DOI:
10.1039/C4RA16634E
(Paper)
RSC Adv., 2015,
5, 31303-31310
Continuous cyclo-polymerisation of L-lactide by reactive extrusion using atoxic metal-based catalysts: easy access to well-defined polylactide macrocycles†
Received
18th December 2014
, Accepted 24th March 2015
First published on 24th March 2015
Abstract
The bulk polymerisation of L-lactide was assessed using the alkaline-earth and lanthanide borohydride complexes Mg(BH4)2 (1), Ca(BH4)2(THF)2 (2) and Ln(BH4)3(THF)3 with Ln = Nd (3), Sm (4) and La (5). All five catalysts display good activities, with up to 77% conversion in 20 min with 5. Lanthanide-based catalysts 3, 4 and 5, which were found to lead to the highest conversions, were tested in reactive extrusion polymerisation conditions in a twin-screw micro-extruder. High activities were also observed in theses experimental conditions, affording up to 73% conversion in only 20 min with lanthanum-based catalyst 5. More interestingly, the polylactides formed display a macrocyclic structure with molecular weights up to 30
000 g mol−1 along with relatively narrow dispersities (1.23–1.79). The macrocycles are formed via intramolecular transesterification reactions taking place during the ring opening polymerisation. Furthermore, the reactive extrusion polymerisations operate at a temperature as low as 130 °C, this monomer usually being polymerized at 185 °C. This is the first example of a cyclic polyester obtained directly via a continuous process.
Introduction
Cyclic polymers attract much attention these days, due to their unique physical properties.1 The cyclic topology and absence of chain ends confer cyclic polymer materials properties that are substantially different to those of their linear analogs, including increased glass transition temperatures (Tg), smaller hydrodynamic volumes and lower intrinsic viscosities.2 Among them, cyclic aliphatic polyesters, in particular polylactides (PLA) and polycaprolactones (PCL), are of high interest as many exhibit both biodegradability and biocompatibility.3 The cyclic topology imparts unique biophysical properties which may provide advantages for a range of biomedical applications, such as novel drug delivery vectors4 or supramolecular hosts.5 Moreover, both the mass and hydrodynamic volumes are substantially reduced compared to their linear counterparts, providing a retarded hydrolytic degradation profile.6 Despite several routes have been reported for the synthesis of cyclic polymers, obtaining well-defined macrocycles of high molecular weights is still a challenge.3 The most common strategy for their preparation is the ring-closure (RC) technique,2,3 which relies on the coupling of linear chains by a bifunctional agent2 or by reactive hetero end groups.7 The main drawback of this technique is the need of a highly diluted medium, in order to favour intramolecular cyclization rather than intermolecular reaction. A more convenient route for the synthesis of high molecular weight macrocycles is the ring-expansion (RE) technique, involving cyclic tin8 or aluminium-based catalysts.9 Finally, well-defined cyclic oligomers or polymers can be obtained through zwitterionic polymerisation, however with the requirement of low monomer to initiator ratios and the use of an aprotic trapping agent for the organocatalyst to terminate the reaction.10 The RE and zwitterionic polymerisation techniques both proceed through Ring Opening Polymerisation (ROP), via coordination-insertion or monomer-activated mechanisms respectively.11 Coordination-insertion ROP involving metal-based catalysts is a powerful and versatile technique, allowing access to well-defined high molecular weight polymers along with controlled microstructures by tuning the catalysts properties with appropriate ligands.11,12 In particular, lanthanide-based catalysts have shown to be highly active and stereoselective initiators for the ROP of lactides and lactones.13,14 Among them, borohydrido lanthanide complexes,14 such as trisborohydrides Ln(BH4)3(THF)x,14c–e were reported to be efficient for the solution polymerisation of L- and rac-lactide and ε-caprolactone in solvents such as THF or toluene. In the search of greener processes, solvent-free (bulk) polymerisation has been widely investigated for these monomers with other classes of initiators.15 In this context, reactive extrusion, which allows the direct polymerisation of the monomer in a twin-screw extruder through a continuous process, is of particular relevance.16 Successful results were recently reported for the ROP of L- and D,L-lactide with tinII octanoate as the catalyst, allowing the formation of stereocomplexes of PLA in the latter case and displaying superior thermal and mechanical properties compared to standard PLA's.16d Herein, the first example of the ring-opening cyclo-polymerisation of a cyclic ester, via intramolecular transesterification reactions, initiated by low toxicity metal-based catalysts17 in reactive extrusion conditions (Scheme 1) is reported. PLA macrocycles of high molecular weights and relatively narrow dispersities are obtained via a continuous process, making it suitable for potential high scale industrial development.
