5,6,7-tetrahydrothieno[3,2-c] pyridine, 48.63 The second was from the derivatives of R-aminophenylacetic acid.64 Both these methods suffered several industrial inconveniences including unsatisfactory yield, efficiency, high raw material cost and environmental impact.
In 2007, Wang et al.65 gave a improved green synthesis of clopidogrel which was accomplished in four steps in one-pot in above 70% overall yield. The synthesis of clopidogrel starting from 2-(2-chlorophenyl)-2-(6,7-dihydrothieno[3,2-c]pyridine-5(4H)-yl)acetonitrile, 49, which is a key intermediate in the process can be cheaply and effectively prepared from commercially available 48 and R-bromo-2-chlorophenyl acetonitrile, 47, which is prepared from the bromination of 2-chlorophenyl acetonitrile 46 (Scheme 20). The direct alkaline hydrolysis of nitrile 49 to acid 51 could be accomplished almost quantitatively in the mixed solvent and high concentration of inorganic strong base in the presence of phase transfer catalyst whereas by controlling the concentration of base (<20%), amide 50 may also be obtained selectively. l-Camphor sulphonic acid (0.45–0.55 equivalent) was used in toluene and a highly selective and effective kinetic resolution method of racemic clopidogrel in above 98.3% ee and 88% total yield was obtained.
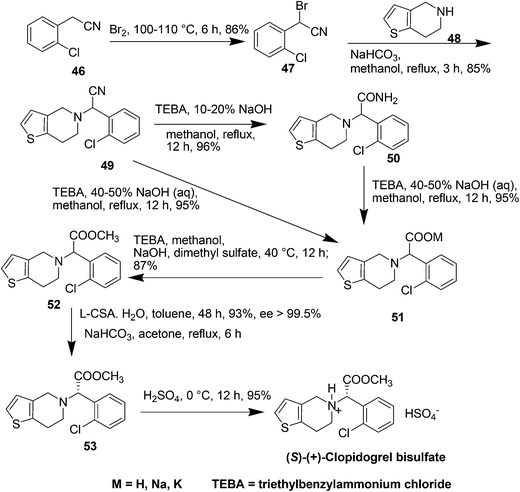 |
| Scheme 20 Green synthetic pathway for plavix. | |
13. Valsartan
Valsartan (Fig. 12) is a member of a class of compounds known as angiotensin II (AT-II) receptor antagonists. This class combines effective anti-hypertensive activity with an excellent profile of safety and tolerability. Activation of AT-II receptors in the outer membrane of vascular smooth muscle cells of the heart and arteries causes the tissues to constrict. AT-II helps to maintain constant blood pressure66 despite fluctuations in ones state of hydration, sodium intake and other physiological variables and also performs the regulatory tasks of inhibiting the excretion of sodium by the kidneys, inhibiting norephedrine re-uptake and stimulating aldosterone biosynthesis. By inhibiting the actions of AT-II on its receptors, valsartan67,68 prevents the increase of blood pressure produced by the hormone–receptor interactions and is used in the treatment of cardiovascular complaints such as hypertension and heart failure. Valsartan is marketed as the free acid under the trade name Diovan®.
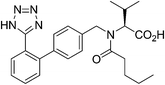 |
| Fig. 12 Structure of valsartan. | |
The multistep valsartan/hydrochlorothiazide production process was originally published68 in 1994. The biphenyl unit is a common structural element in all sartans and its formation represents the key step in the synthesis of sartans: the published methods for the preparation of valsartan make use of Suzuki–Miyaura couplings.69–71 The principal synthetic pathways leading to valsartan depicted in Scheme 21 have common shortcoming of using expensive boronic acid substrates in the cross-coupling step. In 2007, Beutler and co-workers redesigned the three chemical steps of the original synthesis increasing the throughput of the process and eliminating the use of halogenated solvents72 and Goossen and co-workers reported a four step valsartan synthesis using decarboxylative coupling.73 In 2010, Gosh and co-workers reported short and concise valsartan synthesis employing Negishi coupling of oxazoline moieties in place of more expensive coupling involving organoboronic acid.74 In a recent approach for greening the valsartan synthesis (Scheme 22), Pandarus et al.75 in 2013 reported the heterogeneous Suzuki–Miyaura coupling reaction in batch conditions between 2-chlorobenzonitrile and 4-tolylboronic acid, to produce 4-methyl-2-biphenylcarbonitrile over the organosilica matrix functionalized with diphenylphosphine ligands bound to Pd(II) (SiliaCat DPP-Pd) catalyst in ethanol.
