DOI:
10.1039/C5RA00239G
(Paper)
RSC Adv., 2015,
5, 23855-23864
Mononuclear manganese(III) complexes of bidentate NO donor Schiff base ligands: synthesis, structural characterization, magnetic and catecholase studies†
Received
11th January 2015
, Accepted 23rd February 2015
First published on 23rd February 2015
Abstract
We have synthesized four mononuclear manganese(III) complexes (1–4) of four closely related bidentate NO donor Schiff-base ligands, out of which three (2–4) were structurally characterized. Crystal structure determination reveals that all these complexes are in octahedral geometries. Magnetic studies have been carried out on complexes 2, 3 and 4 in the temperature range 2–300 K under a magnetic field of 0.1 T which yielded negative ZFS parameters of −2.96, –3.51 and −3.72 cm−1 respectively. The catecholase activities of complexes 1–4 have been investigated following the oxidation of 3,5-di-tert-butylcatechol (3,5-DTBC) to 3,5-di-tert-butylbenzoquinone (3,5-DTBQ) with molecular oxygen in DCM at 25 °C, which were found to follow the Michalis–Menton type relation giving the highest TON (Kcat) for the so far reported Mn(III) complexes.
Introduction
It is now well established that a number of Mn-containing biomolecules are found to contain manganese as mononuclear (superoxide dismutase1 and manganese dioxygenase2), dinuclear (catalases,3–7 ribonucleotide reductase,8 arginase9) and also as tetranuclear (oxygen evolving complex10) species. A significant advancement has been achieved in the biomimetic structural and functional modelling of manganese containing enzymes by synthetic chemists and biochemists, which have added a wealth of knowledge to our understanding of various aspects of manganese cluster chemistry with respect to structural, electrochemical and magnetic properties. In addition, these structural or functional models are also important in catalyzing a number of organic reactions.11–15 Though structural and functional models of manganese containing metalloenzymes have been extensively explored16,17 this area is still under continuous development.18,19
Catechol oxidase (CO) is a member of the type-III copper proteins20 which catalyzes exclusively the oxidation of catechols to the corresponding quinones, highly reactive intermediates that undergo auto-polymerization to produce melanin, a brown pigment responsible for protecting damaged tissues against pathogens and insects of the higher plants. Although CO is believed to be a copper containing protein and many dicopper complexes are found to act as successful models for such metalloenzymes; there are only few reports where manganese21–36 complexes were found to display such activities as well, despite the fact that no such native enzymes having metal ions other than copper is known. In these complexes tetradentate tripodal ligands, several pyridine derivatives, azametallacrown and compartmental Schiff base ligands have been utilized but there is no report on the mononuclear octahedral Mn(III) complexes of bidentate Schiff base ligands that display catecholase activities. Therefore, design and synthesis of functional model of CO containing metal ions other than copper, say manganese, seems to be interesting and at the same time challenging.
High-spin manganese(III) complexes with 5D ground term splits in octahedral crystal fields into 5T2g, and 5Eg, terms. Non-cubic symmetry and/or Jahn–Teller distortions are responsible to lift the orbital degeneracy of the 5Eg, ground term to orbital singlet lowest, either 5A1g or 5B1g, (in D4h symmetry). The spin degeneracy of the ground state is further lifted by spin–orbit coupling to give the so-called zero-field splitting (ZFS).
The exact nature of the ground state depends critically on the symmetry of the ligand field and the nature of metal ion. If the dx2−y2 orbital is unoccupied, then the complex is expected to be axially elongated, with the 5B1g level lying lowest. Such a ground term is expected37 to have principal susceptibilities in the order K‖ > K⊥, and hence D will be negative. Conversely, compression of the octahedron results in the 5A1g, lying lowest with positive D.
The zero-field splitting in Mn(III) is typically of the order of a few wavenumbers, and so the magnetic properties are expected to be Curie-like and close to spin only value (4.90μB), except at very low temperatures where a rapid decrease in μMn occurs. In fact, a number of studies on hexacoordinated Mn(III) Schiff-base complexes showed that these complexes displayed Curie-like behaviour in the temperature range 80–300 K with magnetic moments of about 4.90μB.38–40 Owing to small size of the ZFS, together with the possibilities of weak magnetic exchange, exact interpretation of the data was not always possible, and the possibility of relatively large errors in the magnitude of such interactions existed. More recently, a number of structural studies on Mn(III) Schiff-base complexes have been reported,41 mostly showing the presence of quite appreciable distortions about the Mn(III) center. Therefore, axially elongated configuration attracted considerable attention in the search for new Mn(III) SIMs with negative D values. In the present paper we are going to describe the catecholase activities and magnetic properties of some monomeric octahedral Schiff-base complexes of the type [Mn(NO)3].
Experimental section
Materials and reagents
The starting materials for the synthesis of the ligand H2Li (i = 1, 2, 3, and 4) like 5-bromo-salicylaldehyde, hydroxyl-amine hydrochloride, Na2SO4, tetrabutylammonium hydroxide, sodium azide, Mn(ClO4)2·6H2O are of reagent grade and used as received. Solvents like methanol, diethyl ether, dichloromethane (DCM), acetonitrile were of reagent grade and dried before use.
Physical measurements
Elemental analyses were carried out using a Perkin-Elmer 240 elemental analyzer. Infrared spectra (400–4000 cm−1) were recorded from KBr pellets on a Nicolet Magna IR 750 series-II FTIR spectrophotometers. 1H-NMR spectra were recorded in CDCl3 on a Bruker 300 MHz NMR Spectrophotometer using tetramethylsilane (δ = 0) as an internal standard. UV-vis spectra and kinetic studies were performed on Agilent 8453 UV-vis spectrophotometer. TOF-MS+ spectra were generated on a waters HRMS instrument (model: XEVO G2QTOF).