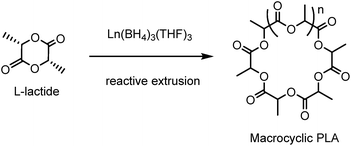 |
| Scheme 1 Macrocyclic polylactide obtained by reactive extrusion. | |
Results and discussion
Bulk polymerisations
The activity of borohydride catalysts toward the bulk polymerisation of L-lactide (L-LA) was first assessed using Schlenk techniques prior to transposition to the micro-extruder (Table 1). In addition to lanthanide borohydride complexes, the screening of their group II analogs (Ca and Mg), which have recently shown to be a promising class of initiators for the ROP of lactides, was performed.18 Five complexes were evaluated: the commercially available Mg(BH4)2 1 and Ca(BH4)2(THF)2 2, as well as Ln(BH4)3(THF)3 (Ln = Nd 3, Sm 4, La 5) which are easily accessible in one step synthesis by reacting the commercial LnCl3 with an excess of NaBH4.19 The bulk polymerisation reactions were first conducted in the experimental conditions reported for the polymerisation of L-lactide by reactive extrusion using tin(II) octanoate,16c i.e. at 185 °C and reaction times inferior to 30 min. All five catalysts were observed to be active in these experimental conditions. Mg-based catalyst 1 was found to be twice more active than its Ca analog 2 (run 1 vs. 4), as already observed with borohydrido alkaline-earth complexes as catalysts for the polymerisation of rac-lactide and ε-caprolactone.18b The lanthanide-based catalysts 3, 4 and 5 display good activities, affording 76, 70 and 56% conversion respectively in only 20 min (runs 7, 10 and 13), along with Mn up to 13
920 g mol−1 (run 7). All catalysts led to relatively narrow dispersities ranging from 1.25 to 1.60. The experimental molecular weights were found to be inferior to the calculated ones for two (runs 1–6) or three (runs 7–15) growing chains per metal centre, which can be attributed to the occurrence of intramolecular transesterification reactions during the polymerisation. These five catalysts were then tested in bulk polymerisation at lower temperatures. At 150 °C, catalysts 1 to 5 led to conversions relatively close to those observed at 185 °C (runs 2, 5, 8, 11 and 14) whereas the conversion obtained with 5 increases up to 65%, vs. 56% at 185 °C (run 13 vs. 14), affording polylactide with a higher molecular weight of 25
360 g mol−1 (run 14). At 130 °C, magnesium-based catalyst 1 was nearly inactive (run 3) in contrast to 2, 3 and 4, the latter ones leading to conversions close to those obtained at 150 °C (runs 6, 9 and 12). In these experimental conditions, catalyst 5 led to the highest conversion of 77% in 20 min (run 15). In the case of lanthanide-based catalysts 3, 4 and 5, it is noteworthy that the highest Mn values were observed at 150 °C in all cases, this temperature being the best compromise between good conversions and the occurrence of less transesterification reactions.
Table 1 Bulk polymerisation of L-LA using Mg(BH4)2 (1), Ca(BH4)2(THF)2 (2) or Ln(BH4)3(THF)3; Ln = Nd (3), Sm (4) or La (5)a
Run |
Catalyst |
T (°C) |
[L-LA]/[M] |
Time (min) |
Conversionb (%) |
Mn SECc (g mol−1) |
Mn calculatedd (g mol−1) |
Đc |
Tge (°C) |
Tme (°C) |
m(L-LA) = 2 g; M = metal; n(M) = 2.77 × 10−5 mol for [L-LA]0/[M]0 = 500 or 1.39 × 10−5 mol for [L-LA]0/[M]0 = 1000. Determined by 1H NMR in CDCl3. Absolute molecular weight and molecular weight distribution (Đ = Mw/Mn) determined by SEC using multi-angle light scattering detector in THF at 40 °C. Expected Mn values for two (runs 1–6) and three (runs 7–15) growing chains per metal centre, Mn = {[L-LA]0/[M]0 × M(L-LA) × conversion}/(number of growing chains). Determined by DSC. |
1 |
1 |
185 |
500 |
30 |
60 |
10 360 |
21 620 |
1.25 |
54.1 |
146.4; 151.9 |
2 |
1 |
150 |
500 |
30 |
57 |
9420 |
20 540 |
1.52 |
52.2 |
151.6; 157.2 |
3 |
1 |
130 |
500 |
80 |
6 |
— |
— |
— |
— |
— |
4 |
2 |
185 |
500 |
30 |
28 |
4700 |
10 090 |
1.49 |
51.4 |
140.3; 148.1 |
5 |
2 |
150 |
500 |
30 |
33 |
7750 |
11 890 |
1.20 |
46.9 |
133.8; 144.4 |
6 |
2 |
130 |
500 |
60 |
36 |
10 430 |
12 970 |
1.19 |
52.8 |
143.4; 150.9 |
7 |
3 |
185 |
1000 |
20 |
76 |
13 920 |
36 515 |
1.35 |
57.9 |
147.4; 153.8 |
8 |
3 |
150 |
1000 |
20 |
72 |
16 840 |
34 590 |
1.30 |
59.7 |
152.9; 159.8 |
9 |
3 |
130 |
1000 |
20 |
72 |
13 070 |
34 590 |
1.38 |
58.1 |
150.9; 158.6 |
10 |
4 |
185 |
1000 |
20 |
70 |
13 800 |
33 630 |
1.30 |
57.6 |
145.2 |
11 |
4 |
150 |
1000 |
20 |
65 |
18 350 |
31 230 |
1.24 |
58.8 |
149.1; 153.9 |
12 |
4 |
130 |
1000 |
20 |
65 |
12 480 |
31 230 |
1.79 |
57.8 |
145.9; 153.4 |
13 |
5 |
185 |
1000 |
20 |
56 |
13 510 |
26 910 |
1.60 |
56.5 |
151.8; 158.9 |
14 |
5 |
150 |
1000 |
20 |
65 |
25 360 |
31 230 |
1.29 |
53.2 |
155.7; 163.9 |
15 |
5 |
130 |
1000 |
20 |
77 |
18 220 |
36 995 |
1.62 |
60.8 |
157.6; 165.0 |