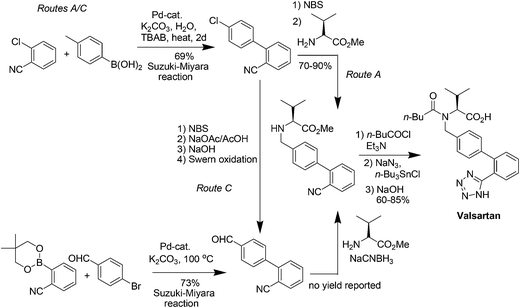 |
| Scheme 21 Synthetic pathways for valsartan. | |
 |
| Scheme 22 Green synthesis of valsartan involving heterogeneous Suzuki–Miyaura coupling. | |
Conclusions
All these developments in the pharmaceutical industries showed that green chemistry and green engineering principles are spreading to the most efficient chemical industry. The new methods of green chemistry have positive results in the pharmaceutical industry because their R & D investment is very robust and can cover research expenses and support innovative ideas. Scientists believe that green chemistry is going to transform the pharmaceutical industry and drug manufacturing in the future. Although green chemistry philosophy has been generally accepted by the scientific community, technical green chemistry evolution through education and investment has yet to achieve the appropriate attention and effort.
References
-
(a) http://www.pharma-mag.com;
(b) M. Butters, D. Catterick, A. Craig, A. Curzons, D. Dale, A. Gillmore, S. P. Green, I. Marziano, J.-P. Sherlock and W. White, Chem. Rev., 2006, 106, 3002 CrossRef CAS PubMed.
- D. J. C. Constable, P. J. Dunn, J. D. Hayler, G. R. Humphrey, J. L. Leazer Jr, R. J. Linderman, K. Lorenz, J. Manley, B. A. Pearlman, A. Wells, A. Zaks and T. Y. Zhang, Green Chem., 2007, 9, 411 RSC.
- T. Y. Zhang, Chem. Rev., 2006, 106, 2583 CrossRef CAS PubMed.
- Practical Process Research & Development, ed. N. G. Anderson, Academic Press, San Diego, CA, 2000, p. 81 Search PubMed.
- Green Solvents for Chemistry; Perspectives and Practice, ed. W. M. Nelson, Oxford University Press, New York, 2003 Search PubMed.
-
(a) Green Chemistry in the Pharmaceutical Industry, ed. P. Dunn, A. Wells and M. T. Williams, Wiley-VCH Verlag, Chichester, West Sussex, UK, Weinheim, New York, 2010 Search PubMed;
(b) Sustainable Industrial Chemistry, ed. F. Cavani, G. Centi, S. Parathoner and F. Trifino, Wiley-VCH, Chichester, West Sussex, UK, 2009 Search PubMed.
-
(a) J. M. Fortunak, P. N. Confalone and J. A. Grosso, Curr. Opin. Drug Discovery Dev., 2007, 10, 651 CAS;
(b) J. M. Fortunak, Future Med. Chem., 2009, 1, 571 CrossRef CAS PubMed;
(c) O. A. Jones, J. N. Lester and N. Voulvoulis, Trends Biotechnol., 2005, 23, 163 CrossRef CAS PubMed;
(d) G. B. Derksen and R. H. Jongbloed, Water Sci. Technol., 2004, 49, 213 Search PubMed;
(e) B. Kasprzyk-Hordem, R. M. Dinsdale and A. J. Guwy, Water Res., 2008, 42, 3498 CrossRef PubMed.
