DOI:
10.1039/C4RA16449K
(Paper)
RSC Adv., 2015,
5, 13261-13269
A simple and versatile approach to self-healing polymers and electrically conductive composites†
Received
17th December 2014
, Accepted 12th January 2015
First published on 15th January 2015
Abstract
In this study, a simple and versatile approach to self-healing polymers and electrically conductive composites is reported. A series of self-healing polymers are readily synthesized by radical copolymerization of two acrylate monomers bearing a hydrogen-bonding motif. Subsequent blending with nanofillers leads to self-healing electrically conductive composites. Their glass transition temperature, the mechanical and electrical properties, and the self-healing capability can be readily tuned by the composition of the polymer as well as the filler fraction. The composite exhibits mechanical force sensing capabilities and high efficiency of both mechanical and electrical self-healing properties. Our design starts from simple chemistry and inexpensive materials, and may offer a new route towards economic self-healing electronic/electrical devices.
1 Introduction
Biological tissues like human skin are evolutionary wonders that can restore their original mechanical stability and functions after damage. Such an ability has long eluded engineering mastery and inserting this feature into synthetic systems has become an important goal for scientists and engineers. After the milestone work reported by White and co-workers,1 self-healing chemistry rapidly expanded in the past decade. A great number of self-healing materials have been demonstrated based on extrinsic or intrinsic healing.2–5 Extrinsic healing employs external healing agents set in the matrix.1,6–12 Intrinsic healing takes advantage of the reshuffling of dynamic covalent13–22 or non-covalent bonds.23–42 Self-healing materials may find numerous potential applications in a vast variety of areas.35,43–45 However, up to date, most of the studies underline the restoration of load-bearing properties. In many hot areas, it is critical and desirable to recover the functions as well after damage happens.46
Soft electrically conductive composites are an important class of materials to fabricate flexible and lightweight electronics and energy storage devices,47,48 such as conductor,49–51 semiconductor,52–54 sensor,55–57 artificial skin,58,59 electrode and solar cell.60,61 In practice, the device serves under mechanical load and are prone to structural fracture. The failure will then shorten the durability of the device, generate electronic waste, and increase the resource consumption and environmental impact. Thus, the development of self-healing electrically conductive materials is of scientific and technique importance.
Till now, a few electrically conductive materials with self-healing properties have been successfully prepared.62–64 Bielawski and colleagues pioneered a conductive thin film comprised of N-heterocyclic carbenes and transition metals.65 The sample was thermally healable, but it showed low conductivity (10−3 S cm−1). Sun and co-workers deposited Ag nanowires on polyelectrolyte multilayer films.66 They dropped ionized water at the crack to run up to healing. However, the leak of water may cause short circuits of the electronic apparatus. Moore and co-workers developed a series of conductive materials embedded with microcapsules.67–69 The break of the capsules at the cracks released the conductive liquid precursors or solvents to restore the electronic properties. But the healing agents soon exhausted, which limits the number of local-healing event.58 To surmount these obstacles, Bao and co-workers blended self-healable supramolecular polymer with conductive nanofiller to prepare electronic skin58 and anode.70 Peng and colleagues adopted similar method and reported a new family of self-healing conducting wires by wrapping conductive sheets around self-healable polymer fibers.71 Bao and Peng's products emerged high conductivity, autonomous and repeatable healing after damage. Their methods are promising for the next generation of soft conductive materials. However, the preparation of their materials involves either expensive components or complicated technique, which may restrict the scope of applications.
In this study, we report a simple and versatile approach to polymers and electrically conductive composites that are capable of autonomous and repeatable healing. As shown in Scheme 1, the polymers are made of two monomers bearing hydrogen bonding groups. These temporal hydrogen bonds can lead to supramolecular networks and reshuffle at the contact of the crack surfaces to manifest self-healing. Conductive composites are prepared by blending the polymers with conductive nanofillers. The healing efficiency of the polymers and the composite materials can be tailored by manipulation of monomer ratio and filler fraction. We focus on the material fabrication, the mechanical and electronic properties, and the self-healing ability of the composites in this work.
