DOI:
10.1039/C4RA16637J
(Paper)
RSC Adv., 2015,
5, 25634-25641
Development of hybrid composites for automotive applications: effect of addition of SEBS on the morphology, mechanical, viscoelastic, crystallization and thermal degradation properties of PP/PS–xGnP composites
Received
18th December 2014
, Accepted 3rd March 2015
First published on 3rd March 2015
Abstract
In this article, we report on a simple and cost effective approach for the development of light-weight, super-tough and stiff material for automotive applications. Nanocomposites based on PP/PS blend and exfoliated graphene nanoplatelets (xGnP) were prepared with and without SEBS. Mechanical, crystallization and thermal degradation properties were determined and correlated with phase morphology. The addition of xGnP to PP/PS blend increased the tensile modulus at the expense of toughness. The presence of xGnP increased the enthalpy of crystallization and enthalpy of fusion of PP in the blends, without affecting segmental mobility and thermal stability. Addition of polystyrene-block-poly(ethylene-ran-butylene)-block-polystyrene (SEBS) improved the toughness of PP/PS blends, but decreased the stiffness. The incorporation of xGnP into this ternary blend generated a super-tough material with improved stiffness and tensile elongation, suitable for automotive applications. It is observed that the presence of SEBS diminished the tendency of agglomeration of xGnP and their unfavorable interactions with thermoplastics, which in turn reduced the internal friction in the matrix.
Introduction
Polymer blending is a simple but effective method to develop advanced polymeric materials. The properties of the blends can be manipulated according to the end use, by changing the concentration of the component polymers. Polypropylene (PP) is an important commodity plastic. Blending of PP with rigid polymers such as polystyrene (PS) and polyphenylene ether (PPE) improves its strength and stiffness, at the expense of toughness.1–4 However, these blends exhibit poor mechanical properties due to their inherent immiscibility and incompatibility.5 It is reported that these problems can be alleviated by the use of compatibilizing agents, which locate at the interface and reduce the unfavorable interfacial interactions6–8 and or retard the rate of coalescence process, thereby reducing the particle size and improving the blend properties.9–14
Recently researchers are interested in using nanofillers or polymer grafted nanofillers as compatibilizers for polymer blends.15–19 You et al.20 synthesized polypropylene-graft-reduced graphene oxide (PP-g-rGO) and used it as a novel compatibilizer for PP/PS immiscible polymer blends. Krishnan et al.21 studied the effect of modified kaolin clays on the mechanical properties of PP/PS 80/20 blends and observed good improvement in mechanical and thermal properties. Elias et al.22 studied the effect of two types of fumed silica (hydrophilic and hydrophobic) on the morphology of PP/PS 70/30 blends and found a significant reduction in the PS droplets size in the presence of both types of silica.
In a recent work, Al-Saleh et al.23 have successfully localized carbon black (CB) at the interface of PP/PS blend by adding SBS copolymer and reduced the percolation threshold of CB-filled (70/30) PP/PS blend. Tiwari et al.24 studied effect of modified clay on the morphology, phase stability and mechanical properties of PP/PS blends. Maleated polypropylene (PP-g-MA) was used to preferentially promote dispersion of the organoclay in the PP matrix.
In an earlier work, we reported on the compatibility and thermo-mechanical properties of PP–xGnP composites in the presence and absence of SEBS.25 The study revealed synergistic effects of SEBS and xGnP on the impact properties of PP. It was also found that internal friction in the sample decreased considerably by the addition of SEBS in PP–xGnP composites. In this context, this study aims at investigating the effect of addition of SEBS on the physical and mechanical properties of the PP/PS–xGnP composites. The addition of amorphous PS into crystalline PP is not only intends to increase the strength but also justifies the use of SEBS, which tends to decrease the internal friction between the components in the blends and reduce the tendency of agglomeration of xGnP. The amount of SEBS, which plays a key role in the interaction between the PP, PS and xGnP in PP/PS blends, is set as 20 wt% to obtain the matrix with optimum stiffness and toughness.
