DOI:
10.1039/C4RA16913A
(Paper)
RSC Adv., 2015,
5, 19512-19519
Complex from ionic β-cyclodextrin polyrotaxane and sodium tetraphenylthiophenesulfonate: restricted molecular rotation and aggregation-enhanced emission†
Received
23rd December 2014
, Accepted 3rd February 2015
First published on 3rd February 2015
Abstract
Because restricted intramolecular rotation (RIR) is the main mechanism responsible for the aggregation-induced or -enhanced emission (AIE or AEE), we thus used a rigid polyrotaxane to impose effective RIR for an ionic, water-soluble and AEE-active luminogen of sodium 4,4′,4′′,4′′′-(thiophene-2,3,4,5-tetrayl)tetrabenzenesulfonate (TPS). Jeffamine-included β-cyclodextrin (JA-included β-CD) with cationic ammonium terminals was used as a rigid component to complex with the sulfonate anions of TPS, resulting in an iTP–CD-JA complex, which was used for characterization. Comparison was also made with a complex of iTP–JA prepared from the complexation of TPS with flexible JA chains. Without the incorporation of rigid β-CD rings, the emission efficiency of the iTP–JA complex was inferior to that of the iTP–CD-JA complex. The role of a rigid template in imposing effective RIR on an AEE-active luminogen was thus demonstrated in this study.
Introduction
As the first-discovered molecule with aggregation-induced emission (AIE) properties, 2,3,4,5,6-pentaphenyl-1-methylsilole1,2 (PMS) exhibits interesting emission behaviour, in which the non-luminescent dilute solution of PMS can be tuned to emit intensely in the solution aggregated state. With the beneficial emission properties in the aggregated state, many AIE-active luminogens containing tetraphenylethylene,3,4 phenothiazine,5,6 anthracene,7,8 triphenylethylene,9,10 distyrylanthracene,11,12 carbazolyl13,14 groups and AIE-active metal complexes15,16 have been prepared and characterized, and for practical aspects, AIE-active materials were also explored extensively in biomedical applications.17–19 Theoretical studies20–22 on AIE-active luminogens of different molecular shapes have concluded that the restriction of intramolecular rotations and vibrations (RIR and RIV) are the main causes for the AIE phenomena observed in the propeller-shaped and shell-like luminogens, respectively. Most AIE-active luminogens are propeller-shaped molecules, and therefore efficient RIR is considered to be the most important mechanism for the AIE-related emission behaviour. With efficient RIR in the aggregated state, the non-radiative decay pathways of the exciton via vibrational/torsional energy relaxation can be largely reduced and result in the desired enhanced luminescence. With the beneficial strong emission in the solid application state, several organic and polymeric materials23–28 with AIE or aggregation-enhanced emission (AEE) properties have been developed and efficiently characterized.
Conceptually, the molecular rotation of organic luminogens can be hindered internally or externally. Internally, bulky substituents with a high rotation energy barrier can be introduced via chemical bonds.23,25,29–31 Externally, the molecular motion of luminogens can be effectively frozen in a rigid media; thus, a viscous polymer matrix can be used as rigid matrix to impose an effective rotational restriction on the AIE-active luminogens, causing the desired emission enhancement of the luminogen/polymer blend. To ensure full miscibility between the luminogens and polymer matrix, hydrogen bonds (H bond)32–39 and electrostatic interactions40,41 have been previously used to generate AIE-active blend systems with intense luminescence. Bound by the viscous polymer chains through preferable H bonds or ionic interaction forces, the AIE-active luminogens are considerably restricted in rotational motion, and therefore they emit intensely.
Unlike the pair-like H bond interactions, the electrostatic interactions involved in ionic species are non-site specific. To accomplish complexation, AIE-active luminogens must be functionalized with cationic (or anionic) groups to complex with polyelectrolytes (PELs) of the opposite charge. After complexation, the hydrophobic aromatic luminogens tend to form aggregates that are phase-separated from hydrophilic PELs. The resulting complexes thus contain two phases stabilized by an intermediate ionic layer. An ammonium-functionalized tetraphenylthiophene (TP) derivative,41 TP-NH3+, was therefore synthesized and reacted with different amounts of anionic PEL of poly(sodium vinylsulfonate) (PSV). The non-site specific long-range electrostatic interaction forces and the self-aggregation of TP-NH3+ species increased with the increasing PSV content; therefore, the emission of the PEL-stabilized TP-NH3+ aggregates can be successfully increased by increasing PSV content in the solution and solid states. Conversely, adding NaCl will dissociate the long-range electrostatic interactions, resulting in the emission quenching of the complex blends.
Cyclodextrin molecules (CDs) are cyclic oligosaccharides made up of six (α), seven (β), eight (γ) or more glucopyranoside units,42 and the three-dimensional structure of the CDs can be considered as a hollow truncated cone (host) for including small molecules43–45 or linear polymers46–52 to generate different polypseudorotaxanes or polyrotaxanes for potential applications in the controlled release of drugs in living organisms and drug delivery.53–55 The hydrophobic cavity of CDs is also an ideal location to impose efficient RIR for AIE-active luminogens. A diboric acid-functionalized tetraphenylethene (TPE) derivative56 can cooperatively bind to two pairs of alternative diols on a β-CD, resulting in a highly emissive solution due to the effective RIR of the CD-incorporated TPE derivative. More recently, TPE was also linked to the α-, β- and γ-CDs57 through ester bonds and the resulting complexes exhibited varied emission responses that depended on the cavity size of the CDs. The solution emission of the β-CD-linked complex was weaker than that of the α-CD-incorporated complex but was stronger than that of the one linked with γ-CD. The highest emission efficiency of the α-CD-linked complex is because of the smaller cavity of α-CD that more effectively restricts the motion of luminogen than the larger-sized β- and γ-CD cavities. Recently, AIE-active amphiphilic tetraphenylethylene derivatives58 were prepared and reacted with γ-CD to obtain new insight into AIE behaviour. When included in the cavity of γ-CD, the amphiphilic luminogens showed an enhanced monomer emission and a decreased aggregate emission. All the examples illustrated the use of CD in constructing new AIE-active systems.