Crystallography
Single crystal X-ray data of 2–4 were collected at room temperature on a Bruker SMART APEX-II CCD diffractometers using graphite monochromated MoKα radiation (λ = 0.71073 Å). Data integration and reductions were processed with SAINT+ software.42 Structures were solved by the direct method and then refined on F2 by the full matrix least square technique with SHELX-97 software.43 The crystallographic data for 2–4 are given in Table 1.
Table 1 Crystal data and details of the structure determination
Formula |
C42H33Br3MnN3O3 |
C90H79Br6Mn2N6O6 |
C42H30Br3Cl3MnN3O3 |
Formula weight |
922.35 |
1929.87 |
1025.68 |
Crystal system |
Triclinic |
Triclinic |
Triclinic |
Space group |
P (no. 2) |
P (no. 2) |
P (no.2) |
a, b, c [Å] |
8.246(2) 12.765(4) 18.256(6) |
11.5135(4) 14.5392(6) 25.8603(10) |
8.8296(15) 12.345(2) 20.440(4) |
α, β, γ [°] |
70.607(9) 84.283(9) 84.456(8) |
103.534(3) 90.011(3) 90.013(3) |
76.581(5) 85.576(6) 70.170(5) |
V [Å3] |
1799.5(9) |
4208.7(3) |
2038.7(6) |
Z |
2 |
2 |
2 |
D(calc) [g cm−3] |
1.702 |
1.523 |
1.671 |
μ(MoKα) [mm−1] |
3.743 |
3.205 |
3.503 |
F(000) |
920 |
1938 |
1016 |
Crystal size [mm] |
0.08 × 0.10 × 0.12 |
0.09 × 0.15 × 0.23 |
0.10 × 0.12 × 0.20 |
Temperature (K) |
120 |
296 |
120 |
Radiation [Å MoKα] |
0.71073 |
0.71073 |
0.71073 |
θmin, θmax [°] |
1.7, 25.7 |
0.8, 27.5 |
1.8, 24.8 |
Dataset |
−6: 10; −14: 14; −21: 20 |
−14: 14; −18: 18; −33: 33 |
−6: 10; −14: 14; −24: 24 |
Tot., Uniq. Data, R(int) |
11577, 6805, 0.030 |
64441, 19368, 0.154 |
13165, 6902, 0.032 |
Observed data [I > 2.0σ(I)] |
4184 |
5262 |
4265 |
Nref, Npar |
6805, 469 |
19368, 997 |
6902, 496 |
R, wR2, S |
0.0390, 0.1275, 0.98 |
0.0804, 0.1650, 0.992 |
0.0689, 0.1786, 1.01 |
Magnetic Measurements
Magnetic measurements were performed using a Quantum Design SVSM (Squid-Vibrating Sample Magnetometer) magnetometer. The static susceptibility measurement were performed in 300–1.8 K temperature range with an applied field of 5 kOe. Measurement of magnetization at different fields and at a given temperature confirm the absence of ferromagnetic impurities. Data were corrected for the sample holder and diamagnetism was estimated from Pascal constants.
Syntheses and characterization
Preparation of Schiff base ligands. All four ligands (HL1–HL4) were synthesized following the same procedure as described below in details for HL1, except using the appropriate aldehyde and amine (Scheme 1).
 |
| Scheme 1 Schematic presentation of preparation of complexes 1–4. | |
Synthesis of ligand HL1. 0.136 g (1 mmol) of 2-hydroxy-5-methyl benzaldehyde was dissolved in 20 mL ethanol to which 0.110 g (1 mmol) of benzylamine was added drop wise and the solution was refluxed for 3–4 hours. It was then cooled and solvent was removed under reduced pressure to get solid product which was recrystallized from MeOH to get pure product. This protocol was adopted for the synthesis of other related ligands viz. HL2, HL3 and HL4.
Analyses.
HL1. 1H-NMR: δ in ppm 2.247 (3H, s, –CH3), 4.73 (2H, s, –CH2), 6.7–7.3 (8H, m, aromatic protons), 8.45 (1H, s, –N
CH), 13.41 (1H, s, –OH). 13C-NMR: δ in ppm 18.96 (s, Me Carbon), 62.20 (s, C7), 116.36–159.41 (m, aromatic carbons), 165.94 (s, C8). Elemental analysis: Calculated, C, 80.00; H, 6.67; N, 6.22; found, C, 81.20; H, 6.33; N, 6.41.
HL2. 1H-NMR: δ in ppm 4.82 (2H, s, –CH2), 6.85–7.40 (8H, m, aromatic protons), 8.35 (1H, s, –N
CH), 13.42 (1H, s, –OH). 13C-NMR: δ in ppm 62.99 (s, C7), 109.94–160.11 (m, aromatic carbons), 164.20 (s, C8). Elemental analysis: calculated, C, 57.93; H, 4.14; N, 4.83; found, C, 58.32; H, 4.39; N, 5.08.
HL3. 1H-NMR: δ in ppm 2.36 (3H, s, –CH3), 4.77 (2H, s, –CH2), 6.85–7.40 (7H, m, aromatic protons), 8.31(1H, s, –N
CH), 13.49 (1H, s, –OH). 13C-NMR: δ in ppm 21.13 (s, Me Carbon), 62.77 (s, C7), 109.99–160.29 (m, aromatic carbons), 164.07 (s, C8). Elemental analysis: calculated, C, 59.21; H, 4.61; N, 4.61; found, C, 60.02; H, 4.35; N, 5.25.