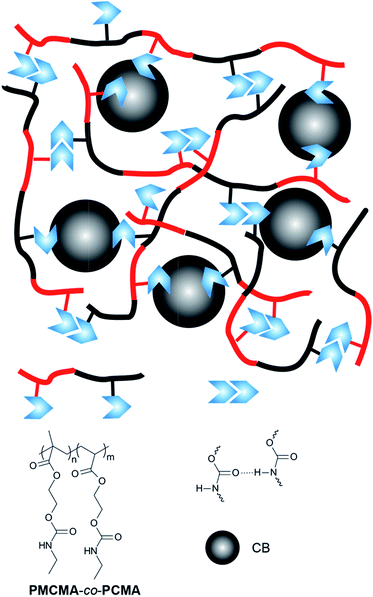 |
| Scheme 1 The design of self-healing polymers and electrically conductive composites. | |
2 Results and discussion
2.1 Material design and synthesis
Synthesis of the polymers is described in Scheme 2. Two acrylate urethane monomers were designed for the copolymerization of the self-healing polymers. The carbamate which can be easily synthesized was selected as a simple reversible hydrogen-bonding motif (Scheme 2a). Both 2-(ethylcarbamoyloxy)ethyl acrylate (CMA) and 2-(ethylcarbamoyloxy)ethyl methacrylate (MCMA) were synthesized by a one-step reaction with high yield (>85%) from inexpensive commercially available reagents. Monomer MCMA bearing a –CH3 group was used to tailor the glass transition temperature (Tg) of the copolymers by adjusting the mole percentage of MCMA in the backbone (vide infra). PMCMA-co-PCMA copolymers with various combinations of PMCMA and PCMA were synthesized using simple radical polymerization and denoted as PX/Y, where X and Y denote the mole percentage of MCMA and CMA, respectively, in the copolymers (X = 100 − Y). The hydrogen bonding formation in the copolymers was confirmed by Fourier transform infrared spectroscopy (FTIR, Fig. S6†). The peak at 3370 cm−1 is an indication of the hydrogen bonding formation between CMA and MCMA.25
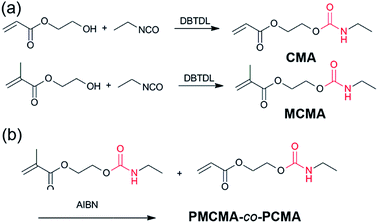 |
| Scheme 2 (a) Reactions to synthesize CMA and MCMA. (b) Random copolymerization of CMA and MCMA to prepare self-healing polymers. | |
For simple demonstration, we chose carbon black (CB) to make conductive composite for three reasons. First, CB has been widely used as conductive filler. Second, CB has a strong affinity with hydrogen bonding motifs,72,73 which helps its dispersion. Third, CB is inexpensive and readily available. Blending the copolymers with prescribed amount of CB using a solution method can afford the electrically conductive composites. Composite samples are referred to as PX/Y-CBZ, where X and Y are defined above and Z denotes the weight fraction of CB particles in the composite.
2.2 Film fabrication
Thin films of both PMCMA-co-PCMA copolymers and the composites were prepared by drop-casting. The as-prepared films are all flexible and mechanically freestanding under room temperature, except that the copolymer films are transparent and the composite films are black. Shown in Fig. 1a and b are the pictures of a P20/80 copolymer film, and Fig. 1c and d are the pictures of a P20/80–CB20 composite film.