This study tests the concept that a rigid Jeffamine-included β-CD can serve as an efficient template in imposing rotational restriction to an AEE-active complex, which is stabilized by long-range electrostatic interactions. To demonstrate the important role of chain rigidity, Jeffamine (H-JA), as the representative flexible chain, and the β-CD-included Jeffamine (iCD-JA) with ammonium terminals (Scheme 1), as the representative rigid chain, were employed as cationic components to complex with sulfonate anions in an AEE-active luminogen of sodium 4,4′,4′′,4′′′-(thiophene-2,3,4,5-tetrayl)tetrabenzenesulfonate (TPS), generating the respective flexible iTP–JA and rigid iTP–CD-JA complexes for comparison. Stabilized by long range electrostatic interaction forces, the hydrophobic TPSs associate to form aggregates separated from the hydrophilic Jeffamine (H-JA) or β-CD-included Jeffamine (iCD-JA). For iCD-JA, the outer β-CD rings will stiffen the flexible Jeffamine chain and result in a rigid template that would be efficient in imposing rotational restriction to the neighbouring TPSs. Without the rigid β-CD as an outer jacket, the flexible chains of H-JA are less effective in restricting the molecular motion of TPS; thus, the emission efficiency of flexible iTP–JA complex is expected to be inferior to that of the rigid iTP–CD-JA complex. A study on the emission behaviour of TPS, iTP–JA and iTP–CD-JA helped in elucidating the important role of RIR on the AEE-active luminogens.
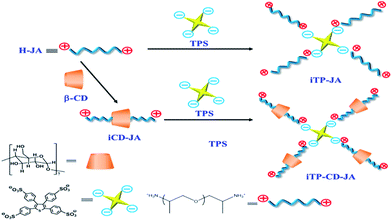 |
| Scheme 1 Chemical structures of Jeffamine and TPS and the preparation of iTP–JA and iTP–CD-JA complex. | |
Experimental
Materials
Dichloromethane, methanol, hydrochloric acid and sodium hydroxide were purchased from Aldrich Chemical Co. Chlorosulfonic acid was purchased from Lancaster Synthesis. Jeffamine D-400 (diamino-terminated poly(propylene glycol)) was purchased from Alfa Aesar. β-Cyclodextrin was purchased from Tokyo Chemical Industry Co. TP was prepared according to a reported procedure.59
Instrumentations
The 1H and 13C NMR spectra were recorded by a Varian VXR-500 MHz instrument. UV-vis absorption spectra were recorded with an Ocean Optics DT 1000 CE 376 spectrophotometer. Emission spectra were obtained from a LabGuide X350 fluorescence spectrophotometer using a 450 W Xe lamp as a continuous light source. Ultraviolet-visible (UV-vis) absorption spectra were recorded with an Ocean Optics DT 1000 CE 376 spectrophotometer. A quartz cell with dimensions of 0.2 × 1.0 × 4.5 cm3 was used for the UV-vis absorption and emission spectra measurements. Solution quantum yield (ΦF) was determined by comparing with a quinine sulfate standard solution (10−4 M in 1 N H2SO4). The quantum yield of the solid samples was measured from an integration sphere made by Ocean Optics. Fourier-transform infrared (FTIR) spectra were obtained on a Bruker Tensor 27. Elemental analyses were performed on an Elementary Vario EL-III C, H, N, O and S analyzer. The decomposition temperature and char yield of the complexes were obtained from a TA Q-50 thermogravimetric analyzer (TGA) with a scan rate of 10 °C min−1.
Synthesis of sodium tetraphenylthiophenesulfonate (TPS)
In a nitrogen-blanketed and stirred solution of TP (2.0 g, 5.15 mmol) in dichloromethane (30 mL), chlorosulfonic acid (1.54 mL, 23.18 mmol) was added dropwise. The reaction mixture was then stirred at room temperature for 24 h before being poured into crushed ice. Into the ice-cooled mixture, aq. NaOH (0.5 N) was added slowly until pH > 9 was obtained. The precipitate was filtered and recrystallized from distilled methanol followed by vacuum drying at 80 °C for 24 h to obtain a white solid product (2.3 g, 56.1%). 1H NMR (500 MHz, D2O) (ppm): 7.72, 7.71 (d, 4H, aromatic H), 7.63, 7.61 (d, 4H, aromatic H), 7.47, 7.45 (d, 4H, aromatic H), 7.22, 7.20 (d, 4H, aromatic H) (Fig. S1A†), 13C NMR (500 MHz, D2O) (ppm): 141.53, 141.22, 139.128, 138.80, 138.72, 136.27, 131.32, 129.64, 125.74, 125.35 (Fig. S1B†). MS m/z: calcd for C28H16Na4O12S5: 795.9; found, 796.6 (M+); anal. calcd for C28H16Na4O12S5: C, 42.21%; H, 2.02%; O, 24.10%; S, 20.12%. Found: C, 42.32%; H, 2.08%; O, 24.07%; S, 20.1%.
Preparation of H-JA
Hydrochloric acid (1.18 M, 2 mL) was dropped slowly into a solution of Jeffamine (2.92 g) in deionized water (4 mL). The solution was stirred vigorously overnight. The resulting stock solution (1.13 M) of H-JA was stored in a refrigerator when not in use.