Experimental
Materials and preparation of blends
PP homopolymer, grade 1110 MAS with a melt flow index of 16.3 g per 10 min (230 °C per 5 kg) having density 0.9 g cm−3 was supplied by Indian Oil Corporation. PS grade Polystyrol 147F GR21, having density 1.05 g cm−3 was supplied by Styrolution India Pvt. ltd. PS has a melt flow index of 6.5 g per 10 min (200 °C per 5 kg). Compatibilizer, polystyrene-block-poly(ethylene-ran-butylene)-block-polystyrene (SEBS) with average Mw ∼ 118
000 by GPC, contains >0.03% antioxidant as inhibitor, was obtained from Sigma Aldrich. Exfoliated graphene nanoplatelets (xGnP) – grade M with 99.5% carbon was obtained from XG Science, Inc. The xGnP have an average thickness of 6 to 8 nanometers and surface area of 120–150 m2 g−1. PP/PS (80/20) blends were prepared by melt mixing, using Thermo Haake Polylab QC system equipped with roller rotors. The mixing was done at 180 °C with a rotor speed of 50 rpm for 8 minutes. For making PP/PS–xGnP composites, PP and PS was melt mixed for two minutes, followed by the addition of xGnP. Similarly, for compatibilized composites initially PP and PS were melt mixed for two minutes, followed by the addition of compatibilizer and xGnP. The total mixing time is 8 minutes. The concentration of xGnP used was 0.1, 0.3, 0.5, and 1 wt%. Each component is added one by one in the above mentioned order, once the torque becomes minimum. The resulting blends and composites were hot pressed into sheets and cut in to pieces and injection molded in a DSM explore, Micro 12cc injection molding machine at 190 °C, for preparing the test specimens for impact and tensile testing as per relevant ISO standards.
Characterization
Scanning electron microscopy (SEM).
The morphology of the blends and composites was examined with a Zeiss Supra 55VP field emission gun scanning electron microscope (SEM) at an activation voltage of 5 kV with a working distance of 10 mm. The cryo-fractured surfaces were coated with thin layers of gold to avoid charging.
Dynamic mechanical analysis (DMA).
Dynamic mechanical analysis was carried out using DMA Q-800 TA instruments, to study the viscoelastic behavior of PP/PS blends. Rectangular specimens of 60 × 12 × 3 mm3 dimensions were used for the study. Analysis was done using a dual cantilever clamp at a dynamic frequency of 1 Hz in the temperature range 35 to 130 °C with a ramp of 3 °C min−1.
Thermo gravimetric analysis (TGA).
The thermal stability of the polymer blends was analysed using a TGA-Q-50 TA instrument in nitrogen atmosphere. The sample weight of about 5–7 mg was used and test was carried out from room temperature to 600 °C at a heating rate of 20 °C min−1.
Differential scanning calorimetry (DSC).
Thermal properties were determined using Mettler Toledo DSC 822e differential scanning calorimetry. The parameters such as crystallization temperature (Tc), melting temperature (Tm), total enthalpy of crystallization (ΔHc), total enthalpy of fusion (ΔHf) and Xc (%) percentage crystallinity are derived from the DSC thermogram. Samples of approximately 10 mg were placed into ceramic pans and the tests were performed in a dry nitrogen atmosphere (flow rate of 20 mL min−1). The heating was done from −50 to 200 °C at a heating rate of 10 °C min−1, followed by cooling to room temperature at 10 °C min−1. The melting and crystallization were determined from the DSC heating and cooling curves.
ΔHf and ΔHc were obtained from the areas under the melting and crystallization peaks. Indium and silver samples were used as calibration standards.
Mechanical properties
The tensile properties of the samples were measured using a universal testing machine (Tinius Olsen) model H 50 KT at a cross head speed of 50 mm min−1, according to ISO 527 on dumbbell shaped specimens. The sample dimensions were 75 × 5 × 2 mm3. The span length used was 55 mm. Impact testing was carried out according to ISO 180 using a Resil impactor junior. Unnotched samples were used for testing. The sample dimensions were 80 × 10 × 4 mm3.