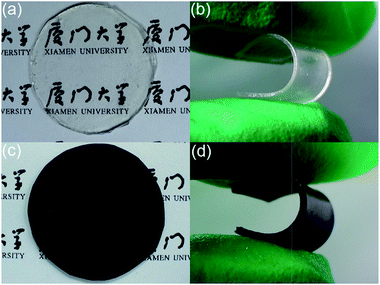 |
| Fig. 1 Photographs of self-healing polymers and conductive composites. (a) and (b) show a transparent P20/80 film. (c) and (d) is a P20/80–CB20 composite. | |
2.3 Thermal and mechanical properties
We first directed our effort to explore the effects of monomer ratio and CB concentration on Tg. Tg is known to affect the chains at the fracture interface to rearrange and diffuse,33,74 thereby the healing efficiency. Fig. 2 shows the loss tangent tan(δ) as a function of temperature T in the range of −50 to 100 °C from DMA test. All curves exhibit a single and asymmetric peak, a typical feature of the random copolymer.75,76 We define Tg as the maximum position of tan(δ). The value of Tg changes from 33 to 45 °C as the mole percentage of MCMA increases from 20% to 40%, respectively. The presence of CB shifts Tg remarkably to higher temperatures. For instance, Tg of P20/80 is 33 °C, but it is 50 °C for P20/80–CB20 (the red line in Fig. 2). CB is expected to have a strong affinity with polymer chains. Therefore, the macromolecules might bind to the surface of CB aggregates to form thin shells.77 These bounded layers experience decreased mobility and should account for the increase of Tg. Here, our observations are consistent with those in CB filled rubbers.78
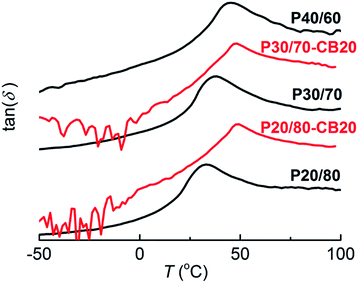 |
| Fig. 2 Tan(δ) as a function of T for self-healing polymers with varying mole percentages of MCMA (black lines) and the composites (red lines). The peak position is defined as Tg. The curves are vertically shifted for clarity. Heating rate is 5 °C min−1. | |
To be used in stretchable electronic devices, the mechanical property of a conductive composite is of critical importance. Fig. 3a shows the stress–strain response of the unfilled polymers. σN first goes up linearly with εN in the elastic region. After the yield point, plastic flow of the specimen dominates, followed by a strain-hardening behavior to the failure. The Young's modulus is in the range of 33.2 ± 5.4 to 336.3 ± 22.6 MPa, while the yield strength varies between 2.0 ± 0.1 and 10.8 ± 0.9 MPa (see more data in Table S1, ESI†). Increasing the mole percentage of MCMA improves both the Young's modulus and the yield strength but decreases the strain at break.
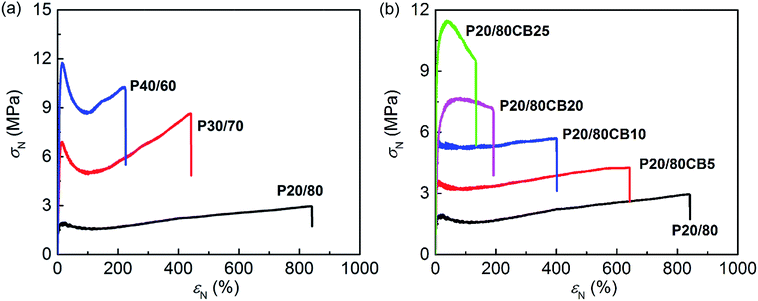 |
| Fig. 3 Mechanical characterization of the self-healing polymers and composites. (a) Nominal stress σN as a function of nominal strain εN for raw polymers with varying mole percentages of MCMA. (b) The effect of CB concentration (weight percentage) on the stress–strain response for a P20/80 polymer. The experiments were carried out at T = 25 °C with a strain rate of 0.05 s−1. | |
Fillers are known to reinforce the mechanical properties of polymers.77 Fig. 3b demonstrates the effect of CB concentration (wt%) on the stress–strain responses. For P20/80, when filled with CB, the Young's modulus and the yield strength increase tenfold and fivefold, respectively, as the CB concentration goes up to 25 wt% (Fig. S7 to S17, Table S2, ESI†). When the filler concentration is above 20 wt%, the strain-hardening region disappears (magenta and green lines in Fig. 3b). These results are in line with the decreased segment mobility in the composites, thereby the increase of Tg (DMA, Fig. 2).