-
(a) R. A. Sheldon, Chem. Ind., 1992, 903 CAS;
(b) R. A. Sheldon, Green Chem., 2007, 9, 1273 RSC;
(c) B. Trost, Science, 1991, 254, 1471 CAS;
(d) R. A. Sheldon, presented at the International Symposium on Catalytic Chemistry for the Global Environment, Sapporo, Japan, July, 1991 Search PubMed.
-
(a) C. Jimenez-Gonzalez, C. S. Ponder, Q. B. Broxterman and J. B. Manley, Org. Process Res. Dev., 2011, 15, 912 CrossRef CAS;
(b) ISO 14044, Environmental management -Life cycle assessment-Requirements and Guidelines, International Organization of Standardization, Geneva, Switzerland, 2006;
(c) H. Wenzel, M. Hauschild and L. Alting, Environmental Assessment of Products; Vol. 1: Methodology, Tools and Case Studies in Product Development, Kluwer Academic Publishers: Norwell, MA, 1997 Search PubMed;
(d) C. Jimenez-Gonzalez and D. J. C. Constable, Green Chemistry and Engineering: A Practical Design Approach, John Wiley and Sons, New York, 2011 Search PubMed.
-
(a) GlaxoSmithKline 2010 Corporate Responsibility Report, 2011, http://www.gsk.com/responsibility/;
(b) A. D. Little and GlaxoSmithKline, Value Chain Carbon Emissions. High Level Estimation and Hotspot Identification: Technical Report, Cambridge, UK, November 2010 Search PubMed.
-
(a) M. Müler, Angew. Chem., Int. Ed., 2005, 44, 362 CrossRef PubMed;
(b) D. E. Butler, T. V. Le, A. Millar and T. N. Nanninga, US5155251, 1992;
(c) G. DeSantis, Z. Zhu, W. A. Greenberg, K. Wong, J. Chaplin, S. R. Hanson, B. Farwell, L. W. Nicholson, C. L. Rand, D. P. Weiner, D. E. Robertson and M. J. Burk, J. Am. Chem. Soc., 2002, 124, 9024 CrossRef CAS PubMed;
(d) G. DeSantis, K. Wong, B. Farwell, K. Chatman, Z. Zhu, G. Tomlinson, H. Huang, X. Tan, L. Bibbs, P. Chen, K. Kretz and M. J. Burk, J. Am. Chem. Soc., 2003, 125, 11476 CrossRef CAS PubMed;
(e) B. H. Hoff and T. Anthonsen, Tetrahedron: Asymmetry, 1999, 10, 1401 CrossRef CAS;
(f) D. R. Yazbek, C. A. Martinez, S. Hu and J. Tao, Tetrahedron: Asymmetry, 2004, 15, 2757 CrossRef PubMed.
- S. K. Ma, J. Gruber, C. Davis, L. Newman, D. Gray, A. Wang, J. Grate, G. W. Huisman and R. A. Sheldon, Green Chem., 2010, 12, 81 RSC.
- D. Kim, L. Wang, M. Beconi, G. J. Eiermann, M. H. Fisher, H. He, G. J. Hickey, J. E. Kowalchick, B. Leiting, K. Lyons, F. Marsilio, M. E. McCann, R. A. Patel, A. Petrov, G. Scapin, S. B. Patel, R. S. Roy, J. K. Wu, M. J. Wyvratt, B. B. Zhang, L. Zhu, N. A. Thornberry and A. E. Weber, J. Med. Chem., 2005, 48, 141 CrossRef CAS PubMed.
- D. M. Kendall, R. M. Cuddihy and R. M. Bergenstal, Eur. J. Intern. Med., 2009, 20, S329 CrossRef CAS PubMed.
- G. A. Herman, P. P. Stein, N. A. Thornberry and J. A. Wagner, Clin. Pharmacol. Ther., 2007, 81, 761 CrossRef CAS PubMed.