HL4. 1H-NMR: δ in ppm 4.78 (2H, s, –CH2), 6.87–7.43 (7H, m, aromatic protons), 8.36 (1H, s, –N
CH), 13.25 (1H, s, –OH). 13C-NMR: δ in ppm 62.34 (s, C7), 110.08–159.99 (m, aromatic carbons), 164.54 (s, C8). Elemental analysis: calculated, C, 51.77; H, 3.39; N, 4.31; found, C, 52.52; H, 3.63; N, 5.02.
Preparation of complexes 1–4. All complexes were synthesized following the same procedure as described below in details for complex 1. Bright amorphous solid product was obtained for 1 while shiny single crystals, suitable for single crystal X-ray diffraction were obtained for complexes 2–4.
Preparation of [Mn(L1)3] (1). 3.0 mmol (0.87 g) ligand (HL1) was dissolved in minimum volume of acetonitrile. To this solution an equimolar amount of (0.303 g) TEA (triethylamine) was added followed by 1 mmol (0.254 g) Mn(ClO4)2·6H2O. It was then refluxed for 1 hour. The resulting greenish brown solution was cooled to room temperature, filtered and kept in rack where upon dark brown amorphous solid product was obtained within 2–3 days. Yield: 0.472 g (65%).
Elemental analyses.
[MnIII(L1)3] (1). C45H42MnN3O3 (MW = 727.77); ESI-MS (+ve): M + H+ = 728.81, elemental analysis: calculated, C, 74.27; H, 5.82; N, 5.77; found, C, 75.10; H, 5.45; N, 5.12.
[MnIII(L2)3] (2). Color: dark brown, C42H33Br3MnN3O3 (MW = 922.35); yield: 0.599 g (65%), calculated, C, 54.68; H, 3.60; N, 4.56; found, C, 55.38; H, 3.85; N, 4.95.
[MnIII(L3)3] (3). Color: dark green, C45H39Br3MnN3O3 (per unit cell) (MW = 964.43); yield: 0.655 g (68%), calculated, C, 56.04; H, 4.08; N, 4.36; found, C, 56.54; H, 4.39; N, 4.77.
[MnIII(L4)3] (4). Color: dark brown, C42H30Br3Cl3MnN3O3 (MW = 1025.68); yield: 0.717 g (70%), calculated, C, 49.18; H, 2.95; N, 4.10; found, C, 50.11; H, 3.15; N, 4.32.
Kinetic studies. All kinetic experiments were carried out under pseudo-first-order conditions, with the Mn(III) complexes as the minor component. All measurements were performed in an Agilent diode-array UV-vis spectrophotometer. Detail kinetic procedures involve the preparation of stock solutions of complexes (1.0 × 10−3 M) and the substrate, 3,5-DTBC, at higher concentrations in pure DCM. From this stock solutions a set of 12 solutions of [3,5-DTBC] = 0.0001–0.01 M were prepared. A 2 ml portion of each solution was pipetted out into a quartz cell and equilibrated for 15 min at 25 °C by inserting into the shell holder which is attached to a Peltier temperature controller system. Now 20 μL of stock solution of the complex was added to it to achieve the ultimate concentration of the complex 1.0 × 10−5 M.
Results and discussion
Synthesis and structural descriptions of complexes 2–4
Bidentate NO donor ligands HLi (i = 1–4) were synthesized by the simple Schiff base condensation between appropriate aldehydes and amines in EtOH under refluxing conditions. All the four Mn(III) complexes were synthesized by the reaction between Mn(ClO4)2·6H2O and appropriate ligand in MeCN at ambient temperature which afford single crystals suitable for X-ray diffraction studies with the exception of complex 1. Several trials to get single crystals of 1 were not successful.
The single crystal X-ray diffraction studies showed that complexes 2–4, depicted in Fig. 1–3, are crystallized in triclinic system with space group P
. All these complexes are monomeric in nature surrounded by three bidentate NO donor Schiff base ligands. The central manganese atom in each complex is in +3 oxidation state. In case of complexes 2 and 4 there are only one complex unit in the unit cell and the Mn atoms are coordinated by N1, O1, N2, O2 and N3, O3 atom from three ligands while in complex 3 two complex species (A and B) are present in the unit cell. In A the central manganese(III) ion (Mn1) are coordinated by N1, O1, N2, O2 and N3, O3 and Mn2 atom in B is coordinated by N4, O4, N5, O5 and N6, O6, which differ only in bond angles and bond lengths. Mn–Oi distances fall in the range 1.858–1.930 Å for 2, 1.877–1.908 Å (A) and 1.881–1.929 Å (B) for 3, whereas 1.862–1.933 Å for 4. The Mn–Ni bond distances are: 2.063–2.30 Å for 2, 2.091–2.277 Å (A) and 2.087–2.252 Å (B) for 3 whereas 2.082–2.310 Å for 4. Some selected bond distances and angles for complexes 2–4 are summarized in Table 2. The non-covalent interactions e.g. hydrogen bonding, π⋯π and C–H⋯π stacking have been taken into account to clarify the possible supramolecular topologies.