Results and discussion
Mechanical properties
The incorporation of xGnP has no significant effect on the impact strength and tensile strength of PP/PS blend (Fig. 1a). Although the tensile elongation of the blend decreased by about 40%, tensile modulus increased by about 15% (Fig. 1b). The results reveal that graphene nanoplatelets have no reinforcement effect in PP/PS blends, but suggest that the PP/PS interfaces are so weak that they cannot transfer stresses between the PP matrix and the PS dispersed phase, even in the presence of xGnP. This indicates that nanoparticles are not well dispersed in the blend or blend interface and contribute nothing to diminish the unfavourable cross-correlations between PP and PS chains in the blend. Reduction in tensile elongation with increasing concentration of xGnP in the blend implies their greater tendency for agglomeration. An increase in tensile modulus is not unexpected as it is measured at very low strain level.
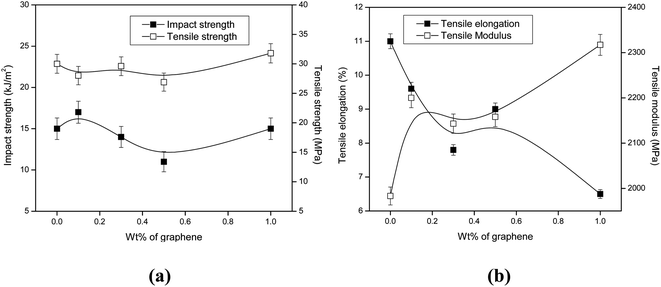 |
| Fig. 1 Mechanical properties of PP/PS–xGnP composites. | |
SEM micrographs (Fig. 2) demonstrate the phase morphology of the blends with and without nanoplatelets. Presence of xGnP have no significant impact on the phase morphology of the blends, except that the dispersed particle size decreased. All the blends showed two-phase, coarse and non-uniform morphology indicating a high interfacial tension and coalescence due to the absence of favorable interfacial interactions.
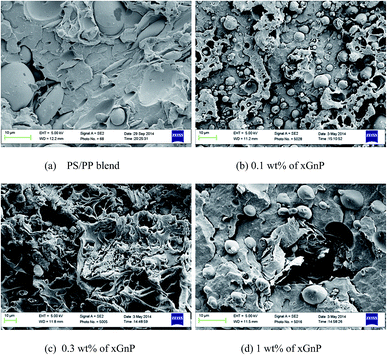 |
| Fig. 2 Scanning electron micrographs of PP/PS–xGnP composites. | |
It is obvious from Fig. 3a that the addition of SEBS increased the impact strength of PP/PS blends appreciably (ca. 200%) and decreased the tensile strength only marginally. It should be noted that the presence of nanoplatelets in the ternary system made no significant change in impact strength, except at highest concentration (1 wt%). Similarly, tensile strength of the ternary system is only slightly affected by the presence of xGnP. It is important to note that addition of SEBS in PP/PS blends resulted in a drastic reduction in tensile modulus (ca. 40%), which may severely restrict its end use applications due to poor stiffness. But the incorporation of even 0.1 wt% of xGnP effectively recompensed this serious constraint by enhancing the tensile modulus of the ternary system by more than 15%. Tensile elongation registered a remarkable improvement. Addition of SEBS into PP/PS blends increased the tensile elongation of the blends by ca. 250%. More importantly, incorporation of only 0.1 wt% of xGnP increased the tensile elongation of the ternary system by about 65% (ca. 500% increase compared to the PP/PS blends). Thus PP/PS–SEBS–xGnP (0.1 wt%) composites exhibit good impact strength (ca. 140% greater than PP/PS blends), excellent tensile elongation (ca. 500% greater than PP/PS blend), reasonably good strength (ca. 15% less than PP/PS blends) and stiffness (ca. 25% less than PP/PS blends). This makes this composite material suitable for automotive applications.