The PLA's synthesized herein display glass transition temperatures (Tg) ranging from 46.9 to 60.8 °C (see further comments in the paragraph “structural characterization of the polylactides”). Regarding melting temperatures (Tm) a double melting peak is observed in most cases. This observation has been reported previously and assigned to a phase transformation phenomenon.16b,20
Reactive extrusion polymerisations
Following the bulk polymerisation preliminary tests, catalysts 2, 3, 4 and 5 were selected for further experiments in a twin-screw micro-extruder (Table 2). The reactive extrusion polymerisation reactions were performed under nitrogen flow with 20 grams of L-LA and a shear rate of 100 rpm. The first experiments were conducted at 185 °C. In these conditions, calcium-based catalyst 2 was found to be poorly active (less than 10% conversion in 60 min using 500 equivalents of L-LA, not reported in Table 2). Regarding lanthanide trisborohydrides 3, 4 and 5, they still display high activities (runs 16, 22 and 28), affording up to 71% conversion in only 20 min with 5 (run 28). One can note that in general the conversions were found slightly lower than those observed in bulk polymerisation conditions, what can be attributed to a higher level of oxygen and water in the nitrogen atmosphere of the extruder compare to that of the glove box used for the bulk experiments (O2 = 1 ppm and H2O = 0 ppm). Moreover, the catalysts were exposed to air very briefly when introduced in the extruder which was not the case in the bulk experiments. PLA's synthesized in reactive extrusion conditions, at 185 °C with a reaction time of 20 min, display average molecular weight values up to 20
750 g mol−1 (run 22) and relatively narrow dispersities (1.35–1.44), the latter ones being much lower than those obtained when tin octanoate was used as the catalyst in the same process (Đ = 1.9–2.9).16c At longer reaction times (80 min), high conversions of 77, 79 and 79% were observed for catalysts 3, 4 and 5 respectively (runs 17, 23 and 29), with Mn up to 24
180 g mol−1 (run 17) and similar dispersities (1.27–1.45).
Table 2 Polymerisation of L-LA by reactive extrusion using Ln(BH4)3(THF)3; Ln = Nd (3), Sm (4) or La (5)a
Run |
Catalyst |
T (°C) |
Time (min) |
Conversionb (%) |
Mn SECc (g mol−1) |
Mn calculatedd (g mol−1) |
Đc |
Tge (°C) |
Tme (°C) |
m(L-LA) = 20 g, [L-LA]0/[Ln]0 = 1000, n(Ln) = 1.39 × 10−4 mol, rotation = 100 rpm under nitrogen. Determined by 1H NMR in CDCl3. Absolute molecular weight and molecular weight distribution (Đ = Mw/Mn) determined by SEC using multi-angle light scattering detector in THF at 40 °C. Expected Mn values for three growing chain per metal centre, Mn = {[L-LA]0/[Ln]0 × M(L-LA) × conversion}/3. Determined by DSC. |
16 |
3 |
185 |
20 |
59 |
17 700 |
28 350 |
1.44 |
56.7 |
155.5; 164.5 |
17 |
3 |
185 |
80 |
77 |
24 180 |
36 995 |
1.35 |
56.2 |
154.6; 163.3 |
18 |
3 |
150 |
20 |
56 |
23 500 |
26 910 |
1.37 |
57.4 |
159.9; 167.0 |
19 |
3 |
150 |
80 |
69 |
23 460 |
33 150 |
1.31 |
54.1 |
166.3 |
20 |
3 |
130 |
20 |
54 |
16 770 |
25 945 |
1.23 |
55.6 |
155.0; 162.3 |
21 |
3 |
130 |
80 |
83 |
18 760 |
31 580 |
1.35 |
46.8 |
144.1; 155.2 |
22 |
4 |
185 |
20 |
54 |
20 750 |
25 945 |
1.35 |
53.3 |
150.6 |
23 |
4 |
185 |
80 |
79 |
23 450 |
37 960 |
1.27 |
51.4 |
151.5 |
24 |
4 |
150 |
20 |
59 |
19 840 |
28 350 |
1.30 |
52.1 |
154.9 |
25 |
4 |
150 |
80 |
76 |
26 360 |
36 515 |
1.24 |
54.6 |
153.3 |
26 |
4 |
130 |
20 |
56 |
18 560 |
26 910 |
1.31 |
45.0 |
147.2 |
27 |
4 |
130 |
80 |
76 |
23 900 |
36 515 |
1.26 |
52.4 |
153.2 |
28 |
5 |
185 |
20 |
71 |
18 750 |
34 110 |
1.40 |
52.0 |
155.0; 161.0 |
29 |
5 |
185 |
80 |
79 |
18 750 |
37 960 |
1.45 |
55.8 |
158.4 |
30 |
5 |
150 |
20 |
73 |
17 270 |
35 070 |
1.47 |
56.4 |
159.0; 166.4 |
31 |
5 |
150 |
80 |
82 |
16 260 |
39 400 |
1.79 |
51.8 |
157.8; 167.5 |
32 |
5 |
130 |
20 |
67 |
18 850 |
32 190 |
1.36 |
57.7 |
161.3; 168.0 |
33 |
5 |
130 |
80 |
81 |
30 300 |
38 920 |
1.29 |
53.3 |
158.2; 166.4 |
Further reactive extrusion experiments were then conducted with lanthanide complexes. Actually, an orange coloration of the polymer was observed when the reaction was performed at 185 °C. This was attributed to partial decomposition of the catalyst at high temperature. As it was previously verified that the lanthanide complexes 3, 4 and 5 were active for the bulk polymerisation of L-lactide at lower temperatures (Table 1), reactive extrusion reactions at 150 and 130 °C were also performed. In these experimental conditions, all three catalysts still display high activities and polylactide with molecular weights as high as 30
300 g mol−1 could be produced in the case of lanthanum-based catalyst 5 when the reaction was performed at 130 °C (run 33). More interestingly the PLA's obtained at 130 and 150 °C are transparent and slightly yellow-colored, as observed with tin octanoate as the catalyst, showing that less thermal degradation of our catalysts occurs at these temperatures (Fig. 1).