Preparation of iCD-JA complex from H-JA and β-CD
Into the solution of β-CD (0.38 g, 0.335 mmol) in deionized water (15 mL), the stock solution of H-JA (0.2 mL, 0.067 mmol) was slowly added dropwise. The resulting mixture was subjected to ultrasonic agitation for 24 h before precipitation from methanol. The white precipitant was collected by filtration and dried in a vacuum oven at 50 °C for 24 h. The stoichiometry of the resulting complex was determined by the solution 1H NMR spectrum of iCD-JA (Fig. 3). The number of the included β-CD per Jeffamine chain was 1.1, calculated from the integrated ratio between propylene methyl protons (peaks a and b in Fig. 3) of Jeffamine and the aromatic protons of TPS.
Preparation of iTP–JA complex
Solution of TPS (0.025 M) in deionized water was added into the solutions of H-JA and the resulting mixtures were ultrasonically mixed for 24 h. The solvent was then removed from the product solution using a rotary evaporator and the final product was obtained after drying in a vacuum oven at 50 °C for 24 h.
Preparation of iTP–CD-JA complex
An inclusion complex of iCD-JA was primarily prepared by ultrasonically shaking the solution of β-CD (0.38 g, 0.335 mmol) and H-JA (0.2 mL, 0.067 mmol) in deionized water (10 mL). To the resulting mixtures, a solution of TPS (0.025 M) in deionized water (5 mL) was subsequently added slowly. After shaking for another 24 h, methanol was then added to precipitate white powder from the solution. The final product was obtained by drying the filtered solid at 50 °C under vacuum for 24 h.
Results and discussion
The key material (Scheme 1) used for the formulations of iTP–JA and iTP–CD-JA complexes is the water-soluble luminogen of TPS. This new AIE-active luminogen of TPS was prepared from the sulfonation reaction of TP. Similar to the TP precursor, the ionic TPS is an AEE-active material; however, because an AEE-active TPS is the premise for an AEE-active complex system, we need to identify the AEE property of TPS before further characterization of the complex system.
AEE emission behaviour of TPS
The AEE property of the water-soluble TPS can be verified by its emission responses towards concentration and aggregation. The concentration effect was confirmed by the emission spectra of the solution and solid TPS (Fig. 1A). The emission spectra, shown in Fig. 1A, basically contains two overlapping bands with the short- (at ∼415 nm) and the long-wavelength (at ∼480 nm) bands from the monomer and aggregate emissions, respectively. For the solution samples, increasing the solution concentration from 10−5 to 10−3 M resulted in the increase of intensity for both the emissions, and for the most condensed solid sample, both the resolved monomer and aggregate emissions are stronger than the solution emissions. With extensive aggregation and frozen molecular motion, the solid sample should exhibit higher emission intensity because it has a more efficient rotational restriction compared to the mobile solution state. Aggregation is beneficial for the aggregation emission, and thus the fraction of the aggregation emission continuously increased as the concentration of TPS was increased from 10−5 to 10−3 M. Indeed, the most condensed solid sample possessed the highest fraction of aggregation emission among all the spectra, as shown in Fig. 1A. The concentration-enhanced emission is therefore correlated with the AEE property.
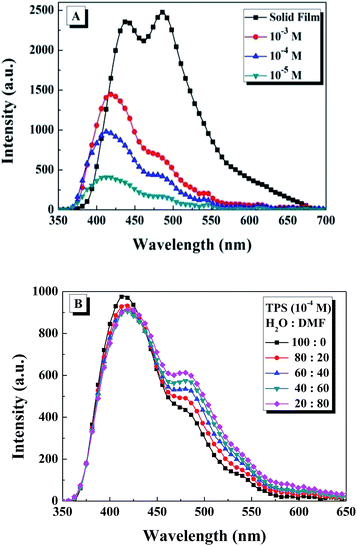 |
| Fig. 1 The emission spectra of (A) the aqueous TPS solutions of different concentrations and (B) the TPS (10−4 M) in H2O–DMF mixtures at different volumetric ratios (λex = 350 nm). | |
Aggregates formed upon non-solvent inclusion can also change the emission behaviour of the AEE-active material. Herein, DMF is a poor solvent used to generate suspended aggregates from the aqueous TPS solution. As illustrated in Fig. 1B, increasing the content of DMF from 0 to 80 vol% slightly changed the emission spectra but careful inspection indicates a consistent trend that the fraction of aggregate emission gradually increases, at the sacrifice of monomer emission, with increasing DMF content in the solution. Although small in magnitude, the systematic variations of the monomer and aggregate emissions still illustrate the important role of aggregation on the emission of TPS; therefore, the water-soluble TPS is an AEE-active luminogen.
Solution properties of the complexes
Mixtures of TPS and H-JA (or iCD-JA) with an equivalent molar ratio of ammonium cation and sulfate anion were primarily mixed in deionized water to generate stable aqueous solutions, from which a solid complex of iTP–JA (or iTP–CD-JA) could be obtained after water removal. Before discussion on the solid system, a primary study of the solution precursors was conducted with the purpose of clarifying the relationship between RIR and AEE-related emission behaviour.
The solution emission spectra, shown in Fig. 2A, were obtained under the condition in which the concentration of the fluorescent TPS was kept at a constant value of 10−2 M. Because the content of the luminogen was the same, the resulting emission spectra should also be the same if no other environmental factor is involved. The spectra, presented in Fig. 2A, are nevertheless different, with the emission intensity varied in the order of iTP–JA > iTP–CD-JA > TPS. The emission difference is nevertheless due to the absorption differences between the solutions because the corresponding UV-vis spectra, shown in Fig. 2B, shows a relative absorbance order of TPS > iTP–JA > iTP–CD-JA. To have a more accurate assessment on the emission behaviour, we measured the solution quantum efficiency (ΦF) using quinine sulfate as the standard.