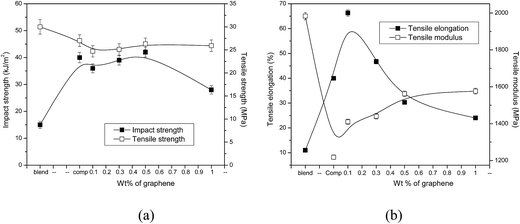 |
| Fig. 3 Mechanical properties of PP/PS–SEBS–xGnP composites. | |
SEM micrographs of PP/PS–SEBS–xGnP composites (Fig. 4) illustrate the dramatic change in the phase morphology, in the presence of SEBS. The matrix/droplet type morphology of PP/PS–xGnP composites is transformed to an interpenetrating, co-continuous type morphology. It is important to mention that the presence of SEBS will decrease the unfavourable cross-correlations between the PP/PP chains. Note that the SEBS is partially miscible with the PS phase (due to the presence of styrene units) and its segments exhibit less unfavourable cross-correlations with the PP chains (due to the presence of ethylene units). Thus SEBS strengthens the interface between the component polymers and improves the interfacial adhesion. The reinforced interface can transfer the stress between the components more effectively and thus enhance the mechanical properties, except stiffness. Now, the incorporation of xGnP into this ternary system makes the difference. The nanoplatelets will diffuse through the polymer chains, finely disperse among the soft SEBS segments and enhance the overall stiffness of the system. A fraction of particles will find their locations at the polymer interface and fill the voids there, if any and contribute towards the strengthening of the interface. Thus the overall effect is a substantial reduction of the tendency of nanoparticles to undergo agglomeration.
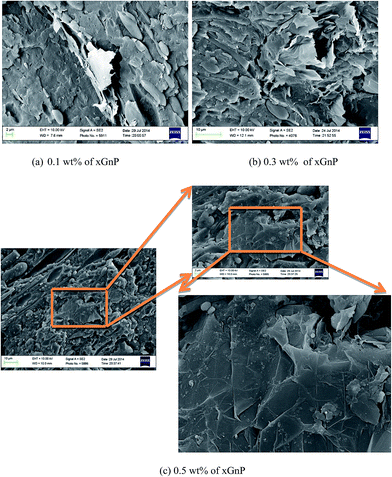 |
| Fig. 4 SEM micrographs of PP/PS–SEBS–xGnP composites. | |
Viscoelastic properties
The storage modulus of PP/PS blends (Fig. 5a) increased with the addition of xGnP. The increase in storage modulus indicates increased stiffness attributed to the reinforcing effect of graphene, which is one of the stiffest known materials.26 Further, the graphene sheets are able to reduce the mobility of the polymer chains by interfering the segmental motion, which also contributes to the enhancement of the storage modulus. It is seen that the storage modulus decreases with temperature since the short range segmental motions that initially gave rise to the glass transition of PP occur very much faster. Note that the long range cooperative motion of chain segments that would result in translational motions of complete molecules is still restricted by the presence of chain entanglements which act as temporary cross links. The Tg of PS phase is observed as 110 °C, where the amplitude of vibrational motion of PS segments becomes greater, and eventually, the thermal energy becomes comparable to the potential energy barrier for the segment vibration.
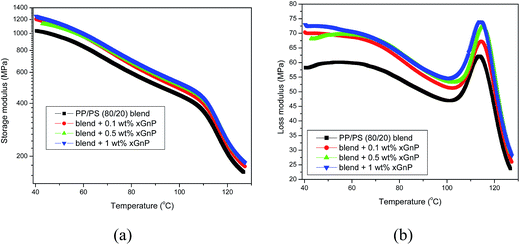 |
| Fig. 5 Plot of (a) storage modulus and (b) loss modulus of PP/PS–xGnP composites. | |
The loss modulus profile of PP/PS–xGnP composites is shown in Fig. 5b. All nanocomposites exhibited higher loss modulus values compared to the neat PP/PS blends. This is expected, since excessive heat is generated by application of applied stress due to the filler–filler and filler polymer interactions. Thus more heat is dissipated from the nanocomposites. The main peak at around 114 °C corresponds to the Tg of the PS phase. From the loss modulus profile, it is clear that the incorporation of xGnP causes slight broadening of peak width of the loss modulus curves. The peak broadening may be due to the interactions between the xGnP and the polymer.27 Note that the Tg of the PS phase was not affected by the addition of the xGnP.