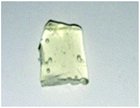 |
| Fig. 1 Crude PLA material obtained by reactive extrusion polymerisation à 130 °C. | |
Following these results, some experiments were conducted at 150 °C with a much higher monomer to catalyst ratio of 2500 equivalents of L-Lactide vs. 3, 4 and 5 (runs 34–39, Table 3). In these conditions, all catalysts are still active and form materials with molecular weights and dispersities in the same range as previous runs (up to 25
330 g mol−1, Đ = 1.24–1.55), though with lower conversions.
Table 3 Polymerisation of L-LA by reactive extrusion at 150 °C using Ln(BH4)3(THF)3; Ln = Nd (3), Sm (4) or La (5) with [L-LA]/[Ln] = 2500a
Run |
Catalyst |
Time (min) |
Conversionb (%) |
Mn SECc (g mol−1) |
Mn calculatedd (g mol−1) |
Đc |
Tge (°C) |
Tme (°C) |
m(L-LA) = 20 g, [L-LA]0/[Ln]0 = 2500, T = 150 °C, n(Ln) = 5.55 × 10−5 mol, rotation = 100 rpm under nitrogen. Determined by 1H NMR in CDCl3. Absolute molecular weight and molecular weight distribution (Đ = Mw/Mn) determined by SEC using multi-angle light scattering detector in THF at 40 °C. Expected Mn values for three growing chain per metal centre, Mn = {[L-LA]0/[Ln]0 × M(L-LA) × conversion}/3. Determined by DSC. |
34 |
3 |
20 |
38 |
21 050 |
45 645 |
1.24 |
57.6 |
156.4; 164.0 |
35 |
3 |
80 |
50 |
22 580 |
60 060 |
1.37 |
57.4 |
166.2 |
36 |
4 |
20 |
42 |
18 030 |
50 450 |
1.55 |
54.6 |
153.3 |
37 |
4 |
80 |
61 |
25 330 |
73 270 |
1.42 |
59.0 |
157.1; 164.0 |
38 |
5 |
20 |
32 |
12 620 |
38 440 |
1.54 |
59.1 |
164.2 |
39 |
5 |
80 |
42 |
20 040 |
50 450 |
1.31 |
61.4 |
167.6 |
Structural characterization of the polylactides
The structure of the obtained polylactides was then determined. It is noteworthy that the ROP of lactides with borohydride initiators, can lead to either linear α,ω-dihydroxy telechelic H[OCH(Me)CO](n−1)OCH(Me)CH2OH or mixed hydroxyl-aldehyde-terminated H[OCH(Me)CO]nH polymers, both displaying 1H NMR signals for the terminated-side end group at δ = 4.33 ppm for HOCHMe and δ = 1.47 ppm for HOCHMe in CDCl3,14d–j or to cyclic structures.14g Looking at the 1H NMR spectra of the PLA's synthesized by reactive-extrusion, no end-group could be observed for polymers synthesized with 3 and 4 (Fig. 2 and ESI 1 and 2†).21 The samples were then analyzed by matrix-assisted laser desorption-ionization time-of-flight (MALDI-TOF) mass spectrometry. The mass spectra display in all cases signals relative to sodium complexed cyclic PLA's (Fig. 3 and ESI 3 and 4†).21 The formation of macrocycles is consistent with the fact that Mn values did not increase linearly vs. conversion and are inferior to the calculated values for three growing chains per metal centre. The formation of these PLA macrocycles results from intramolecular transesterification reactions (back-biting), i.e the reaction of an ester group on the growing chain with the Ln-OR active bond (Scheme 2). Indeed, MALDI-TOF-MS analysis showed distributions containing both even-membered and odd-membered oligomers, with peaks separated by 72 Da, unambiguously establishing that transesterification processes take place. These secondary reactions seem to occur when the chain reaches a critical length, preventing the molecular mass to increase along with conversion. Intramolecular transesterification reactions were already observed during the ROP of cyclic esters via coordination-insertion mechanism involving metal-based complexes, however the cycles usually represent only a fraction of the population along with a significantly larger percentage of linear macromolecules.13e,14g,22 In the particular case of lanthanide trisborohydride complexes, the ROP of L- and rac-lactide in THF was previously studied and the authors reported the formation of linear dihydroxy telechelic polylactides.14d,e In order to assess if the formation of the cycles was linked to the absence of solvent or to the reactive extrusion process itself, PLA's synthesized by bulk polymerisation were also analyzed by MALDI-TOF mass spectrometry (Fig. 4 and ESI 5–7†). Spectra of PLA's obtained using catalysts 3, 4 and 5 (runs 7, 10, 13 and 14) all display a main population of cyclic polymer along with a small secondary linear fraction. The structure of the linear polymer was attributed to H-PLA-OH, which could result from the partial hydrolysis of the cycles.13e,22 Actually, in the case of the bulk polymerisation reactions, the resulting reaction mixtures were treated with non-anhydrous solvents in order to isolate the polymer (see Experimental section), whereas the materials synthesized in the extruder did not received any further treatment. One can conclude that, with the lanthanide trisborohydride catalysts, the absence of solvent in the reaction medium dramatically favors the intramolecular transesterification reactions, leading to the formation of macrocycles exclusively.