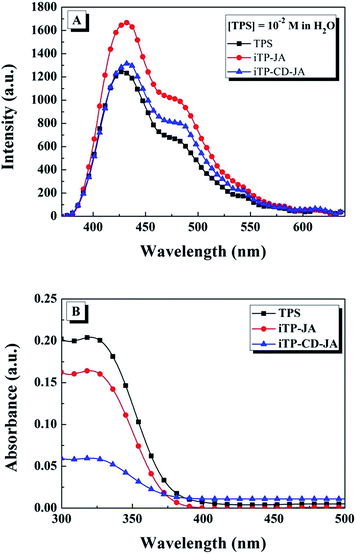 |
| Fig. 2 Solution (A) emission and (B) absorption spectra of pure TPS, iTP–JA and iTP–CD-JA complex in H2O ([TPS] = 10−2 M, λex = 350 nm). | |
By taking into account the data obtained from the absorption and emission spectra, it can be observed that the resulting ΦFs (Table 1) actually follow an order of iTP–CD-JA > iTP–JA > TPS, which is essentially different from the emission intensity order shown in Fig. 2A. Upon photo irradiation, the excited iTP–CD-JA species actually emitted more efficiently than the excited iTP–JA and excited TPS. The resolved ΦFs reflect the relative rotational restriction imposed by the surrounding environment. Compared to the flexible H-JA, the rigid iCD-JA is more efficient in imposing rotational restriction on the neighbouring TPS luminogens. We may also indirectly conclude that the β-CDs in iCD-JA are indeed threaded by the Jeffamine chain because without the incorporation of rigid β-CDs, the iCD-JA in the complex should not exert a different extent of rotational restriction to result in an emission that is essentially distinguished from the flexible iTP–JA. Without the polymer ingredient, the small-mass TPSs are free to move in the mobile water media, rendering an emission with the least efficiency among the three solution samples. Effective rotational restriction is the key factor controlling the solution emission, and we can also evaluate the relative extent of rotational restriction by solution 1H NMR spectroscopy.
Table 1 Quantum yield (ΦF) of TPS, iTP–JA, and iTP–CD-JA in the solution and solid states
Sample |
ΦFa (solution) |
ΦFb (solid) |
Measured by using an quinine sulfate standard (in 1 N H2SO4). Measured from an integration sphere. |
TPS |
0.031 |
0.18 |
iTP–JA |
0.059 |
0.21 |
iTP–CD-JA |
0.117 |
0.36 |
The 1H NMR band shape analysis has been previously utilized in the studies of rotation-induced conformational changes around the axes of the single bonds linked to the central rings of an AIE-active silole derivative.60 The fast conformational exchanges caused by the fast molecular rotations result in sharp resonance peaks, whereas the slower exchanges due to restricted molecular rotation broaden the resonance peaks. Accordingly, the 1H NMR band shape analysis can be used to evaluate the relative extent of rotational restriction in the solutions of TPS, iTP–JA and iTP–CD-JA. Except the spectrum of pure β-CD, the 1H NMR spectra of TPS, iTP–JA and iTP–CD-JA (Fig. 3) were all obtained on a solution with the same TPS concentration (=10−2 M) to eliminate potential complication due to the differences in concentration. In the spectral range of 7–8 ppm, the spectrum of pure TPS contains four large and sharp doublets in correlation to the resonances of aromatic protons of TPS. The sharp aromatic resonance peaks broadened and reduced in intensity for the solutions of iTP–JA and iTP–CD-JA. The viscous polymer chains of H-JA and iCD-JA therefore impose effective rotational restriction on the neighboring TPSs, resulting in sluggish response of the aromatic protons of TPS toward the stimuli of the external magnetic field. Comparatively, the rigid iCD-JA is more effective in imposing rotational restriction than the flexible H-JA because the aromatic resonance peaks in the spectrum of iCD-JA are broader and exhibit lower intensity than those in the spectrum of iTP–JA. The band shape analysis revealed that the relative rotational restriction in the three systems is in the order iTP–CD-JA > iTP–JA > TPS, which is in correlation with the emission intensity shown in Fig. 2A.
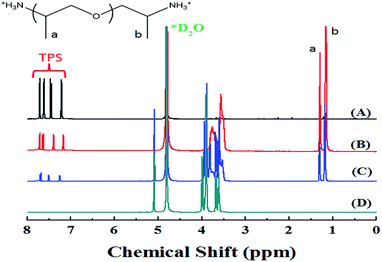 |
| Fig. 3 1H NMR spectra of (A) pure TPS, (B) iTP–JA, (C) iTP–CD-JA and (D) β-CD in D2O ([TPS] = 10−2 M). | |
Inclusion of Jeffamine also changed the proton resonances of β-CD. A previous study61–63 indicates that the inclusion of polymer causes upfield shifts of the resonance peaks of β-CD, and the chemical shifts of the inside protons, namely, H3 and H5 (Fig. 4), are slightly higher than other protons (H1, H2, H4 and H6) located at outside layer. Fig. 4 is the solution 1H NMR spectra of β-CD and iTP–CD-JA in the spectral range (3.4 to 5.2 ppm) covering the resonance peaks of β-CD. Values of chemical shifts observed in Fig. 4 are summarized in Table 2 to illustrate the chemical shift differences caused by polymer inclusion. Due to the high electronic density, all the resonance peaks of iTP–CD-JA appear at a higher field than those of pure β-CD. However, the up-field shifts are especially significant for the interior protons of H3 and H5, and the up field shifts are therefore attributed to the polymer inclusion. This result demonstrates the successful preparation of the polymer-included complex system.