- D. Williams-Herman, E. Round, A. S. Swern, B. Musser, M. J. Davies, P. P. Stein, K. D. Kaufman and J. M. Amatruda, BMC Endocr. Disord., 2008, 8, 14 CrossRef PubMed.
-
(a) K. B. Hansen, J. Balsells, S. Dreher, Y. Hsiao, M. Kubryk, M. Palucki, N. Rivera, D. Steinhuebel, J. D. Armstrong III, D. Askin and E. J. J. Grabowski, Org. Process Res. Dev., 2005, 9, 634 CrossRef CAS;
(b) K. B. Hansen, Y. Hsiao, F. Xu, N. Rivera, A. Clausen, M. Kubryk, S. Krska, T. Rosner, B. Simmons, J. Balsells, N. Ikemoto, Y. Sun, F. Spindler, C. Malan, E. J. J. Grabowski and J. D. Armstrong III, J. Am. Chem. Soc., 2009, 131, 8798 CrossRef CAS PubMed;
(c) D. Steinhuebel, Y. Sun, K. Matsumura, N. Sayo and T. Saito, J. Am. Chem. Soc., 2009, 131, 11316 CrossRef CAS PubMed.
- C. K. Savile, J. M. Janey, E. C. Mundorff, J. C. Moore, S. Tam, W. R. Jarvis, J. C. Colbeck, A. Krebber, F. J. Fleitz, J. Brands, P. N. Devine, G. W. Huisman and G. J. Hughes, Science, 2010, 329, 305 CrossRef CAS PubMed.
- A. A. Desai, Angew. Chem., Int. Ed., 2011, 50, 1974 CrossRef CAS PubMed.
-
(a) K.-E. Andersson and G. Wagner, Physiol. Rev., 1995, 75, 191 CAS;
(b) M. Boolell, M. J. Allen, S. A. Ballard, S. Gepi-Attee, G. J. Muirhead, A. M. Naylor, I. H. Osterloh and J. C. Gingell, Int. J. Impotence Res., 1996, 8, 47 CAS;
(c) A. S. Bell, D. Brown and N. K. Terrett, EP-0463
756, 1991;
(d) D. J. Dale, J. Draper, P. J. Dunn, M. L. Hughes, F. Hussain, P. C. Levett, G. B. Ward and A. S. Wood, Org. Process Res. Dev., 2002, 6, 767 CrossRef CAS;
(e) D. J. Dale, P. J. Dunn, C. Golightly, M. L. Hughes, P. C. Levett, A. K. Pearce, P. M. Searle, G. B. Ward and A. S. Wood, Org. Process Res. Dev., 2000, 4, 17 CrossRef CAS;
(f) P. J. Dunn, in Process Chemistry in the Pharmaceutical Industry, ed. K. G. Gadamasetti and T. Braish, CRC Press, Boca Raton, FL, 2008, p. 267 Search PubMed.
- P. J. Dunn, S. Galvin and K. Hettenbach, Green Chem., 2004, 6, 43 RSC.
- B. A. Lauria-Horner and R. B. Pohl, Expert Opin. Invest. Drugs, 2003, 12, 663 CrossRef CAS PubMed.
-
(a) I. Selak, Pregabalin (Pfizer), Curr. Opin. Invest. Drugs, 2001, 2, 828 CAS;
(b) M. S. Hoekstra, D. M. Sobieray, M. A. Schwindt, T. A. Mulhern, T. M. Grote and B. K. Huckabee, Org. Process Res. Dev., 1997, 1, 26 CrossRef CAS.
- R. A. Jennings, W. S. Kissel, T. V. Le, I. C. Lennon, T. A. Mulhern, J. A. Ramsden and R. A. Wade, J. Org. Chem., 2003, 68, 5731 CrossRef PubMed.
- Z. Xie, J. Feng, E. Garcia, M. Bernett, D. Yazbeck and J. Tao, J. Mol. Catal. B: Enzym., 2006, 41, 5 CrossRef PubMed.