It is obvious that storage modulus values of ternary systems (Fig. 6a) are considerably lower than those of binary system (Fig. 5). Thus the addition of SEBS decreased the stiffness of the system. The storage modulus of ternary blends increased with xGnP concentration, which implies an enhancement of stiffness by the incorporation of graphene platelets. It is also important to note that the loss modulus of ternary system increased with the addition of xGnP. The higher loss modulus is due to the excessive heat generation due to friction, because of the polymer–filler interactions. But, the loss moduli of PP/PS–SEBS–xGnP composites are less than those of corresponding PP/PS–xGnP composites. This implies the overall reduction in internal friction or improvement in damping behavior of the composites.
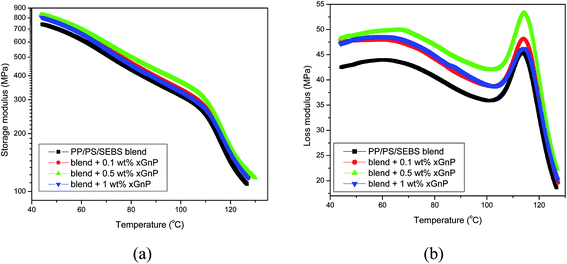 |
| Fig. 6 Plot of (a) storage modulus and (b) loss modulus of PP/PS–SEBS blend and PP/PS–SEBS–xGnP composites. | |
DSC measurements
Melting and crystallization properties of binary and ternary systems with and without graphene computed from the DSC heating and cooling thermograms (Fig. 7) are summarized in Table 1. The parameters, melting point (Tm) and enthalpy of fusion (ΔHf) are estimated from the DSC heating curves while crystallization temperature (Tc) and enthalpy of crystallization (ΔHc) were determined from the cooling curves. The degree of crystallinity of nanocomposites can be determined using the equation: | 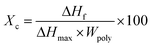 | (1) |
where ΔHf is the normalized enthalpy of fusion, ΔHmax is the enthalpy of fusion for a theoretically 100% crystalline polymer and Wpoly is the weight fraction of PP in the blend. The term ΔHmax is a reference value and represents the heat of fusion if the polymer is 100% crystalline, this value for PP is 207.1 J g−1.
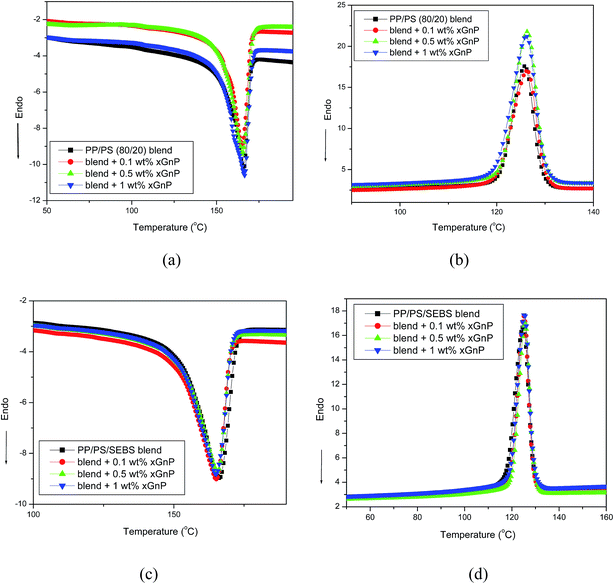 |
| Fig. 7 (a) Heating and (b) cooling thermograms of PP/PS–xGnP composites, (c) heating and (d) cooling thermograms of PP/PS–SEBS–xGnP composites. | |
Table 1 DSC summary of Tm, Tc, ΔHm, ΔHc and Xc for neat PP/PS, PP/PS–SEBS, PP/PS–xGnP and PP/PS–SEBS–xGnP systems
T
m
|
T
m (°C) |
T
c (°C) |
ΔHm (J g−1) |
ΔHc (J g−1) |
X
c (%) |
PP/PS blend (80/20) |
166.03 |
125.71 |
66.78 |
80.57 |
40.31 |
Blend + 0.1 wt% xGnP |
164.97 |
126.27 |
75.40 |
87.42 |
45.55 |
Blend + 0.5 wt% xGnP |
165.11 |
126.27 |
74.86 |
85.14 |
45.41 |
Blend + 1 wt% xGnP |
166.56 |
126.09 |
70.91 |
87.78 |
43.23 |
Compatibilized blend
|
166.30 |
124.92 |
55.94 |
68.37 |
40.52 |
Blend + 0.1 wt% xGnP |
164.96 |