2.4 Electrical properties of the composite
As shown in Fig. S24 and S25 (ESI†), all of the composites display linear, non-hysteretic current (I)–voltage (U) characteristic. The conductivity κ was obtained from three independent measurements for each weight fraction. κ reaches the order of 1 S cm−1 at 25 wt%. The increase of κ as a function of CB content follows the percolation theory (red line, see Fig. S26† for P30/70–CB20). The threshold value is 5 wt%, close to the literature reports for CB filled polymers.79,80 Good dispersion of the high structure CB in the polymer matrix is essential for the mechanical and electrical properties (Fig. S23†). This is further evidenced by SEM examination of the cross-sections of the fractures (Fig. 4a inset and Fig. S27†).
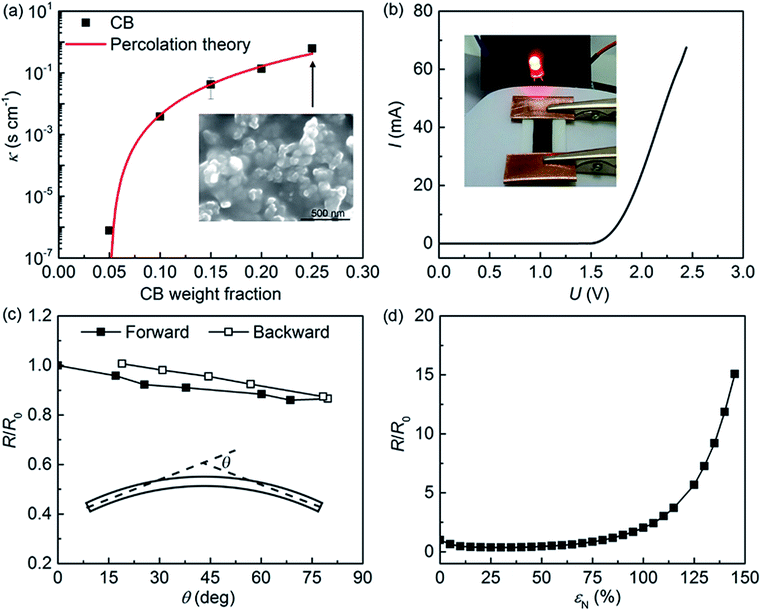 |
| Fig. 4 Electrical properties of the composite. (a) Conductivity κ as a function of CB weight fraction. The red line is the best-fit of percolation theory. The inlet is the cross-sectional SEM image of a P20/80–CB25 sample. Scale bar: 500 nm. (b) The current (I)–voltage (U) curve of an LED-integrated circuit using a P20/80–CB20 wire. The inlet shows a typical image of the circuit when the LED is on. (c) and (d) are the relative resistance changes R/R0 of a P20/80–CB20 specimen subjected to bending and stretching, respectively. | |
The excellent conductivity of our composites makes them good candidates for use as electrodes and sensors in electronic devices. For demonstration, we adopted P20/80–CB20 as a wire to turn on a light-emitting diode (LED). Fig. 4b illustrates the I–U curve of an LED-integrated circuit. The slope of the linear reign above 1.5 V gives a turn-on resistance around 10 Ω. We further studied the influence of bending and stretching on the electrical property of the film. We flexed a P20/80–CB20 specimen and recorded the relative resistance R/R0 as a function of flexion angle θ (Fig. 4c). R0 and R are the resistance before and after bending, respectively. R/R0 decreases to 0.86 as θ increases to 90°. The change is repeatable in both forward direction (increasing θ) and backward direction (decreasing θ). The drop of R/R0 is due to the reduction of the inter-distance of CB aggregates, caused by the compression stress. Fig. 4d shows the change of R/R0 of the same material (P20/80–CB20) during stretching. When εN is smaller than the yield strain (ca. ∼70%), the resistance is close to its original value, i.e., R/R0 ∼ 1. As εN is above 100%, R/R0 abruptly increases. At high strain, the inter distance of CB aggregates increases and their contacts break. Both effects can hinder the hopping of the electrons. These responses to bending and stretching render the composites promising materials for sensing mechanical forces.
2.5 Self-healing properties
Having demonstrated the thermal, mechanical, and electrical properties of our composites, we then studied their self-healing ability.