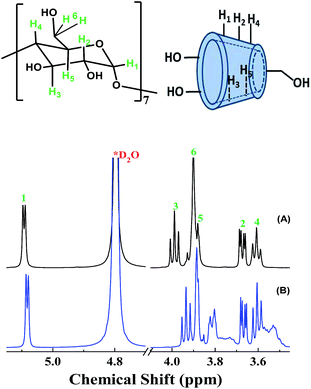 |
| Fig. 4 Solution 1H NMR spectra of (A) pure β-CD (B) iTP–CD-JA in D2O. [TPS] = 10−2 M. | |
Table 2 Chemical shifts (ppm) of resonance peaks for pure β-CD and iTP–CD-JA complex
Sample |
H1 |
H2 |
H3 |
H4 |
H5 |
H6 |
Chemical shift difference between β-CD and iTP–CD-JA. |
β-CD |
5.0928 |
3.6733 |
3.9884 |
3.6074 |
3.8799 |
3.9005 |
iTP–CD-JA |
5.0825 |
3.6679 |
3.9347 |
3.6049 |
3.8135 |
3.8848 |
Δδa |
0.0103 |
0.0054 |
0.0537 |
0.0025 |
0.0664 |
0.0157 |
Solid properties of the complexes
By comparing TGA thermograms of β-CD, TPS, iTP–JA and iTP–CD-JA (Fig. 5) solids, we were able to verify that β-CDs in iTP–CD-JA were indeed threaded by Jeffamine. Pure TPS, β-CD and two complexes of iTP–JA and iTP–CD-JA all showed an early weight-loss at low temperatures (<100 °C) due to the absorbed water; except that, the resolved decomposition traces should be true reflection of the thermal stability of all samples. TPS, having a small-mass, exhibited a superior thermal stability with the major decomposition initialized at a high temperature (>500 °C). The high thermal stability of TPS is due to the ionic sodium sulfonate bonds because the neutral analogue of tetraphenylthiophene (TP) decomposes at a considerably low temperature (<300 °C, Fig. S2†). In contrast to the stable TPS, pure β-CD is considerably unstable and its decomposition starts at a low temperature of ∼332 °C (ref. 64) and proceeds rapidly with little residues at temperatures above 350 °C. Therefore, the incorporation of β-CD is detrimental to the thermal stability of the complexes. In contrast, the TPS component acts to reinforce thermal stability to the complexes as evidenced by the late decomposition of the iTP–JA complex. With both β-CD and TPS in the complex, iTP–CD-JA exhibited a dual-decomposition behaviour with the early-stage decomposition assigned to the easily-degraded β-CD at a low temperature of ∼300 °C and the second-stage high-temperature decomposition due to the degradation of the ionic TPS that began at a high temperature of 700 °C. The main decomposition at the early stage corresponds to a 49% weight-loss, which approximates the theoretical weight percentage (53%) of the β-CD rings in iTP–CD-JA. This result therefore indicates that most of the β-CDs added in the solution preparation step are still present in the solid iTP–CD-JA and are acting to rigidify the flexible Jeffamine chains, facilitating the iCD-JA template to efficiently impose the desired rotational restriction. The resulting iTP–CD-JA complex should be a good emitter with the effective rotational restriction.
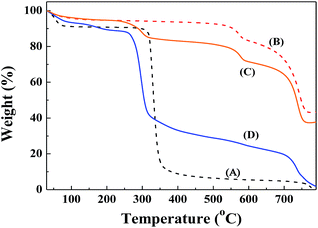 |
| Fig. 5 TGA thermograms of (A) β-CD, (B) TPS, (C) iTP–JA and (D) iTP–CD-JA (heating rate = 10 °C min−1). | |
The advantage of Jeffamine-included β-CD (iCD-JA) in immobilizing the luminogenic TPSs can be evaluated from the solid emission spectra shown in Fig. 6. Primarily, all the three solids, namely, TPS, iTP–JA and iTP–CD-JA, emit with a higher intensity compared to their solution analogues (cf. Fig. 2A). In particular, solid iTP–CD-JA showed much better emission intensity than its solution precursor. Quantum yields (ΦF) determined from the solution and the solid samples (Table 1) also illustrated the same conclusion that ΦFs of the solid samples are all comparatively higher than their solution counterparts. The high solid state emission is reasonable by considering that molecular rotation of the fluorescent TPSs should be considerably blocked in the frozen solid state. The resulting ΦF values measured from the integration sphere are in the order iTP–CD-JA (=0.36) > iTP–JA (=0.21) > TPS (=0.18). For the effective rotational restriction imposed by the rigid iCD-JA, iTP–CD-JA complex exhibited the highest ΦF of 0.36. With a lower content (wt%) of the fluorescent TPS, iTP–CD-JA, however, still exhibited a higher emission efficiency than pure TPS and iTP–JA. Effective rotational restriction, rather than the content of the luminogen, is therefore the key factor in determining the emission efficiency of the AEE-active system.
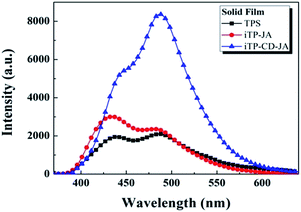 |
| Fig. 6 Solid FL spectra of pure TPS, iTP–JA and iTP–CD-JA complex (λex = 350 nm). | |
Besides the intensity differences, the most noticeable feature in Fig. 6 is the large aggregate emission, with an intensity higher than the monomer emission, shown in the spectrum of iTP–CD-JA. The large aggregate emission for iTP–CD-JA refers to a high degree of aggregation of the TPSs in the complex. In the highly-aggregated phase-separated domains, TPSs are supposed to be tightly packed and clumsy in rotation, resulting in an intense aggregated emission. The effective rotational restriction imposed by the rigid iCD-JA template is thus verified from the emission of iTP–CD-JA.