 |
| Fig. 1 The molecular view of complex 2. All H-atoms are omitted for clarity. | |
 |
| Fig. 2 The molecular view of complex 3. All H-atoms are omitted for clarity. | |
 |
| Fig. 3 The molecular view of complex 4. All H-atoms are omitted for clarity. | |
Table 2 Selected bond distances and bond angles of complexes 2–4
2 |
3 |
4 |
Mn1–O1 |
1.858(3) |
Mn1–O2 |
1.909(8) |
Mn1–O1 |
1.866(5) |
Mn1–O2 |
1.931(3) |
Mn1–N2 |
2.277(10) |
Mn1–O2 |
1.934(6) |
Mn1–O3 |
1.879(3) |
Mn1–O3 |
1.877(6) |
Mn1–O3 |
1.862(5) |
Mn1–N1 |
2.300(3) |
Mn1–N1 |
2.091(10) |
Mn1–N1 |
2.281(7) |
Mn1–N2 |
2.234(3) |
Mn1–O1 |
1.905(6) |
Mn1–N2 |
2.082(7) |
Mn1–N3 |
2.063(3) |
Mn1–N3 |
2.244(8) |
Mn1–N3 |
2.310(7) |
|
|
Mn2–O5 |
1.893(6) |
|
|
|
|
Mn2–O6 |
1.881(6) |
|
|
|
|
Mn2–O4 |
1.930(8) |
|
|
|
|
Mn2–N5 |
2.253(10) |
|
|
|
|
Mn2–N6 |
2.245(10) |
|
|
|
|
Mn2–N4 |
2.087(10) |
|
|
![[thin space (1/6-em)]](https://www.rsc.org/images/entities/char_2009.gif) |
2 |
3 |
4 |
O1–Mn1–O2 |
90.63(12) |
O1–Mn1–O2 |
91.2(3) |
O1–Mn1–O2 |
88.1(2) |
O1–Mn1–O3 |
175.94(13) |
O1–Mn1–O3 |
178.5(3) |
O1–Mn1–O3 |
179.8(3) |
O1–Mn1–N1 |
87.57(13) |
O1–Mn1–N1 |
90.4(3) |
O1–Mn1–N1 |
94.3(2) |
O1–Mn1–N2 |
86.65(13) |
O1–Mn1–N2 |
91.4(3) |
O1–Mn1–N2 |
88.9(3) |
O1–Mn1–N3 |
90.03(14) |
O1–Mn1–N3 |
86.8(3) |
O1–Mn1–N3 |
95.6(3) |
O2–Mn1–O3 |
90.40(13) |
O2–Mn1–O3 |
89.7(3) |
O2–Mn1–O3 |
91.8(2) |
O2–Mn1–N1 |
82.65(12) |
O2–Mn1–N1 |
177.4(3) |
O2–Mn1–N1 |
92.2(3) |
O2–Mn1–N2 |
95.26(12) |
O2–Mn1–N2 |
83.6(3) |
O2–Mn1–N2 |
175.1(3) |
O2–Mn1–N3 |
170.65(13) |
O2–Mn1–N3 |
88.8(3) |
O2–Mn1–N3 |
83.1(3) |
O3–Mn1–N1 |
96.46(14) |
O3–Mn1–N1 |
88.8(3) |
O3–Mn1–N1 |
85.5(2) |
O3–Mn1–N2 |
89.35(14) |
O3–Mn1–N2 |
87.5(3) |
O3–Mn1–N2 |
91.2(3) |
O3–Mn1–N3 |
89.60(15) |
O3–Mn1–N3 |
94.5(3) |
O3–Mn1–N3 |
84.6(2) |
N1–Mn1–N2 |
173.83(15) |
N1–Mn1–N2 |
94.3(3) |
N1–Mn1–N2 |
91.9(3) |
N1–Mn1–N3 |
88.07(12) |
N1–Mn1–N3 |
93.4(3) |
N1–Mn1–N3 |
168.9(2) |
N2–Mn1–N3 |
94.09(13) |
N2–Mn1–N3 |
172.1(3) |
N2–Mn1–N3 |
93.3(2) |
|
|
O5–Mn2–N6 |
87.2(3) |
|
|
|
|
O6–Mn2–N4 |
88.5(3) |
|
|
|
|
O6–Mn2–N5 |
87.8(3) |
|
|
|
|
O6–Mn2–N6 |
93.5(3) |
|
|
|
|
N4–Mn2–N5 |
94.2(3) |
|
|
|
|
N4–Mn2–N6 |
93.7(3) |
|
|
|
|
N5–Mn2–N6 |
172.0(3) |
|
|
|
|
O4–Mn2–O5 |
91.0(3) |
|
|
|
|
O4–Mn2–O6 |
89.8(3) |
|
|
|
|
O4–Mn2–N4 |
177.4(3) |
|
|
|
|
O4–Mn2–N5 |
83.8(3) |
|
|
|
|
O4–Mn2–N6 |
88.4(3) |
|
|
|
|
O5–Mn2–O6 |
178.9(3) |
|
|
In complex 2 the supramolecular interactions between: (i) aromatic hydrogen and one phenoxido oxygen (green dotted) (ii) π(aromatic)⋯π(aromatic) (magenta dotted lines) and (iii) C–H⋯π (green dotted lines) lead to the formation of supramolecular 1D chains along the crystallographic c axis (Fig. 4) and these 1D chains are further extended to 2D network (Fig. S9†) via inter-locking Br⋯H interactions among themselves in the crystallographic ab plane. Similarly, complex 3 exhibits the same supramolecular topologies (Fig. 5 and S10†) via hydrogen bonding as well as C–H⋯π interactions in crystallographic bc plane. The hydrogen bonding (Br⋯H and Cl⋯H) and C–H⋯π interactions for complex 4 also play a crucial role for the formation of H-bonded supramolecular network (Fig. S11†).