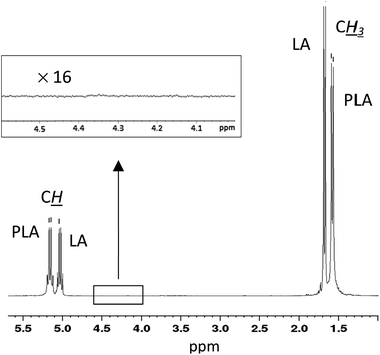 |
| Fig. 2 1H NMR spectrum (CDCl3) of the crude PLA synthesized by reactive extrusion polymerisation (run 18). | |
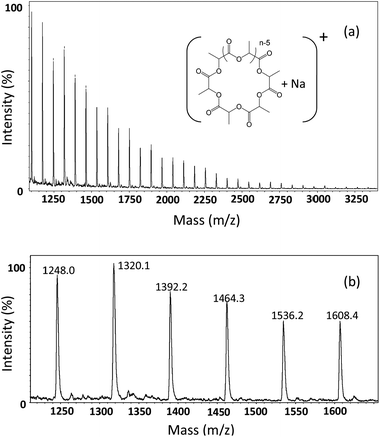 |
| Fig. 3 (a) MALDI-TOF mass spectrum of macrocyclic PLA synthesized by reactive extrusion (run 26) (b) expansion of the spectrum in the range m/z = 1200–1650 amu. Mass = (n × 72.07) + 22.99 corresponding to cyclic PLA. | |
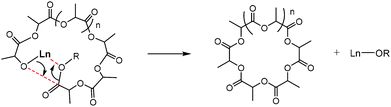 |
| Scheme 2 Intramolecular transesterification leading to macrocyclic PLA. | |
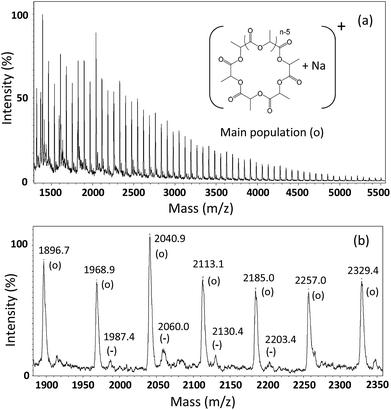 |
| Fig. 4 (a) MALDI-TOF mass spectrum of PLA synthesized by standard bulk polymerisation (run 10) (b) expansion of the spectrum in the range m/z = 1880–2360 amu. Main population corresponds to cyclic PLA, mass = (n × 72.07) + 22.99 (o). Small fraction of linear PLA (H-PLA-OH), mass = (n × 72.07) + 18 + 22.99 (-). | |
Regarding the glass transition temperatures (Tg), the polymers obtained in bulk conditions with catalysts 3, 4 and 5 generally display higher Tg than that reported for polylactide synthesized by reactive extrusion with tin octanaote (Tg = 56 °C for Mn = 75
000 g mol−1),16c even if the Mn values were found inferior (12
480 to 25
360 g mol−1). This observation is in agreement with the cyclic structure of these polymers, as it is known that the materials displaying such architecture exhibit higher Tg values compare to their linear analogs.2 In the case of polylactides synthesized by reactive extrusion, Tg values were sometimes found lower as the thermal analysis were performed on the raw materials containing some residual L-lactide monomer which acts as an internal plasticizer.
Conclusions
In summary, the efficiency of alkaline-earth and lanthanide borohydride complexes, Mg(BH4)2, Ca(BH4)2(THF)2 and Ln(BH4)3(THF)3 (Ln = Nd, Sm and La), as initiators for the bulk polymerisation of L-lactide has been reported. All five catalysts display good activities, the latter systems displaying the highest activities, affording up to 77% conversion in 20 min in the case of the lanthanum-based complex. Regarding the reactive extrusion reactions conducted in a twin-screw micro-extruder, lanthanide trisborohydrides exhibit activities relatively close to those observed in bulk polymerisation conditions (up to 73% conversion in 20 min with lanthanum catalyst). It has to be highlighted that the polylactides synthesized in these experimental conditions display almost exclusively cyclic architectures, arising from intramolecular transesterification reactions taking place during the ring opening polymerisation. The polylactide macrocycles exhibit molecular weights up to 30
000 g mol−1 and relatively narrow dispersities for this kind of process (1.23–1.79). It has also been demonstrated that with lanthanide borohydrides as the catalysts, the reaction can proceed at a temperature as low as 130 °C, the reactive extrusion polymerisation of this monomer being usually conducted at 185 °C.16a–e It has to be pointed out that (i) this is the first example of macrocyclic polyesters synthesized directly via a continuous process, (ii) this would allow high scale industrial development of macrocyclic polyesters. This discovery is also significant as cyclic polylactides are materials of high interest due to their potential biomedical applications such as novel drug delivery vectors4 or supramolecular hosts.5 Finally, the metals involved in the production of these PLA macrocycles display low-toxicities, what is appropriate in such a context. The scale-up of the reaction on a high-scale extruder is in progress.
Experimental
General procedures
L-lactide (L-LA) was supplied by Purac, conditioned in small sealed bags under vacuum and used as received (H2O content, measured by Karl-Fischer analysis, was found inferior to 5 ppm). The sealed bags were open just before mixing L-lactide with the catalyst. Mg(BH4)2 and Ca(BH4)2(THF)2 were purchased from Aldrich and used as received. Ln(BH4)3(THF)3 (Ln = La, Nd, Sm) were synthesized according to the literature procedure.19
NMR analysis
1H NMR spectra of PLA were recorded on a Bruker Avance 300 MHz instrument at 300 K in CDCl3. The chemical shifts were calibrated using the residual resonances of the solvent.