Long-range electrostatic interaction of the iTP–CD-JA complex
The emission behaviour of the iTP–CD-JA complex is closely related to the extent of the aggregation of luminescent TPS. As illustrated in Scheme 2, the molecular chain arrangements of the complex iTP–CD-JA are determined by the long-range electrostatic interactions between the cationic iCD-JA and the sulfonate anions of TPS. With non-site specific and long-range interactions, the aromatic rings of TPSs tend to associate together to form interpolymer aggregates, which are phase-separated from the hydrophilic iCD-JA chains and stabilized by the long-range electrostatic forces between the ammonium cations of iCD-JA and the sulfonate anions of TPS. The long-range electrostatic forces should be prevalent in the solution preparative state. After the removal of solvent, the solid blend that remains has the fundamental two-phase morphology, within which the TPS anions do not distribute randomly but rather form aggregates with a high local concentration. With the high local concentration, the TPS are therefore tightly packed and contribute to the large aggregate emission in the spectrum of iTP–CD-JA (Fig. 6).
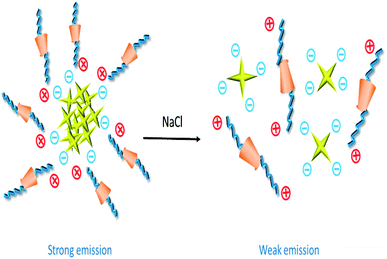 |
| Scheme 2 A schematic illustration for the association and dissociation of long-range interactions between TPS anions and iCD-JA cations. | |
In the solution preparative state, relative strength between the hydrophobic dispersion forces of the aggregated luminogens and the long-range electrostatic interactions affect the stability of the aggregated particles. The formation of aggregated TPSs is beneficial for the AEE-related emission efficiency; conversely, the dissociation of the aggregated TPS phase will lead to the lowering of the luminescence. To demonstrate, strong polyelectrolytes of NaCl were intentionally added to the complex solution of iTP–CD-JA. With small size and high ionic strength, NaCl ions are capable of penetrating into the interior of the aggregated complexes of iTP–CD-JA, dissociating the ionic ammonium-sulfate bonds between iCD-JA and TPSs.
Certain fractions of the ionic ammonium-sulfate bonds are thus ruptured by the strong NaCl electrolyte, leading to the collapse of the aggregated TPSs and the release of free TPSs (Scheme 2). The released TPSs in the aqueous media are no longer hindered in rotation as compared to when they were in the aggregated TPS; therefore, adding NaCl to the aqueous iTP–CD-JA solution results in emission spectra (Fig. 7) with comparatively lower intensity and broader features compared to the pure iTP–CD-JA without the NaCl additive. The important role of long-range electrostatic interaction on the AEE-related emission behaviour was therefore demonstrated.
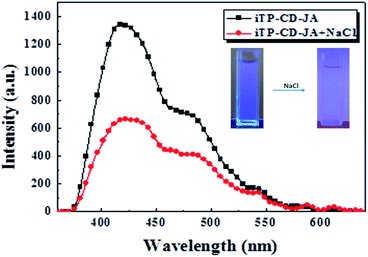 |
| Fig. 7 Emission spectra of the aqueous iTP–CD-JA solutions with and without NaCl additive ([TPS] = 10−4 M, [NaCl] = 10−2 M, λex = 350 nm) demonstrating the luminescence difference upon the addition of NaCl (λex = 365 nm). | |
Conclusion
In present work, a water-soluble luminogen of TPS was synthesized and was identified to be AEE-active by its emission responses toward concentration and aggregation. The water-soluble TPS was then mixed with H-JA and iCD-JA to generate flexible and rigid complexes of iTP–JA and iTP–CD-JA, respectively, for evaluating the relationship between rotational restriction and AEE-related emission. Results from solution 1H NMR spectra indicate that a more effective rotational restriction is operative in the iTP–CD-JA complex as compared to the flexible iTP–JA. With more effective rotational restriction, the solution of iTP–CD-JA emitted with a higher efficiency than the solution of iTP–JA.
The emission of solid iTP–JA was higher in intensity than that of small-mass TPS but was inferior to the solid iTP–CD-JA. These results suggest that the rigid iCD-JA is more effective in imposing rotational restriction on the luminogenic TPSs as compared to the flexible H-JA used in iTP–JA. Instead of the content of the luminogen in the complex, effective rotational restriction is the real operative factor controlling the emission efficiency of the complex blend.
Acknowledgements
We appreciate the financial support from the Ministry of Science and Technology, Taiwan, under the contract no. NSC 102-2221-E-110-084-MY3.
Notes and references
- J. Luo, Z. Xie, J. W. Y. Lam, L. Cheng, H. Chen, C. Qiu, H. S. Kwok, X. Zhan, Y. Liu, D. Zhu and B. Z. Tang, Chem. Commun., 2001, 1740 RSC.
- B. Z. Tang, X. Zhan, G. Yu, P. P. S. Lee, Y. Liu and D. Zhu, J. Mater. Chem., 2001, 11, 2974 RSC.
- X. Zhang, Z. Chi, H. Li, B. Xu, X. Li, S. Liu, Y. Zhanga and J. Xu, J. Mater. Chem., 2011, 21, 1788 RSC.