- F. Fulluga, G. Pitacco, E. Valentin and C. D. Venneri, Tetrahedron: Asymmetry, 2008, 19, 945 CrossRef PubMed.
- C. A. Martinez, S. Hu, Y. Dumond, J. Tao, P. Kelleher and L. Tully, Org. Process Res. Dev., 2008, 12, 392 CrossRef CAS.
- J. Verweij and E. de Vroom, Recl. Trav. Chim. Pays-Bas, 1993, 112, 66 CrossRef CAS.
- A. Bruggink, E. C. Roos and E. de Vroom, Org. Process Res. Dev., 1998, 2, 128 CrossRef CAS.
- N. Ran, L. Zhao, Z. Chen and J. Tao, Green Chem., 2008, 10, 361 RSC.
-
(a) The American Society of Health-System Pharmacists, “Simvastatin”, Drugs.com, retrieved 3 April 2011;
(b) A. W. Alberts, J. Chen, G. Kuron, V. Hunt, J. Huff, C. Hoffman, J. Rothrock, M. Lopez, H. Joshua, E. Harris, A. Patchett, R. Monaghan, S. Currie, E. Stapley, G. Albers-Schonberg, O. Hensens, J. Hirshfield, K. Hoogsteen, J. Liesch and J. Springer, Proc. Natl. Acad. Sci. U. S. A., 1980, 77, 3957 CrossRef CAS;
(c) A. Endo, J. Antibiot., 1980, 33, 334 CrossRef CAS;
(d) L. Hendrickson, C. R. Davis, C. Roach, D. K. Nguyen, T. Aldrich, P. C. McAda and C. D. Reeves, Chem. Biol., 1999, 6, 429 CrossRef CAS;
(e) J. Kennedy, K. Auclair, S. G. Kendrew, C. Park, J. C. Vederas and C. R. Hutchinson, Science, 1999, 284, 1368 CrossRef CAS.
- W. F. Hoffman, A. W. Alberts, P. S. Anderson, J. S. Chen, R. L. Smith and A. K. Willard, J. Med. Chem., 1986, 29, 849 CrossRef CAS.
- D. Askin, T. R. Verhoeven, T. M. Liu and L. Shinkai, J. Org. Chem., 1991, 56, 4929 CrossRef CAS.
- X. Xie and Y. Tang, Appl. Environ. Microbiol., 2007, 73, 2054 CrossRef CAS PubMed.
-
(a) D. J. Augeri, J. A. Robl, D. A. Betebenner, D. R. Magnin, A. Khanna, J. G. Robertson, A. Wang, L. M. Simpkins, P. Taunk, Q. Huang, S.-P. Han, B. Abboa-Offei, M. Cap, L. Xin, L. Tao, E. Tozzo, G. E. Welzel, D. M. Egan, J. Marcinkeviciene, S. Y. Chang, S. A. Biller, M. S. Kirby, R. A. Parker and L. G. Hamann, J. Med. Chem., 2005, 48, 5025 CrossRef CAS PubMed;
(b) “Bristol, Takeda Drugs Offer Alternatives to Januvia (Update2)”, Bloomberg, 2008-06-07.
- R. L. Hanson, S. L. Goldberg, D. B. Brzozowski, T. P. Tully, D. Cazzulino, W. L. Parker, O. K. Lyngberg, T. C. Vu, M. K. Wong and R. N. Patel, Adv. Synth. Catal., 2007, 349, 1369 CrossRef CAS.
-
(a) G. MacQueen, L. Born and M. Steiner, CNS Drug Rev., 2001, 7, 1 CrossRef CAS PubMed;
(b) A. L. McRae and K. T. Brady, Expert Opin. Pharmacother., 2001, 2, 883 CrossRef CAS PubMed;
(c) W. M. Welch, A. R. Kraska, R. Sarges and B. K. Koe, J. Med. Chem., 1984, 27, 1508 CrossRef CAS;
(d) G. P. Taber, D. M. Pfisterer and J. C. Colberg, Org. Process Res. Dev., 2004, 8, 385 CrossRef CAS.