In the first set of experiments, we explored the healing in mechanical property of the bulk film. As sketched in Fig. 5a, we bisected a thin film and kept the halves in contact for 15 s. The film was either left to heal at room temperature for 24 h (thereafter “HRT”) or heated at 60 °C for 1 h (thereafter “H60”). Dumbbell-shaped specimens were punched from the healed film. Pristine specimen was obtained from untreated film and referred to as “Pri”. We define the mechanical property healing efficiency η as the restoration of toughness (the area under the stress–strain curve) after healing. Fig. 5b shows representative stress–strain curves for filled and unfilled P20/80. Unfilled samples (blue lines) emerge excellent self-healing ability with η = 95% (blue dashed line). If the interfaces were left in ambient air for over 5 min, η drops to 63% because of the moisture from the ambient air which binds to hydrogen bonding motifs. This infers that the hydrogen bonding network plays important role in the healing mechanism. For fixed CMA/MCMA ratio, the higher of the filler concentration, the lower η is. For example, η drops from 83% to 40% as the filler concentration changes from 5 wt% (red lines) to 20 wt% (black lines) under same conditions. We can improve η by healing at elevated temperature to accelerate chain diffusion for highly filled composites. For instance, η of P20/80–CB20 healed at 60 °C for 1 h reaches 85%, much higher than that healed at room temperature. These results indicate that the healing mechanism is also related with the mobility of the polymer chains. The CB aggregates as well as the polymer layers bounded to them drastically slow down the mobility of the segments (higher Tg). Collectively, the healing mechanism is highly correlated with the binding strength of the dynamic bonds as well as the diffusion of the chains. P30/70 composites display similar mechanical healing behaviors (Fig. S19, ESI†).
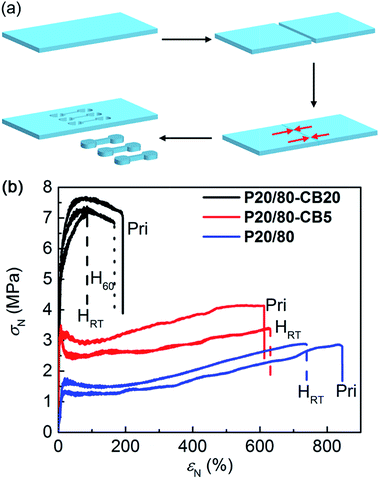 |
| Fig. 5 (a) Schematic representation of self-healing study using mechanical characterization. (b) Typical stress–strain curves of P20/80 (blue lines), P20/80–CB5 (red lines), and P20/80–CB20 (black lines). Pri: pristine sample (solid lines). HRT: healed at room temperature for 24 h (dashed lines). H60: healed at elevated temperature (60 °C) for 1 h (dotted line). | |
Based on above results, we propose here a simplified healing mechanism. When cut, the hydrogen bonds may be dissociated. After the broken interfaces are joined together, the polymer chains diffuse across the wounded boundaries. At the same time, the exchanges of hydrogen bonding motifs and the formation of new hydrogen bonds lead to bridges of supramolecular network across the interfaces, and the damage is healed.
In the second set of experiments, we examined their ability to restore electrical conductivity. We overplayed a film on the top of another film of the same composite. The jointed films were placed between two copper sheets (Fig. 6a). We recorded the conductivity of the jointed films (κd) relative to that of a single film (κs) as a function of time t (Fig. 6b). At the beginning, κd ≪ κs because of the interface between the two films which hinders current transmission. κd/κs gradually improves and approaches to 1, suggesting the fusion of the boundaries, i.e., efficient healing. We then peeled the two films off and rejoined them. κd/κs suddenly decreases and approaches to 1 again. This procedure can be repeated several times (Fig. 6b).