125.12 |
55.07 |
69.54 |
39.92 |
Blend + 0.5 wt% xGnP |
165.48 |
125.44 |
55.04 |
67.93 |
40.03 |
Blend + 1 wt% xGnP |
164.97 |
125.30 |
56.21 |
68.41 |
41.05 |
From Table 1, it can be seen that PP/PS–xGnP composites exhibit greater value of ΔHm and ΔHc, compared to neat PP. This indicates that xGnP plays an important role in accelerating the rate crystallization of the PP, particularly with 0.1 wt% of xGnP. The percentage crystallinity value of PP increased from 40.3 to 45.6 with the addition of 0.1 wt% of xGnP. From these results, it can be argued that a small fraction of graphene platelets can act as effective nucleating sites for the crystallization of PP. But, at higher concentrations of graphene the nucleation effect is reduced, indicating agglomeration of the xGnP. The nucleating effect of xGnP was observed in several other studies.28–31 However, no significant change in Tm and Tc was observed by the addition of xGnP. It is important to note that addition of xGnP in ternary systems has little effect on the crystallization behavior of PP. This can be correlated with the phase morphology of the ternary system. As mentioned earlier, the ternary system exhibits an interpenetrating, co-continuous phase structure and xGnP nanoplatelets prefer to be dispersed in the soft SEBS phase. Thus xGnP has no direct contact with PP and therefore no influence on the rate of crystallization.
Thermo gravimetric analysis
The thermal degradation properties are evaluated from TGA thermograms (Fig. 8). It has been reported that graphene is capable of increasing the thermal stability of PP29 and PS.32 However, TGA results revealed that nanofillers have only marginal effect on the thermal stability of the composites. All systems showed single step degradation mechanism. Thermal degradation of composites are summarized in Fig. 9, in terms of parameters like initial degradation temperature (IDT), maximum degradation temperature (MDT) and final degradation temperature (FDT). The IDT and MDT of the PP/PS–xGnP composites remained unaltered, but the FDT was slightly improved by the incorporation of xGnP. IDT and MDT values of ternary systems are greater than those of binary systems. This implies that the presence of SEBS in PP/PS blends has a sort of compatibilizing action; which stabilizes the phase morphology (as evidenced from SEM micrographs), improves the interfacial situation of the blends (as observed from mechanical and viscoelastic properties) retards the tendency of filler agglomeration and refines dispersion of graphene platelets in SEBS phase.