- R. Capdeville, E. Buchdunger, J. Zimmermann and A. Matter, Nat. Rev. Drug Discovery, 2002, 1, 493 CrossRef CAS PubMed.
- A. Arora and E. M. Scholar, J. Pharmacol. Exp. Ther., 2005, 315, 971 CrossRef CAS PubMed.
- I. Melnikova and J. Golden, Nat. Rev. Drug Discovery, 2004, 3, 993 CrossRef CAS PubMed.
- A. Hochhaus and T. Hughes, Hematol. Oncol. Clin. North America, 2004, 18, 641 CrossRef PubMed.
-
(a) J. Zimmermann, CAS no. 120:107056, EP Patent 564,409, 1993;
(b) J. Zimmermann, CAS no. 125:4681, U.S. Patent 5,521,184, 1996.
- J. Zimmermann, E. Buchdunger, H. Mett, T. Meyer, N. B. Lydon and P. Traxler, Bioorg. Med. Chem. Lett., 1996, 6, 1221 CrossRef CAS.
- A. Kompella, B. R. K. Adibhatla, P. R. Muddasani, S. Rachakonda, V. K. Gampa and P. K. Dubey, Org. Process Res. Dev., 2012, 16, 1794 CrossRef CAS.
- S. Bhattacharyya, L. Fan, L. Vo and J. Labadie, Comb. Chem. High Throughput Screening, 2000, 3, 117 CrossRef CAS.
- M. C. Wani, H. L. Taylor, M. E. Wall, P. Coggon and A. T. McPhail, J. Am. Chem. Soc., 1971, 93, 2325 CrossRef CAS.
- F. A. Holmes, R. S. Walters, R. L. Theriault, A. D. Forman, L. K. Newton, M. N. Raber, A. U. Buzdar, D. K. Frye and G. N. Hortobagyi, J. Natl. Cancer Inst., 1991, 83, 1797 CrossRef CAS PubMed.
- M. Markman, Yale J. Biol. Med., 1991, 64, 583 CAS.
- F. L. Sung, T. C. W. Poon, E. P. Hui, B. B. Y. Ma, E. Liong, K. F. To, D. P. W. S. Huang and A. T. C. Chan, In Vivo, 2005, 19, 237 CAS.
- Z. Xie, H. L. Guan, X. Chen, C. Lu and L. Chen, J. Controlled Release, 2007, 117, 210 CrossRef CAS PubMed.
- M. Sufness and M. E. Wall, in Taxol: Science and Applications, ed. M. Sufness, CRC Press, Boca Raton, FL, 1995, p. 3 Search PubMed.
-
(a) R. A. Holton, US Pat., 5015744, 1991 and 5136060, 1992;
(b) A. K. Singh, R. E. Weaver, G. L. Powers, V. W. Rosso, C. Wei, D. A. Lust, A. S. Kotnis, E. T. Comoezoglu, M. Liu, K. S. Bembenek, B. D. Phan, D. J. Vanyo, M. L. Davies, R. J. Mathew, V. A. Palaniswamy, W.-S. Li, K. Gadamasetti, C. J. Spagnuolo and W. J. Winter, Org. Process Res. Dev., 2003, 7, 25 CrossRef CAS.
- J. C. Walker, Abstracts of Papers, 37th Middle Atlantic Regional Meeting of the American Chemical Society, New Brunswick, NJ, 2005, GENE-616.
-
(a) R. N. Patel, Annu. Rev. Microbiol., 1998, 98, 361 CrossRef PubMed;
(b) S. Jennewein and R. Croteau, Appl. Microbiol. Biotechnol., 2001, 57, 13 CrossRef CAS.
-
(a) Y. Yukimune, H. Tabata, Y. Higashi and Y. Hara, Nat. Biotechnol., 1996, 14, 1129 CrossRef CAS PubMed;
(b) M. Seki, C. Ohzora, M. Takeda and S. Furusaki, Biotechnol. Bioeng., 1997, 53, 214 CrossRef CAS;
(c) J.-J. Zhong, J. Biosci. Bioeng., 2002, 94, 591 CrossRef CAS.