 |
| Fig. 4 The supramolecular interactions: H-bonding (green dotted lines), CH⋯π(phenyl) (blue lines) and π(phenyl)⋯π(phenyl) (magenta lines) are combined to form 1D network along crystallographic c-axis in 2. All H-atoms except those involved in interactions are omitted for clarity. | |
 |
| Fig. 5 The supramolecular interactions: H-bonding (green dotted line), CH⋯π (phenyl) (blue dotted line) are combined to form 1D framework along crystallographic bc plane in 3. | |
Electronic spectra of complexes
The electronic spectra of all the four complexes were recorded in DCM, the details of which are incorporated in Table 3. Bands at ∼333 and 389 nm correspond to the LMCT transitions (Fig. 6).44
Table 3 Summary of electronic spectra of Mn(III) complexes in DCM
Complexes |
λmax (nm) |
ε (dm3 mol−1 cm−1) × 104 |
λmax (nm) |
ε (mol−1 dm3 cm−1) × 105 |
1 |
389 |
6.82 |
324 |
1.32 |
2 |
385 |
1.67 |
327 |
0.38 |
3 |
388 |
1.06 |
329 |
0.20 |
4 |
389 |
2.69 |
328 |
0.43 |
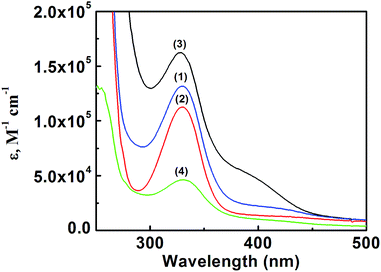 |
| Fig. 6 Electronic spectra of manganese(III) complexes 1–4 in DCM. | |
Catecholase activity
General features. The reaction of excess 3,5-ditertiarybutyl catechol (3,5-DTBC) with [Mn(Li)3] in DCM leads to the formation of 3,5-ditertiarybutyl quinone (3,5-DTBQ) as shown in eqn (1). Fig. 7 and S12, S13† show time resolved spectra of the reaction of Mn(III) complexes (1–4) with 3,5-DTBC. When the complexes, 1–4 (∼1.0 × 10−5 M) were treated with 3,5-DTBC in DCM, under aerobic conditions there was a gradual increase in absorbance at 403 nm, characteristics of the formation of 3,5-DTBQ. |
 | (1) |
 |
| Fig. 7 Time-resolved spectra for the reaction of 1 and 2 with 5-DTBC in DCM. | |
Kinetics. The formation of 3,5-DTBQ was monitored with time at a wavelength of ∼400 nm (Fig. 7). The observed rate constants (ki) were extracted by the initial rate method. Plots of ki vs. [3,5-DTBC] gave non-linear curve of decreasing slope (Fig. 8) which are best described by eqn (2). A reasonable rate law can be derived as described in eqn (3), where V = initial rate constant (ki); [S] = concentration of the substrate, 3,5-DTBC; KM = (k2 + k3)/k1, Michaelis–Menten constant for the metal complex and Vmax = maximum initial rate attained for a particular concentration of the metal complex in the presence of a large excess of the 3,5-DTBC. The liner form of the Michaelis–Menten equation is given in eqn (4), known as Lineweaver–Burk equation and corresponding fitting is shown in Fig. S14.† Non-linear fitting of data to Michaelis–Menten eqn (3) leads to the evaluation of Vmax, KM and Kcat = KM/[complex] and all these parameters are listed in Table 4. |
 | (2) |
|
 | (3) |
|
 | (4) |
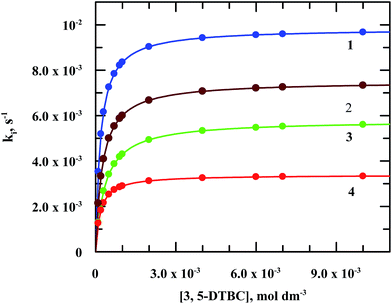 |
| Fig. 8 Plots of ki vs. [3,5-ditertiarybutyl catechol] for the reaction of 3,5-DTBC with molecular oxygen catalyzed by manganese(III) complexes. | |
Table 4 Summary of catecholase activity of 1–4 with 3,5-ditertiarybutyl catechol in DCM. Conditions: [c] = 1.0 × 10−5 M
Complex |
104 × KM |
103 × Vmax (M s−1) |
Kcat (s−1) |
1 |
1.79 ± 0.28 |
9.84 ± 3.27 |
17.9 |
2 |
3.48 ± 0.48 |
5.69 ± 0.22 |
34.8 |
3 |
1.70 ± 0.63 |
3.39 ± 0.27 |
17.0 |
4 |
2.51 ± 0.45 |
7.51 ± 0.33 |
25.1 |
It is interesting to note that there are only few examples of where manganese complexes were claimed to display CO activities.21,25,45–49 As for example, radical Mn(IV) complexes catalyzed the oxidation of 3,5-DTBC to 3,5-DTBQ in presence of molecular oxygen under mild conditions.44,45 Some mononuclear Mn(III) complexes also found to favour the oxidation of catechol to quinone where direct involvement of Mn(III) was suggested.45 Table 5 displays the catalytic activities of some mononuclear Mn(III) as well as some multinuclear complexes. It is worth mentioning that our complexes showed the highest TON (Kcat, h−1) for the catalytic oxidation of 3,5-DTBC to 3,5-DTBQ under mild conditions by molecular oxygen and the existence of 3,5-DTBQ was identified from ESI-MS+ (m/z) study (Fig. 9).