SEC measurements
The number-average molar masses (Mn) and dispersities (Đ = Mw/Mn) of the polylactides were determined by size exclusion chromatography (SEC) in THF at 40 °C (1 mL min−1) with a triple detection system, equipped with an Alliance Waters e2695, a multiangle light scattering detector (MALS, Wyatt Technology mini DAWN TREOS), and a refractive index detector (Waters 2414). The SEC system was equipped with three Waters Styragel (HT1, HT3 and HT4) columns. The MALS method was used to determine the absolute molecular weights of the PLAs. The differential refractive index (DRI) increment (dn/dc) value was determined for our macrocyclic PLAs and found to be 0.0478 mL g−1 in THF at 40 °C. Samples were prepared by dissolving the product (∼10 mg) in 4 mL of THF. The solutions were then filtered with 0.45 μm filters.
MALDI-TOF mass spectroscopy measurements
Mass spectra were recorded by matrix-assisted laser desorption and ionization time-of-flight (MALDI-TOF) mass spectrometry using a Bruker autoflex III smartbeam mass spectrometer, equipped with the laser that produces pulses at 337 nm using dithranol as a matrix and NaI as cationizing agent. Spectra were recorded in linear mode at an accelerating potential of 20 kV. Samples were prepared by dissolving the polymer in THF at a concentration of 2–5 mg mL−1. A 10 μL aliquot of this solution was mixed with 20 μL of matrix solution and 10 μL of NaI solution (both at 20 mg mL−1 in THF). Standards (polystyrenes of known structure, purchased from Polymer Standards Service) were used to calibrate the mass scale.
DSC analysis
Thermal analysis were carried out by Differential Scanning Calorimetry (DSC) measurements using a TA Instruments Q100 apparatus, in a temperature range between 20 °C and 250 °C. A representative DSC experimental procedure is as follows. A small amount of material (∼5 mg) was loaded onto an aluminum pan with a cover. In the DSC experiment, the sample was heated from the ambient temperature to 250 °C at a rate of 10°C min−1, whereupon the temperature was held for 10 min to remove all thermal history. The sample was then cooled to 20 °C at a rate of 10 °C min−1, where the temperature was held for another 10 min before heating again to 250 °C at a rate of 10 °C min−1. The glass transition temperature (Tg) was determined from the second heating scan by a mathematical averaging method. Straight lines were fit tangent to the heat flow curve before and after the transition. From the average of the slopes and intercepts of these two lines, a third line was obtained and Tg was taken as the temperature at which the heat flow curve intersected this third line. The melting temperatures (Tm) were also determined from the second heating scan and taken as the temperatures corresponding to the melting peak maximum. The measurements were conducted using indium and zinc as standards for calibration.
Typical procedure for bulk polymerisations
L-lactide (2 g) and the catalyst (2.77 × 10−5 mol for [L-LA]0/[M]0 = 500 or 1.39 × 10−5 mol for [L-LA]0/[Ln]0 = 1000) were weighted and mixed together in a Schlenk in a glove box. The vessel was heated at 130, 150 or 185 °C under stirring for a given time. At the end of the reaction, the highly viscous medium was dissolved in non-anhydrous chloroform. An aliquot of the obtained solution was taken and dried under vacuum in order to determine the conversion by 1H NMR measurements in CDCl3. The PLA in solution in chloroform was then poured in methanol, filtered and dried under vacuum before being analyzed by SEC, DSC and MALDI-TOF MS.
Typical procedure for reactive extrusion experiments
The L-lactide (20 g) and the catalyst (1.39 × 10−4 mol for [L-LA]0/[Ln]0 = 1000 or 5.55 × 10−5 mol for [L-LA]0/[Ln]0 = 2500) were mixed before feeding the extruder with a manual stirring. The polymerisation was performed under nitrogen in a small-scale co-rotating twin screw extruder, developed by DSM N.V. (Geleen, the Netherlands) with a screw length of 12 cm and a capacity of 20 g. The temperature of the micro-extruder was set from 130 to 185 °C and a shear rate of 100 rpm was used. The polymerisation was followed by taking aliquots of the reaction and the conversion was determined by integration of the signals relative to L-LA and PLA on the 1H NMR spectrum.
Acknowledgements
The authors would like to thank Antoine Gallos and Pierre Bachelet for reactive extrusion experiments, Aurélie Malfait for SEC analysis, Andréa Messina for DSC analysis, Nicholas Stanley for careful reading of the manuscript, CNRS and Région Nord Pas de Calais for fundings.
References
- Z. Jia and M. J. Monteiro, J. Polym. Sci., Part A: Polym. Chem., 2012, 50, 2085 CrossRef CAS.
- J. Roovers, in Cyclic Polymers, ed. J. A. Semlyen, Kluwer, Dordrecht, 2nd edn, 2000, pp. 34–383 Search PubMed.
-
(a) J. N. Hoskins and S. M. Grayson, Polym. Chem., 2011, 2, 289 RSC;
(b) H. R. Kricheldorf, J. Polym. Sci., Part A: Polym. Chem., 2010, 48, 251 CrossRef CAS.