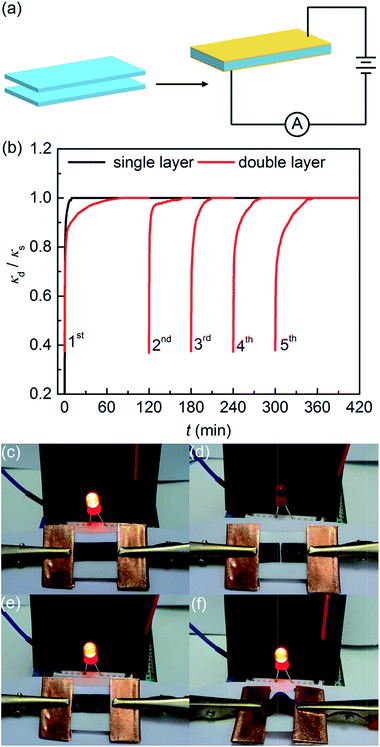 |
| Fig. 6 (a) Schematic representation of electronic characterization of self-healing composite by overlapping two films together. The yellow plates represent Cu sheets. (b) Relative changes of conductivity κ/κ0 as a function of time t after the overlapping. (c) to (f) Demonstration of the healing process for a conductive composite in an LED-integrated circuit: (c) pristine conductor; (d) open circuit by breaking the film; (e) healed film; (f) healed film subjected to bending. | |
To demonstrate the healing in both the mechanical and electrical properties intuitively, we integrated our composite into an LED circuit as an electrode (Fig. 6c). When the film was bifurcated using a razor blade, the lighted LED was extinguished (Fig. 6e). The two pieces of the electrode were then brought together and gently pressed (Fig. 6e). The LED lighted up again as with the use of the pristine film. After 5 min at room temperature, the healed electrode displayed good flexibility (Fig. 6f).
3 Conclusions
In summary, we have demonstrated a simple and versatile approach to self-healing polymers and electrically conductive composites. Two monomers MCMA and CMA bearing carbamate group were synthesized via one-step reaction from inexpensive starting materials. Their random copolymers formed transient networks through hydrogen bonding interactions. Flexible electrically conductive composites were readily prepared by the incorporation of CB fillers into the polymer host. The mole percentage of MCMA and the CB weight fraction greatly affected Tg and the mechanical properties of both the polymers and the composites. Conductivity of the composites as a function of filler content followed the prediction of percolation theory. At 25 wt% CB, the conductivity reached the order of 1 S cm−1, making the composites suitable for uses in electronic/electrical devices. The conductivity was also found to responsive to bending and stretching, rendering the composites promising materials for sensing mechanical forces. Both the polymer and the conductive composites exhibited notable self-healing abilities, which can be ascribed to the dynamic associations of hydrogen-bonding carbamate motifs and the diffusion of the polymer chains across the fractured surfaces. The healing efficiency could be tuned by the polymer composition as well as the CB fraction.
As far as we know, this is the first example of self-healing conductor and electronic mechanical sensor involved with simple chemistry and low-cost materials. Our design may offer a new route towards the fabrication of economic self-healing electronic/electrical devices in the future.
4 Experimental
4.1 Reagents and materials
Ethyl isocyanate (98%), 2-hydroxyethyl acrylate (99.5%), and 2-hydroxyethyl methacrylate (96%) were purchased from Energy Chemical. 2,2′-Azobis(2-methyl propionitrile) (AIBN) was obtained from Aladdin. Dibutylbis((1-oxododecyl)oxy)-stannane (DBTDL), dimethylformamide (DMF), methanol and diethyl ether were purchased from J & K and used as received. Carbon black (CB, acetylene, 100%) was obtained from Alfa Aesar. The filler has a specific surface area of 75 m2 g−1 and a bulk density in the range of 170 to 230 g L−1.
4.2 Synthesis of monomers
Scheme 2a sketches the synthesis of CMA and MCMA. For CMA, ethyl isocyanate 17.96 g (0.25 mol, 1.0 equiv.) and 2-hydroxyethyl acrylate 33.18 g (0.28 mol, 1.1 equiv.) were added and mixed in a 100 mL Schlenk flask. Ten drops of DBTDL were then added dropwise. The mixture was kept stirring at room temperature for 4 h under nitrogen atmosphere. The mixture was diluted by 20 mL CH2Cl2 and washed with deionized water for 5 times to remove unreacted 2-hydroxyethyl acrylate. The organic phase was dried over anhydrous Na2SO4, filtered, and solvent evaporated. A colourless and viscous liquid was obtained (39.87 g, 85% yield). Followed the same procedures as CMA, MCMA was obtained (90% yield) using 2-hydroxyethyl methacrylate as starting material. The 1H NMR spectra of both monomers are shown in Fig. S1 to S2 (ESI†).