Table 5 Catalytic activity of synthetic catecholase mimic
Compound |
Kcat (h−1) |
104 × KM (M) |
Kcat/KM (s−1 M−1) |
Ref. |
1 |
6.44 × 104 |
1.79 |
9.84 |
This work |
2 |
1.25 × 105 |
3.48 |
5.69 |
This work |
3 |
6.12 × 104 |
1.70 |
3.39 |
This work |
4 |
9.04 × 104 |
2.51 |
7.51 |
This work |
[Mn(bpia)(OAc)(OCH3)](PF6) |
86 |
15.0 |
16 |
21 |
[Mn(bipa)(OAc)(OCH3)](PF6) |
101 |
12.0 |
23 |
21 |
[Mn(bpia)(Cl)2](ClO4) |
230 |
13.0 |
49 |
21 |
[Mn(bipa)(Cl)2](ClO4) |
130 |
8.0 |
45 |
21 |
[Mn(diclofenac)2(H2O)] |
225 |
|
|
46 |
[Mn(tpa)2](ClO4)2 |
4 |
|
|
47 |
[MnL1(OOCH)(OH2)] |
936.64 |
5.50 |
1.606 × 10−4 |
25 |
[MnL2(OH2)2][Mn2L22(NO2)3] |
365.34 |
6.41 |
6.089 × 10−5 |
25 |
[Mn2L21(NO2)2] |
1432.74 |
49.17 |
2.388 × 10−4 |
25 |
[Mn2IIIMn4IIO2(pyz)2(C6H5CH2COO)10]n |
2.547 × 103 |
1.75 |
7.076 × 10−5 |
48 |
Mn2L8Cl4·4H2O |
3.60 × 103 |
9.00 |
1.00 × 10−4 |
26 |
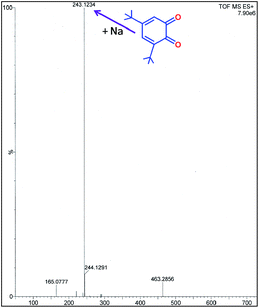 |
| Fig. 9 ESI-MS+ (m/z) spectrum for the ultimate catecholase product during the reaction of 2 with 3,5-DTBC. | |
Magnetic study. Magnetic measurements have been carried out on all three mononuclear crystalline complexes 2–4. Variable-temperature DC magnetic susceptibility (χM) of complexes 2–4 is investigated in the temperature range of 2–300 K under a magnetic field of 0.1 T. Fig. 10 shows the variation of susceptibility in the form of χMT vs. T. The observed χMT values (2.95 and 2.65 cm3 K mol−1) for complexes 2 and 3 at 300 K are close to the value obtained for uncoupled high spin S = 2 Mn(III) system (g = 2), while the same is quite low for complex 4 (1.21 cm3 K mol−1). Upon lowering the temperature for complexes 2 and 3, the χMT product remains almost constant between 300 to 25 K and then decreases sharply to lower temperature regime down to 2 K, signifying the presence of magnetic anisotropy (zero-field splitting). For complex 4, the χMT product starts increasing from 300 to 25 K and follows the same path at lower temperatures similar to complexes 2 and 3. This anomalous behavior is due to the presence of strong supra-molecular π⋯π stacking interaction (intermolecular distance: 3.890 Å) in complex 4 which is weak in complex 3 and almost absent in complex 2. This mediates the ferromagnetic exchange coupling (+0.16 cm−1) between the two Mn(III) ions of the adjacent mononuclear units in complex 4. The above susceptibility behavior is fitted using the following axial spin Hamiltonian (5). |
 | (5) |
where, the specific terms have their usual meaning.
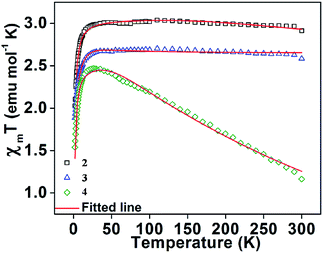 |
| Fig. 10 The variation of χMT (experimental points) with temperature for the three complexes 2, 3 and 4. Solid lines represent the theoretical curve and the points are the experimental data. | |
Weak intermolecular interaction (J) acting between two Mn(III) ions is incorporated in the expression (5) with zero field splitting (D) parameter to obtain the best fit parameters for g (Lande factor) and D (ZFS). The χMT data of the complexes were fitted assuming the same values of Lande g factors (g1 and g2) and the ZFS terms (D1 and D2) for the two exchange spin systems (S1 and S2). The magnetization data were fitted by taking the same g values obtained from the fitted plots of χMT vs. T (Fig. 11 and S15, S16†). This also leads to the same values of exchange and ZFS parameters, summarized in Table 6. In each case, the best fitted curve was obtained by the negative values of zero-field splitting (D) parameter.
 |
| Fig. 11 M vs. H plots collected at 2, 4 and 5 K for complex 2. Solid lines represent the theoretical curve and the points are the experimental data. | |
Table 6 Results of fitting of dc magnetic data and comparison with related systemsa
Complex |
D (cm−1) |
J (cm−1) |
g |
104 × R2 |
Estimated errors: D (= ±0.02), J (= ±0.01); g (= ±0.01). Ref. 49. Dbm = dibenzoylmethane. |
Mn(L2)3 (2) |
–2.96 |
–0.08 |
1.98 |
1.89 |
Mn(L3)3 (3) |
–3.51 |
–0.06 |
1.97 |
3.78 |
Mn(L4)3 (4) |
–3.72 |
+0.16 |
1.98 |
5.34 |
[Mn(dbm)3]b |
−4.52 |
|
2.03 |
1.30 |
[Mn(dbm)2(DMSO)2](ClO4)b |
−3.42 |
|
1.92 |
4.10 |
[Mn(dbm)2(py)2](ClO4)b |
−4.46 |
|
1.97 |
0.47 |
Table 6 displays ZFS values (D) for some recently reported octahedral Mn(III) complexes along with our results. The large negative D values are consistent with the Jahn–Teller axis elongation of the octahedral geometry.