-
(a) N. Nasongkla, B. Chen, N. Macaraeg, M. E. Fox, J. M. J. Fréchet and F. C. Szoka, J. Am. Chem. Soc., 2009, 131, 3842 CrossRef CAS PubMed;
(b) B. Chen, K. Jerger, J. M. J. Fréchet and F. C. Szoka, J. Controlled Release, 2009, 140, 203 CrossRef CAS PubMed.
- M. H. Chisholm, J. C. Callucci and H. Yin, Proc. Natl. Acad. Sci. U. S. A., 2006, 103, 15315 CrossRef CAS PubMed.
- J. N. Hoskins and S. M. Grayson, Macromolecules, 2009, 42, 6406 CrossRef CAS.
- L. Rique-Lurbert, M. Schappacher and A. Deffieux, Macromolecules, 1994, 27, 6318 CrossRef.
-
(a) S. Roelens, A. D. Cort and L. Mandolini, J. Org. Chem., 1992, 57, 1472 CrossRef CAS;
(b) H. R. Kricheldorf and S.-R. Lee, Macromolecules, 1995, 28, 6718 CrossRef CAS;
(c) H. R. Kricheldorf and G. Schwarz, Macromol. Rapid Commun., 2003, 24, 359 CrossRef CAS;
(d) M. Ryner, A. Finne, A.-C. Albertsson and H. R. Kricheldorf, Macromolecules, 2001, 34, 7281 CrossRef CAS;
(e) H. Li, A. Debuigne, R. Jérôme and P. Lecomte, Angew. Chem., Int. Ed., 2006, 45, 2264 CrossRef CAS PubMed.
-
(a) J. Weil, R. T. Mathers and Y. D. Y. L. Getzler, Macromolecules, 2012, 45, 1118 CrossRef CAS;
(b) J. A. Castro-Osma, C. Alonso-Moreno, J. C. Garcia-Martinez, J. Fernandez-Baeza, L. F. Sanchez-Barba, A. Lara-Sanchez and A. Otero, Macromolecules, 2013, 46, 6388 CrossRef CAS.
-
(a) F. Nederberg, E. F. Connor, M. Möller, T. Glauser and J. L. Hedrick, Angew. Chem., Int. Ed., 2001, 40, 2712 CrossRef CAS;
(b) D. A. Culkin, W. Jeong, S. Csihony, E. D. Gomez, N. R. Balsara, J. L. Hedrick and R. M. Waymouth, Angew. Chem., Int. Ed., 2007, 46, 2627 CrossRef CAS PubMed;
(c) W. Jeong, E. J. Shin, D. A. Culkin, J. L. Hedrick and R. M. Waymouth, J. Am. Chem. Soc., 2009, 131, 4884 CrossRef CAS PubMed;
(d) E. J. Shin, H. A. Brown, S. Gonzalez, W. Jeong, J. L. Hedrick and R. M. Waymouth, Angew. Chem., Int. Ed., 2011, 50, 6388 CrossRef CAS PubMed;
(e) E. Piedra-Arroni, C. Ladavière, A. Amgoune and D. Bourissou, J. Am. Chem. Soc., 2013, 135, 13306 CrossRef CAS PubMed;
(f) O. Coulembier, J. De Winter, T. Josse, L. Mespouille, P. Gerbaux and P. Dubois, Polym. Chem., 2014, 5, 2103 RSC.
-
(a) O. Dechy-Cabaret, B. Martin-Vaca and D. Bourissou, Chem. Rev., 2004, 104, 6147 CrossRef CAS PubMed;
(b) C. Jérôme and P. Lecomte, Adv. Drug Delivery Rev., 2008, 60, 1056 CrossRef PubMed.
-
(a) D. Takeuchi, Encyclopedia of Polymer Science and Technology, ed. H. F. Mark, Wiley, 4th edn, 2014, pp. 1–26 Search PubMed;
(b) S. Dagorne, M. Normand, E. Kirillov and J.-F. Carpentier, Coord. Chem. Rev., 2013, 257, 1869 CrossRef CAS PubMed;
(c) J.-F. Carpentier, Macromol. Rapid Commun., 2010, 31, 1696 CrossRef CAS PubMed;
(d) J. Wu, T. L. Yu, C.-T. Chen and C.-C. Lin, Coord. Chem. Rev., 2006, 250, 602 CrossRef CAS PubMed.
-
(a) A. Otero, J. Fernandez-Baeza, A. Lara-Sanchez, C. Alonso-Moreno, I. Marquez-Segovia, L. F. Sanchez-Barba and A. M. Rodriguez, Angew. Chem., Int. Ed., 2009, 48, 2176 CrossRef CAS PubMed;
(b) E. Grunova, E. Kirillov, T. Roisnel and J.-F. Carpentier, Dalton Trans., 2010, 39, 6739 RSC;
(c) A. Buchard, R. H. Platel, A. Auffrant, X. F. Le Goff, P. Le Floch and C. K. Williams, Organometallics, 2010, 29, 2892 CrossRef CAS;
(d) Z. Zhang and D. Cui, Chem.–Eur. J., 2011, 17, 11520 CrossRef CAS PubMed;
(e) H. Ma and J. Okuda, Macromolecules, 2005, 38, 2665 CrossRef CAS;
(f) H. Ma, T. P. Spaniol and J. Okuda, Inorg. Chem., 2008, 47, 3328 CrossRef CAS PubMed.