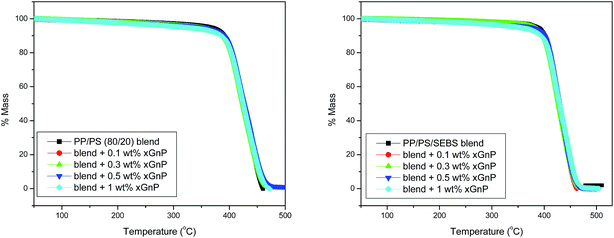 |
| Fig. 8 TGA curves of (a) neat PP/PS blend and PP/PS–xGnP composites (b) PP/PS–SEBS blends and PP/PS–SEBS–xGnP composites. | |
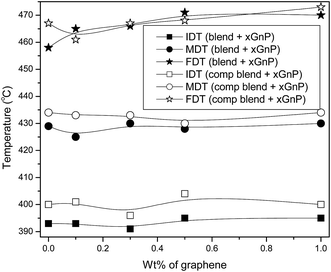 |
| Fig. 9 IDT, MDT and FTD of PP/PS, PP/PS–SEBS, PP/PS–xGnP and PP/PS–SEBS–xGnP systems. | |
Conclusion
Nanocomposites based on PP/PS blend and exfoliated graphene nanoplatelets (xGnP) were prepared with and without SEBS and studied the mechanical, viscoelastic, thermal degradation and crystallization properties and phase morphology. It was observed that graphene nanoplatelets have no reinforcement effect in PP/PS blends, as they are not well dispersed in the blend or blend interface and contributed nothing towards the reduction of the unfavourable cross-correlations between PP and PS chains in the blend, which was supported by phase morphology studies. However, addition of SEBS into the blends made a dramatic change. PP/PS–SEBS–xGnP (0.1 wt%) exhibited good impact strength (ca. 140% greater than PP/PS blends), excellent tensile elongation (ca. 500% greater than PP/PS blend), reasonably good strength (ca. 15% less than PP/PS blends) and stiffness (ca. 25% less than PP/PS blends). These results suggest that PP/PS–SEBS–xGnP (0.1 wt%) composite material is suitable for automotive applications. The incorporation of xGnP into this ternary system made significant impact as they diffused through the polymer chains, finely dispersed among soft SEBS segments and enhanced the overall stiffness of the system. The DMA results revealed an overall reduction in internal friction or improvement in damping behavior of the composites, in the presence of SEBS. It was also observed that graphene platelets at very low concentrations can act as effective nucleating sites for the crystallization of PP. The thermogravimetric analysis disclosed that the initial degradation temperature (IDT) and maximum degradation temperature (MDT) of composites increased in the presence of SEBS.
Acknowledgements
J P acknowledges the Department of Science and Technology, Government of India, for financial support under an Innovation in Science Pursuit for Inspired Research (INSPIRE) Faculty Award (contract grant number IFA-CH-16). N H acknowledges the funding from Australian Academy of Science under the Australia India Early Career Research Fellowship program.
References
- J. Li, H. Li, C. Wu, Y. Ke, D. Wang, Q. Li, L. Zhang and Y. Hu, Eur. Polym. J., 2009, 45, 2619–2628 CrossRef CAS PubMed.
- A. A. Adewole, A. Denicola, C. G. Gogos and L. Mascia, Adv. Polym. Technol., 2000, 19, 180–193 CrossRef CAS.
- S. S. Morye, Polym. Eng. Sci., 2005, 45, 1369–1376 CAS.
- S. S. Morye, Polym. Eng. Sci., 2005, 45, 1377–1384 CAS.
- J. Parameswaranpillai, G. Joseph, R. V. Chellappan, A. K. Zachakariah and N. Hameed, J. Polym. Res., 2015, 22, 2, DOI:10.1007/s10965-014-0641-y.
- W. R. Waldman and M. De Paoli, Polym. Degrad. Stab., 2008, 93, 273–280 CrossRef CAS PubMed.
- X. Zhao, Y. Huang, M. Kong, Q. Yang and G. Li, RSC Adv., 2014, 4, 59302–59309 RSC.
- N. Wang, N. Gao, Q. Fang and E. Chen, Mater. Des., 2011, 32, 1222–1228 CrossRef CAS PubMed.
- M. F. Díaz, S. E. Barbosa and N. J. Capiati, Polymer, 2005, 46, 6096–6101 CrossRef PubMed.