Conclusion
We have synthesized four mononuclear Mn(III) complexes of bidentate Schiff base ligands out of which three (2–4) were characterized structurally and found to have octahedral geometries which showed excellent catechol oxidase activity with very high, probable the highest, turn over number (TON) of the so far reported Mn(III) complexes. Another interesting aspect of this study is that these complexes being in regular octahedral geometry and devoid of any labile ancillary ligand which encourages the coordination of catechol to the metal center also showed CO activity. Cryomagnetic studies on complexes 2–4 gives negative ZFS values, comparable to recently reported values.
Acknowledgements
Financial support from CSIR (ref. 02(2490)/11/EMR-II) and UGC [39-735/2010(SR)], New Delhi, are gratefully acknowledged.
References
- S. Mukhopadhyay, S. K. Mandal, S. Bhaduri and W. H. Armstrong, Chem. Rev., 2004, 104, 3981 CrossRef CAS PubMed.
- B. Zhou, R. Tao, S. Q. Shen and J. Q. Liang, Phys. Rev. A, 2002, 66, 01030 Search PubMed.
- D. Gatteschi and R. Sessoli, Angew. Chem., Int. Ed., 2003, 42, 268 CrossRef CAS PubMed.
- J. W. Whittaker, in Metal Ions in Biological Systems, ed. A. Sigel and H. Sigel, Marcel Dekker, New York, 2000, vol. 37, p. 587 Search PubMed.
- L. Que Jr and M. F. Reynolds, in Metal Ions in Biological Systems, ed. A. Sigel and H. Sigel, Marcel Dekker, New York, 2000, vol. 37, p. 505 Search PubMed.
-
(a) Y. Kono and I. Fridovich, J. Biol. Chem., 1983, 258, 6015 CAS;
(b) W. F. Beyer Jr and I. Fridovich, Biochemistry, 1985, 24, 6460 CrossRef CAS.
-
(a) V. V. Barynin, A. A. Vagin, W. R.Melik-Adamyan, A. I. Grebenko, S. V. Khangulov, A. N. Popov, M. E. Andrianova and B. K. Vainshtein, Dokl. Akad. Nauk, 1986, 288, 877 CAS;
(b) V. V. Barynin, P. D. Hempstead, A. A. Vagin, S. V. Antonyuk, W. R. M. Adamyan, V. S. Lamzin, P. M. Harrison and P. J. Artymyuk, J. Inorg. Biochem., 1997, 67, 196 CrossRef CAS.
- G. S. Allgood and J. J. Perry, J. Bacteriol., 1986, 168, 563 CAS.
- I. Michaud-Soret, L. Jacquamet, N. Debaecker-Petit, L. Le Pape, V. V. Barynin and J. M. Latour, Inorg. Chem., 1998, 37, 3874 CrossRef CAS.
- A. J. Wu, J. E. Penner-Hahn and V. L. Pecoraro, Chem. Rev., 2004, 104, 903 CrossRef CAS PubMed.
- G. Auling and H. Follmann, in Metal Ions in Biological Systems, ed. A. Sigel and H. Sigel, Marcel Dekker, New York, 1994, vol. 30, p. 131 Search PubMed.
- D. E. Ash, J. D. Cox and D. W. Christianson, in Metal Ions in Biological Systems, ed. A. Sigel and H. Sigel, Marcel Dekker, New York, 2000, vol. 37, p. 407 Search PubMed.
- M. U. Triller, W. Y. Hsieh, V. L. Pecoraro, A. Rompel and B. Krebs, Inorg. Chem., 2002, 41, 5544 CrossRef CAS PubMed.
- K. A. Jørgensen, Chem. Rev., 1989, 89, 431 CrossRef.
- M. J. Gunter and P. Turner, Coord. Chem. Rev., 1991, 108, 115 CrossRef CAS.
- T. Katsuki, Coord. Chem. Rev., 1995, 140, 189 CrossRef CAS.
- Catalytic Asymmetric Synthesis, ed. T. Katsuki and I. Ojima, Wiley, New York, Second edn, 2000, p. 287 Search PubMed.
- S. Majumder, S. Hazra, P. Biswas and S. Mohanta, Polyhedron, 2009, 28, 2473 CrossRef CAS PubMed.
- V. L. Pecoraro, M. J. Baldwin and A. Gelasco, Chem. Rev., 1994, 94, 807 CrossRef CAS.
- A. J. Wu, J. E. Penner-Hahn and V. L. Pecoraro, Chem. Rev., 2004, 104, 903 CrossRef CAS PubMed.
- M. U. Triller, D. Pursche, W. Y. Hsieh, V. L. Pecoraro, A. Rompel and B. Krebs, Inorg. Chem., 2003, 42, 6274 CrossRef CAS PubMed.
- S. Mukherjee, T. Weyhermuller, E. Bothe, K. Wieghardt and P. Chaudhuri, Dalton Trans., 2004, 3842 RSC.
- S. Mukherjee, E. Rentschler, T. Weyhermuller, K. Wieghardt and P. Chaudhuri, Chem. Commun., 2003, 1828 RSC.