- Z. Wang, J.-H. Ye, J. Li, Y. Bai, W. Zhanga and W. He, RSC Adv., 2015, 5, 8912 RSC.
- J. Zhang, B. Xu, J. Chen, L. Wang and W. Tian, J. Phys. Chem. C, 2013, 117, 23117 CAS.
- S. Y. Su, H. H. Lin and C. C. Chang, J. Mater. Chem., 2010, 20, 8653 RSC.
- B. Sun, X. Yang, L. Ma, C. Niu, F. Wang, N. Na, J. Wen and J. Ouyang, Langmuir, 2013, 29, 1956 CrossRef CAS PubMed.
- X. Zhang, Z. Chi, B. Xu, C. Chen, X. Zhou, Y. Zhang, S. Liua and J. Xu, J. Mater. Chem., 2012, 22, 18505 RSC.
- H. Li, Z. Chi, B. Xu, X. Zhang, Z. Yang, X. Li, S. Liu, Y. Zhanga and J. Xu, J. Mater. Chem., 2010, 20, 6103 RSC.
- H. Li, Z. Chi, X. Zhang, B. Xu, S. Liu, Y. Zhang and J. Xu, Chem. Commun., 2011, 47, 11273 RSC.
- J. He, B. Xu, F. Chen, H. Xia, K. Li, L. Ye and W. Tian, J. Phys. Chem. C, 2009, 113, 9892 CAS.
- B. Xu, J. Zhang, H. Fang, S. Ma, Q. Chen, H. Sun, C. Imc and W. Tian, Polym. Chem., 2014, 5, 479 RSC.
- S. Dong, Z. Li and J. Qin, J. Phys. Chem. B, 2009, 113, 434 CrossRef CAS PubMed.
- J. Huang, X. Yang, J. Wang, C. Zhong, L. Wang, J. Qina and Z. Li, J. Mater. Chem., 2012, 22, 2478 RSC.
- Z. Wei, Z.-Y. Gu, R. K. Arvapally, Y.-P. Chen, R. N. McDougald Jr, J. F. Ivy, A. A. Yakovenko, D. Feng, M. A. Omary and H.-C. Zhou, J. Am. Chem. Soc., 2014, 136, 8269 CrossRef CAS PubMed.
- N. B. Shustova, T.-C. Ong, A. F. Cozzolino, V. K. Michaelis, R. G. Griffin and M. Dincă, J. Am. Chem. Soc., 2012, 134, 15061 CrossRef CAS PubMed.
- X. Zhang, X. Zhang, L. Tao, Z. Chi, J. Xub and Y. Wei, J. Mater. Chem. B, 2014, 2, 4398 RSC.
- X. Zhang, X. Zhang, B. Yang, M. Liu, W. Liu, Y. Chena and Y. Wei, Polym. Chem., 2014, 5, 356 RSC.
- M.-C. Hsieh, C.-H. Chien, C.-C. Chang and T.-C. Chang, J. Mater. Chem. B, 2013, 1, 2350 RSC.
- J. Chen, C. C. W. Law, J. W. Y. Lam, Y. Dong, S. M. F. Lo, I. D. Williams, D. Zhu and B. Z. Tang, Chem. Mater., 2003, 15, 1535 CrossRef CAS.
- J. Shi, N. Chang, C. Li, J. Mei, C. Deng, X. Luo, Z. Liu, Z. Bo, Y. Q. Dong and B. Z. Tang, Chem. Commun., 2012, 48, 10675 RSC.
- J. Mei, Y. Hong, J. W. Y. Lam, A. Qin, Y. Tang and B. Z. Tang, Adv. Mater., 2014, 26, 5429 CrossRef CAS PubMed.
- Aggregation-Induced Emission: Fundamentals, ed. A. Qin and B. Z. Tang, John Wiley & Sons, Ltd., New York, 2013 Search PubMed.
- J. Liu, J. W. Y. Lam and B. Z. Tang, J. Inorg. Organomet. Polym., 2009, 19, 249 CrossRef CAS.
- Y. Hong, J. W. Y. Lam and B. Z. Tang, Chem. Commun., 2009, 4332 RSC.
- J. Wu, W. Liu, J. Ge, H. Zhang and P. Wang, Chem. Soc. Rev., 2011, 40, 3483 RSC.
- Y. Hong, J. W. Y. Lam and B. Z. Tang, Chem. Soc. Rev., 2011, 40, 5361 RSC.
- A. Qin, J. W. Y. Lam and B. Z. Tang, Prog. Polym. Sci., 2012, 37, 182 CrossRef CAS PubMed.
- C. T. Lai and J. L. Hong, J. Phys. Chem. B, 2010, 114, 10302 CrossRef CAS.
- M. Wang, G. Zhang, D. Zhang, D. Zhu and B. Z. Tang, J. Mater. Chem., 2010, 20, 1858 RSC.
- Z. Zhao, S. Chen, J. W. Y. Lam, C. K. W. Jim, C. Y. K. Chan, Z. Wang, P. Lu, C. Deng, H. S. Kwok, Y. Ma and B. Z. Tang, J. Phys. Chem. C, 2010, 114, 7963 CAS.
- Y. Liu, X. Tao, F. Wang, J. Shi, J. Sun, W. Yu, Y. Ren, D. Zou and M. Jiang, J. Phys. Chem. C, 2007, 111, 6544 CAS.
- P. Chen, R. Lu, P. Xue, T. Xu, G. Chen and Y. Zhao, Langmuir, 2009, 25, 8395 CrossRef CAS PubMed.
- R. H. Chien, C. T. Lai and J. L. Hong, J. Phys. Chem. C, 2011, 115, 12358 CAS.