- http://www.epa.gov/greenchemistry/pubs/docs/award_recipients_1996_2004.pdf,6–7.
- E. De Clercq, Nat. Rev. Drug Discovery, 2006, 5, 1015 CrossRef CAS PubMed.
- S. Abrecht, M. C. Federspiel, H. Estermann, R. Fischer, M. Karpf, H.-J. Mair, T. Oberhauser, G. Rimmler, R. Trussardi and U. Zutter, Chimia, 2007, 61, 93 CrossRef CAS.
- L. Wang and C. L. Weller, Trends Food Sci. Technol., 2006, 17, 300 CrossRef CAS PubMed.
- R. Carr, F. Ciccone, R. Gabel, M. Guinn, D. Johnston, J. Mastriona, T. Vandermeer and M. Groaning, Green Chem., 2008, 10, 743 RSC.
- R. Gabel, M. D. Groaning and D. A. Johnston, WO 2007/074091, July 5, 2007.
- A. K. Ressmann, P. Gaertner and K. Bica, Green Chem., 2011, 13, 1442 RSC.
-
(a) P. A. Gurbel, C. M. O'Connor, C. C. Cummings and V. L. Serebruany, Pharmacol. Res., 1999, 40, 107 CrossRef CAS PubMed;
(b) L. H. Yang, D. Hoppensteadt and J. Fareed, Thromb. Res., 1998, 92, 83 CrossRef CAS.
-
(a) A. Bousquet, S. Calet and A. Heymes, 1992;
(b) A. Bousquet, and A. Musolino, 2003;
(c) M. Bouisset and J. Radisson, 1991.
-
(a) B. Castro, J. Dormoy and A. Previero, 2000;
(b) M. Descamps and J. Radisson, 1993.
- L. Wang, J. Shen, Y. Tang, Y. Chen, W. Wang, Z. Cai and Z. Du, Org. Process Res. Dev., 2007, 11, 487 CrossRef CAS.
- J. V. Duncia, A. T. Chiu, D. J. Carini, G. B. Gregory, A. L. Johnson, W. A. Price, G. J. Wells, P. C. Wong, J. C. Calabrese and P. B. M. W. M. Timmermans, J. Med. Chem., 1990, 33, 1312 CrossRef CAS.
- M. J. Wyvratt and A. A. Patchett, Med. Res. Rev., 1985, 5, 483 CrossRef CAS.
- P. Bühlmayer, P. Furet, L. Criscione, M. de Gasparo, S. Whitebread, T. Schmidlin, R. Lattmann and J. Wood, Bioorg. Med. Chem. Lett., 1994, 4, 29 CrossRef.
- N. Miyaura and A. Suzuki, Chem. Rev., 1995, 95, 2457 CrossRef CAS.
-
(a) A. F. Littke and G. C. Fu, Angew. Chem., Int. Ed., 2002, 41, 4176 CrossRef CAS;
(b) J. Kristensen, M. Lysen, P. Vedsø and M. Begtrup, Org. Lett., 2001, 3, 1435 CrossRef CAS PubMed.
- U. Beutler, M. Boehm, P. C. Fuenfschilling, T. Heinz, J.-P. Mutz, U. Onken, M. Mueller and W. Zaugg, Org. Process Res. Dev., 2007, 11, 892 CrossRef CAS.
- L. J. Goossen and B. Melzer, J. Org. Chem., 2007, 72, 7473 CrossRef CAS PubMed.
- S. Ghosh, A. S. Kumar and G. N. Mehta, Beilstein J. Org. Chem., 2010, 6, 27 Search PubMed.
- V. Pandarus, D. Desplantier-Giscard, G. Gingras, F. Béland, R. Ciriminna and M. Pagliaro, Org. Process Res. Dev., 2013, 17, 1492 CrossRef CAS.
|
This journal is © The Royal Society of Chemistry 2015 |