4.3 Synthesis of PMCMA-co-PCMA
For a typical polymerization (Scheme 2b), MCMA 7.26 g (0.036 mol, 70 equiv.), CMA 15.77 g (0.084 mol, 30 equiv.), AIBN 198 mg (1.206 mmol, 1.0 equiv.) and DMF (90 mL) were dissolved in a 250 mL Schlenk flask. The mixture was degassed with three freeze–pump–thaw cycles. The flask was kept in an oil bath (70 °C) and the mixture was mechanically stirred for 30 h. Raw product was precipitated three times in diethyl ether to remove residual monomers. Dry solid was obtained by solvent evaporation under vacuum at 70 °C (Fig. S3 to S5, ESI†).
4.4 Fourier transform infrared spectroscopy (FTIR)
FTIR spectroscopic analyses were obtained with a Nicolet380 Fourier transformation infrared spectrometer coupled with an infrared microscope spectrometer (produced by Thermo Electron Corporation, U.S.A.), operating in transmission mode. Filmy samples were produced by casting the solution of the polymer onto KBr disks.
4.5 Fabrication of conductive composites
PMCMA-co-PCMA (0.800 g) was dissolved in methanol (5 mL) and mixed with CB (0.200 g) in a Teflon caster. The mixture was stirred for 4 h and sonicated for 20 min to stabilize the suspension. The suspension was then slowly dried under room temperature for 96 h. The residue solvent was further removed by vacuum drying at 70 °C for another 24 h and clean film was harvested from the caster.
4.6 Tensile test
Dumbbell-shaped specimens were punched from films for tensile test. The dimensions of the sample were 5.0 × 2.0 × 0.5 mm (length, width, and thickness). Measurements were performed on an Instron 3343 machine. The strain rate was set at 0.05 s−1 (a rough cross head speed of 0.25 mm s−1). Nominal strain is defined as εN = (L − L0)/L0 × 100%, where L0 and L are the distance between the two clamps before and after stretching, respectively. The nominal stress σN = F/S0 (F is the force at the grips, and S0 is the area of the cross section before deformation).
4.7 Dynamic mechanical analysis (DMA)
Films were cut into rectangular shapes of 15.0 × 2.0 × 0.6 mm (length, width, and thickness). Tests were carried out on NETZSCH DMA 242C. Data were recorded as a function of temperature from −100 to 120 °C at a frequency of 1 Hz. Heating rate was set at 5 °C min−1.
4.8 Scanning electron microscope (SEM)
Specimens were immersed in liquid nitrogen and then fractured. The fracture surface of the composite was observed on Hitachi S-4800.
4.9 Conductivity measurement
For a typical conductivity measurement, a specimen was loaded between two parallel copper plates and the resistance (I–U) was measured using a Victor/vc 890C+ digital multimeter.
4.10 Self-healing test
For mechanical healing test, thin films were bisected and the fracture surfaces were kept contact for 15 s. Healing was performed at room temperature for 24 h or at 60 °C for 1 h. The later procedure only applied for highly filled samples. Dumbbell-shaped specimens were then punched from the healed films. The mechanical healing efficiency η is defined as: η = WH/WP, where WP and WH are the toughness (area under the stress–strain curve) of the pristine and the healed sample, respectively.
To demonstrate the restoration of electrical properties, two sets of experiments were carried out. In the first demonstration, a film was overplayed on the top of another film of the same composite. The jointed films were placed in between two copper plates and the conductivity of the jointed films (κd) as a function of time was recorded. The healed films were then separated at the jointed surfaces and the measurement was repeated. Similar measurement was conducted on a single film (κs) for comparison. In a second demonstration, a composite film was connected in series with an LED and used as an electrical conductor. Turning-off and on of the LED was controlled by breaking the film and jointing the damaged film, respectively.