- L. Caporaso, N. Iudici and L. Oliva, Macromolecules, 2005, 38, 4894–4900 CrossRef CAS.
- A. K. Gupta and S. N. Purwar, J. Appl. Polym. Sci., 1985, 30, 1799–1814 CrossRef CAS PubMed.
- P. H. P. Macaubas and N. R. Demarquette, Polymer, 2001, 42, 2543–2554 CrossRef CAS.
- A. Halimatudahliana, H. Ismail and M. Nasir, Polym. Test., 2002, 21, 263–267 CrossRef CAS.
- W. Brostow, T. H. Grguric, O. Olea-Mejia, D. Pietkiewicz and V. Rek, e-Polymers, 2008, 034 Search PubMed.
- G. P. Kar, S. Biswas and S. Bose, Phys. Chem. Chem. Phys., 2015, 17, 1811–1821 RSC.
- Y. Cao, J. Zhang, J. Feng and P. Wu, ACS Nano, 2011, 5, 5920–5927 CrossRef CAS PubMed.
- R. Bahrami, T. I. Löbling, A. H. Gröschel, H. Schmalz, A. H. E. Müller and V. Altstädt, ACS Nano, 2014, 8, 10048–10056 CrossRef CAS PubMed.
- S. Ye, Y. Cao, J. Feng and P. Wu, RSC Adv., 2013, 3, 7987–7995 RSC.
- Y. Cao, J. Feng and P. Wu, J. Mater. Chem., 2012, 22, 14997–15005 RSC.
- F. You, D. Wang, X. Li, M. Liu, Z. M. Dang and G. H. Hu, J. Appl. Polym. Sci., 2014, 131, 40455, DOI:10.1002/app.40455.
- A. K. Krishnan, T. S. George, R. Anjana, N. Joseph and K. E. George, J. Appl. Polym. Sci., 2013, 127, 1409–1415 CrossRef CAS PubMed.
- L. Elias, F. Fenouillot, J. C. Majeste and P. Cassagnau, Polymer, 2007, 48, 6029–6040 CrossRef CAS PubMed.
- M. H. Al-Saleh and U. Sundararaj, Composites, Part A, 2008, 39, 284–293 CrossRef PubMed.
- R. R. Tiwari and D. R. Paul, Polymer, 2011, 52, 1141–1154 CrossRef CAS PubMed.
-
J. Parameswaranpillai, G. Joseph, K. P. Shinu, P. R. Sreejesh, S. Jose, N. V. Salim and N. Hameed, under review.
- M. A. Milani, D. González, R. Quijada, N. R. S. Basso, M. L. Cerrada, D. S. Azambuja and G. B. Galland, Compos. Sci. Technol., 2013, 84, 1–7 CrossRef CAS PubMed.
- K. N. Indira, P. Jyotishkumar and S. Thomas, Fibers Polym., 2014, 15, 91–100 CrossRef CAS PubMed.
- S. Zhao, F. Chen, C. Zhao, Y. Huang, J. Dong and C. C. Han, Polymer, 2013, 54, 3680–3690 CrossRef CAS PubMed.
- P. Song, Z. Cao, Y. Cai, L. Zhao, Z. Fang and S. Fu, Polymer, 2011, 52, 4001–4010 CrossRef CAS PubMed.
- M. El Achaby, F. Z. Arrakhiz, S. Vaudreuil, E. M. Essassi, A. Qaiss and M. Bousmina, J. Appl. Polym. Sci., 2013, 127, 4697–4707 CrossRef CAS PubMed.
- C. Vallés, A. M. Abdelkader, R. J. Young and I. A. Kinloch, Faraday Discuss., 2014, 173, 379–390 Search PubMed.
- A. S. Patole, S. P. Patole, H. Kang, J. B. Yoo, T. H. Kim and J. H. Ahn, J. Colloid Interface Sci., 2010, 350, 530–537 CrossRef CAS PubMed.
|
This journal is © The Royal Society of Chemistry 2015 |
Click here to see how this site uses Cookies. View our privacy policy here.