-
(a) M. Visseaux and F. Bonnet, Coord. Chem. Rev., 2011, 255, 374 CrossRef CAS PubMed;
(b) S. M. Guillaume, L. Maron and P. W. Roesky, in Catalytic behavior of rare-earth borohydride complexes in polymerisation of polar monomers, ed. J.-C. G. Bünzli and V. K. Pecharsky, Elsevier, Amsterdam, 2014, vol. 244, pp. 1–86 Search PubMed;
(c) S. Guillaume, M. Schappacher and A. Soum, Macromolecules, 2003, 36, 54 CrossRef CAS;
(d) Y. Nakayama, S. Okuda, H. Yasuda and T. Shiono, React. Funct. Polym., 2007, 67, 798 CrossRef CAS PubMed;
(e) Y. Nakayama, K. Sasaki, N. Watanabe, Z. Cai and T. Shiono, Polymer, 2009, 50, 4788 CrossRef CAS PubMed;
(f) F. Bonnet, A. Cowley and P. Mountford, Inorg. Chem., 2005, 44, 9046 CrossRef CAS PubMed;
(g) H. E. Dyer, S. Huijser, N. Susperregui, F. Bonnet, A. D. Schwarz, R. Duchateau, L. Maron and P. Mountford, Organometallics, 2010, 29, 3602 CrossRef CAS;
(h) T. V. Mahrova, G. K. Fukin, A. V. Cherkasov, A. A. Trifonov, N. Ajellal and J.-F. Carpentier, Inorg. Chem., 2009, 48, 4258 CrossRef CAS PubMed;
(i) W. Li, M. Xue, J. Tu, Y. Zhanga and Q. Shen, Dalton Trans., 2012, 41, 7258 RSC;
(j) J. Kratsch, M. Kuzdrowska, M. Schmid, N. Kazeminejad, C. Kaub, P. Ona-Burgos, S. M. Guillaume and P. W. Roesky, Organometallics, 2013, 32, 1230 CrossRef CAS;
(k) A. Momin, F. Bonnet, M. Visseaux, L. Maron, M. J. Ferguson, X.-F. Le Goff, J. Takats and F. Nief, Chem. Commun., 2001, 47, 12203 RSC;
(l) F. Jaroschik, F. Bonnet, X.-F. Le Goff, F. Nief and M. Visseaux, Dalton Trans., 2010, 39, 6761 RSC.
- For examples of bulk polymerisation of lactides see:
(a) H. R. Kricheldorf, I. Kreiser-Saunders and D.-O. Damrau, Macromol. Symp., 1999, 144, 269 CrossRef CAS;
(b) P. Degée, P. Dubois, R. Jérôme, S. Jacobsen and H.-G. Fritz, Macromol. Symp., 1999, 144, 289 CrossRef.
-
(a) J.-M. Raquez, P. Degee, Y. Nabar, R. Narayan and P. Dubois, C. R. Chim., 2006, 9, 1370 CrossRef CAS PubMed;
(b) S. Jacobsen, H. G. Fritz, P. Degée, P. Dubois and R. Jérôme, Polymer, 2000, 41, 3395 CrossRef CAS;
(c) S. Bourbigot, G. Fontaine, A. Gallos and S. Bellayera, Polym. Adv. Technol., 2011, 22, 30 CrossRef CAS;
(d) A. Gallos, G. Fontaine and S. Bourbigot, Macromol. Mater. Eng., 2013, 298, 1016 CrossRef CAS;
(e) E. Deenadayalan, A. K. Lele and M. Balasubramanian, J. Appl. Polym. Sci., 2009, 112, 1391 CrossRef CAS;
(f) A. V. Machado, V. Bounor-Legare, N. D. Gonçalves, F. Melis, P. Cassagnau and A. Michel, J. Appl. Polym. Sci., 2008, 110, 3480 CrossRef CAS.
- Data available on metal chlorides allow the comparison of rare earth metals and other metals commonly used in ROP of cyclic esters, namely aluminum, zinc and tin (K. M. Stridsberg, M. Ryner and A.-C. Albertsson, Adv. Polym. Sci., 2002, 157, 42). The median lethal doses show that LaCl3 (4200 mg kg−1) is less toxic compared to AlCl3 (3700 mg kg−1), SnCl2 (700 mg kg−1), or ZnCl2 (350 mg kg−1) (N. I. Saxs, Dangerous properties of industrial materials, 6th edn, Van Nostrand Reinhold Company, New York, 1984).
-
(a) M. G. Cushion and P. Mountford, Chem. Commun., 2011, 47, 2276 RSC;
(b) R. A. Collins, J. Unruangsri and P. Mountford, Dalton Trans., 2013, 42, 759 RSC.
- S. M. Cendrowski-Guillaume, G. L. Gland, M. Nierlich and M. Ephritikhine, Organometallics, 2000, 19, 5654 CrossRef CAS.
- J. Zhang, K. Tashiro, H. Tsuji and A. J. Domb, Macromolecules, 2008, 41, 1352 CrossRef CAS.
- In the case of the PLA sample synthesized with 5, a very small fraction of linear HO-PLA-H is observed and was attributed to hydrolysis of the ester groups of the cycles by moisture traces.13e,22.
-
(a) M. H. Chisholm and E. E. Delbridge, New J. Chem., 2003, 27, 1177 RSC;
(b) G. Montaudo, M. S. Montaudo, C. Puglisi, F. Samperi, N. Spassky, A. LeBorgne and M. Wisniewski, Macromolecules, 1996, 29, 6461 CrossRef CAS.
Footnote |
† Electronic supplementary information (ESI) available: 1H NMR and MALDI-TOF MS spectra of the polylactides. See DOI: 10.1039/c4ra16634e |
|
This journal is © The Royal Society of Chemistry 2015 |