- R. H. Chien, C. T. Lai and J. L. Hong, J. Phys. Chem. C, 2011, 115, 20732 CAS.
- C. M. Yang, I. W. Lee, T. L. Chen, W. L. Chien and J. L. Hong, J. Mater. Chem. C, 2013, 1, 2842 RSC.
- W. L. Chien, C. M. Yang, T. L. Chen, S. T. Li and J. L. Hong, RSC Adv., 2013, 3, 6930 RSC.
- S. L. Deng, T. L. Chen, W. L. Chien and J. L. Hong, J. Mater. Chem. C, 2014, 2, 651 RSC.
- T. S. Hsiao, S. L. Deng, K. Y. Shih and J. L. Hong, J. Mater. Chem. C, 2014, 2, 4828 RSC.
- C. A. Chou, R. H. Chien, C. T. Lai and J. L. Hong, Chem. Phys. Lett., 2010, 501, 80 CrossRef CAS PubMed.
- R. H. Chien, C. T. Lai and J. L. Hong, Macromol. Chem. Phys., 2012, 213, 666 CrossRef CAS.
- C. R. Cristian, L. Constantin and E. T. Alan, Macromolecules, 2001, 34, 1318 CrossRef.
- T. Höfler and G. Wenz, J. Inclusion Phenom. Mol. Recognit. Chem., 1996, 25, 81 CrossRef.
- K. A. Udachin and J. A. Ripmeester, J. Am. Chem. Soc., 1998, 120, 1080–1081 CrossRef CAS.
- M. T. Stone and H. L. Anderson, Chem. Commun., 2007, 2387 RSC.
- M. Okada, Y. Kawaguchi, H. Okumura, M. Kamachi and A. Harada, J. Polym. Sci., Part A: Polym. Chem., 2000, 38, 4839 CrossRef CAS.
- J. Li, X. Ni, Z. Zhou and K. W. Leong, J. Am. Chem. Soc., 2003, 125, 1788 CrossRef CAS PubMed.
- M. V. Rekharsky and Y. Inoue, Chem. Rev., 1998, 98, 1875 CrossRef CAS PubMed.
- T. Ooya, H. S. Choi, A. Yamashita, N. Yui, Y. Sugaya, A. Kano, A. Maruyama, H. Akita, R. Ito, K. Kogure and H. Harashima, J. Am. Chem. Soc., 2006, 128, 3852 CrossRef CAS PubMed.
- X. Q. Guo, L. X. Song, F. Y. Du, Z. Dang and M. Wang, J. Phys. Chem. B, 2011, 115, 1139 CrossRef CAS PubMed.
- L. X. Song, X. Q. Guo, F. Y. Du and L. Bai, Polym. Degrad. Stab., 2010, 95, 508 CrossRef CAS PubMed.
- Y. Liu, Y. L. Zhao, Y. Chen and M. Wang, Macromol. Rapid Commun., 2005, 26, 401 CrossRef CAS.
- M. E. Davis, Mol. Pharmaceutics, 2009, 6, 659 CrossRef CAS PubMed.
- D. W. Pack, A. S. Hoffman, S. Pun and P. S. Stayton, Nat. Rev. Drug Discovery, 2005, 4, 581 CrossRef CAS PubMed.
- H. Okumura, Y. Kawaguchi and A. Harada, Macromolecules, 2001, 34, 6338 CrossRef CAS.
- Y. Liu, A. Qin, X. Chen, X. Y. Shen, L. Tong, R. Hu, J. Z. Sun and B. Z. Tang, Chem.–Eur. J., 2011, 17, 14736 CrossRef CAS PubMed.
- G. Liang, J. W. Y. Lam, W. Qin, J. Li, N. Xie and B. Z. Tang, Chem. Commun., 2014, 50, 1725 RSC.
- S. Song, H.-F. Zheng, D.-M. Li, J.-H. Wang, H.-T. Feng, Z.-H. Zhu, Y.-C. Chen and Y.-S. Zheng, Org. Lett., 2014, 16, 2170 CrossRef CAS PubMed.
- F. K. Su, J. L. Hong and L. L. Lin, Synth. Met., 2004, 142, 63 CrossRef CAS.
- J. Chen, C. C. W. Law, J. W. Y. Lam, Y. Dong, S. M. F. Lo, I. D. Williams, D. Zhu and B. Z. Tang, Chem. Mater., 2003, 15, 1535 CrossRef CAS.
- M. G. Teixeira, J. V. de Assis, C. G. P. Soares, M. F. Venâncio, J. F. Lopes, C. S. Nascimento Jr, C. P. A. Anconi, G. S. L. Carvalho, C. S. Lourenço, M. V. de Almeida, S. A. Fernandes and W. B. de Almeida, J. Phys. Chem. B, 2014, 118, 81 CrossRef CAS PubMed.
- C. Yuan, Z. Jin and X. Xu, Carbohydr. Polym., 2012, 89, 492 CrossRef CAS PubMed.
- K. P. Sambasevam, S. Mohamad, N. M. Sarih and N. A. Ismail, Int. J. Mol. Sci., 2013, 14, 3671 CrossRef CAS PubMed.
- P. J. Salustio, G. Feio, J. L. Figueirinhas, J. F. Pinto and H. M. C. Marques, Eur. J. Pharm. Biopharm., 2009, 71, 377 CrossRef CAS PubMed.
Footnote |
† Electronic supplementary information (ESI) available: Fig. S1 and S2. See DOI: 10.1039/c4ra16913a |
|
This journal is © The Royal Society of Chemistry 2015 |
Click here to see how this site uses Cookies. View our privacy policy here.