- S. Mukherjee, E. Rentschler, T. Weyhermüller, K. Wieghardt and P. Chaudhuri, J. Chem. Soc., Chem. Commun., 2003, 1828 RSC.
- P. Seth, M. G. B. Drew and A. Ghosh, J. Mol. Catal. A: Chem., 2012, 365, 154–161 CrossRef CAS PubMed.
- K. S. Banu, T. Chattopadhyay, A. Banerjee, M. Mukherjee, S. Bhattacharya, G. K. Patra, E. Zangrando and D. Das, Dalton Trans., 2009, 8755–8764 RSC.
- P. Chakraborty, S. Majumder, A. Jana and S. Mohanta, Inorg. Chim. Acta, 2014, 410, 65–75 CrossRef CAS PubMed.
- A. Guha, K. S. Banu, A. Banerjee, T. Ghosh, S. Bhattacharya, E. Zangrando and D. Das, J. Mol. Catal. A: Chem., 2011, 338, 51 CAS.
- J. Kaizer, G. Barath, R. Csonka, G. Speier, L. Korecz, A. Rockenbauer and L. Párkányi, J. Inorg. Biochem., 2008, 102, 773 CrossRef CAS PubMed.
- J. Kaizer, R. Csonka, G. Barath and G. Speier, Transition Met. Chem., 2007, 32, 1047 CrossRef CAS.
- A. Majumder, S. Goswami, S. R. Batten, M. S. E. Fallah, J. Ribas and S. Mitra, Inorg. Chim. Acta, 2006, 359, 2375 CrossRef CAS PubMed.
- K. S. Banu, T. Chattopadhyay, A. Banerjee, M. Mukherjee, A. S. Bhattacharya, G. K. Patra, E. Zangrando and D. Das, Dalton Trans., 2009, 8755 RSC.
- S. Mukherjee, T. Weyhermuller, E. Bothe, K. Wieghardt and P. Chaudhuri, Dalton Trans., 2004, 3842 RSC.
- G. Blay, I. Fernandez, J. R. Pedro, R. Ruiz, T. T. Sanchez, E. Pardo, F. Lloret and M. C. Munoz, J. Mol. Catal. A: Chem., 2006, 250, 20 CrossRef CAS PubMed.
- A. Jana, N. Aliaga-Alcalde, E. Ruiz and S. Mohanta, Inorg. Chem., 2013, 52, 7732 CrossRef CAS PubMed.
- J. Kaizer, R. Csonka, G. Baráth and G. Speier, Transition Met. Chem., 2007, 32, 1047 CrossRef CAS.
- L. I. Simándi and T. L. Simándi, J. Chem. Soc., Dalton Trans., 1998, 3275 RSC.
- J. Krzystek, G.-J. Yeagle, J.-H. Park, R.-D. Britt, M.-W. Meisel, L.-C. Brunel and J. Telser, Inorg. Chem., 2003, 42, 4610 CrossRef CAS PubMed.
- J. Krzystek, A. Ozarowski and J. Telser, Coord. Chem. Rev., 2006, 250, 2308 CrossRef CAS PubMed.
- J. Vallejo, A. Pascual-Álvarez, J. Cano, I. Castro, M. Julve, F. Lloret, J. Krzystek, G. D. Munno, D. Armentano, W. Wernsdorfer, R. Ruiz-García and E. Pardo, Angew. Chem., Int. Ed., 2013, 52, 14075 CrossRef CAS PubMed.
- B. J. Kennedy and K. S. Murray, Inorg. Chem., 1985, 24, 1552 CrossRef CAS and the references therein.
- A. Altomare, M. C. Burla, M. Camalli, G. L. Cascarano, C. Giacovazzo, A. Guagliardi, A. G. G. Moliterni, G. Polidori and R. Spagna, J. Appl. Crystallogr., 1999, 32, 115 CrossRef CAS.
- G. M. Sheldrick, SHELX97 – Programs for Crystal Structure Analysis (Release 97-2), Institüt für Anorganische Chemie der Universität, Tamman strasse 4, D-3400 Göttingen, Germany, 1998 Search PubMed.
- A. Westphal, A. Klinkebiel, H.-M. Berends, H. Broda, P. Kurz and F. Tuczek, Inorg. Chem., 2013, 52(2372), 44 Search PubMed; E. I. Solomon, U. M. Sundaram and T. E. Machonkin, Chem. Rev., 1996, 96, 2563 CrossRef CAS PubMed.
- N. Kitajima and Y. Morooka, Chem. Rev., 1994, 94, 737 CrossRef CAS.
- D. Kovala-Demertzi, S. K. Hadjikakou, M. A. Demertzis and Y. Deligiannakis, J. Inorg. Biochem., 1998, 69, 223 CrossRef CAS.
- Y. Gultneh, A. Farooq, K. D. Karlin, S. Liu and J. Zubieta, Inorg. Chim. Acta, 1993, 211, 171 CrossRef CAS.
- P. Kar, R. Haldar, C. J. Gómez-García and A. Ghosh, Inorg. Chem., 2012, 51, 4265 CrossRef CAS PubMed.
- L. Chen, J. Wang, Y.-Z. Liu, Y. Song, X.-T. Chen, Y.-Q. Zhang and Z.-L. Xue, Eur. J. Inorg. Chem., 2015, 271, DOI:10.1002/ejic.201402964.
Footnote |
† Electronic supplementary information (ESI) available. CCDC [1041929(2), 1041930(3), 1041931(4)]. For ESI and crystallographic data in CIF or other electronic format see DOI: 10.1039/c5ra00239g |
|
This journal is © The Royal Society of Chemistry 2015 |