Acknowledgements
This work was supported by National Natural Science Foundation of China (no. 21304076) and China Postdoctoral Science Foundation (no. 2013M541857 and no. 2014T70612).
Notes and references
- S. R. White, N. R. Sottos, P. H. Geubelle, J. S. Moore, M. R. Kessler, S. R. Sriram, E. N. Brown and S. Viswanathan, Nature, 2001, 409, 794–797 CrossRef CAS PubMed.
- S. D. Bergman and F. Wudl, J. Mater. Chem., 2008, 18, 41–62 RSC.
- D. Y. Wu, S. Meure and D. Solomon, Prog. Polym. Sci., 2008, 33, 479–522 CrossRef CAS.
- E. B. Murphy and F. Wudl, Prog. Polym. Sci., 2010, 35, 223–251 CrossRef CAS.
- M. D. Hager, P. Greil, C. Leyens, S. van der Zwaag and U. S. Schubert, Adv. Mater., 2010, 22, 5424–5430 CrossRef CAS PubMed.
- K. S. Toohey, N. R. Sottos, J. A. Lewis, J. S. Moore and S. R. White, Nat. Mater., 2007, 6, 581–585 CrossRef CAS PubMed.
- M. W. Keller, S. R. White and N. R. Sottos, Adv. Funct. Mater., 2007, 17, 2399–2404 CrossRef CAS.
- M. M. Caruso, B. J. Blaiszik, S. R. White, N. R. Sottos and J. S. Moore, Adv. Funct. Mater., 2008, 18, 1898–1904 CrossRef CAS.
- B. J. Blaiszik, M. Baginska, S. R. White and N. R. Sottos, Adv. Funct. Mater., 2010, 20, 3547–3554 CrossRef CAS.
- K. S. Toohey, C. J. Hansen, J. A. Lewis, S. R. White and N. R. Sottos, Adv. Funct. Mater., 2009, 19, 1399–1405 CrossRef CAS.
- C. C. Corten and M. W. Urban, Adv. Mater., 2009, 21, 5011–5015 CrossRef CAS PubMed.
- J.-H. Park and P. V. Braun, Adv. Mater., 2010, 22, 496–499 CrossRef CAS PubMed.
- R. J. Wojtecki, M. A. Meador and S. J. Rowan, Nat. Mater., 2011, 10, 14–27 CrossRef CAS PubMed.
- X. X. Chen, M. A. Dam, K. Ono, A. Mal, H. B. Shen, S. R. Nutt, K. Sheran and F. Wudl, Science, 2002, 295, 1698–1702 CrossRef CAS PubMed.
- B. J. Adzima, C. J. Kloxin and C. N. Bowman, Adv. Mater., 2010, 22, 2784–2787 CrossRef CAS PubMed.
- P. Reutenauer, E. Buhler, P. J. Boul, S. J. Candau and J. M. Lehn, Chem.–Eur. J., 2009, 15, 1893–1900 CrossRef CAS PubMed.
- C. M. Chung, Y. S. Roh, S. Y. Cho and J. G. Kim, Chem. Mater., 2004, 16, 3982–3984 CrossRef CAS.
- H. Otsuka, K. Aotani, Y. Higaki, Y. Amamoto and A. Takahara, Macromolecules, 2007, 40, 1429–1434 CrossRef CAS.
- J. Canadell, H. Goossens and B. Klumperman, Macromolecules, 2011, 44, 2536–2541 CrossRef CAS.
- M. Pepels, I. Filot, B. Klumperman and H. Goossens, Polym. Chem., 2013, 4955–4965 RSC.
- Y. Amamoto, J. Kamada, H. Otsuka, A. Takahara and K. Matyjaszewski, Angew. Chem., Int. Ed., 2011, 50, 1660–1663 CrossRef CAS PubMed.
- H. M. Klukovich, Z. S. Kean, S. T. Iacono and S. L. Craig, J. Am. Chem. Soc., 2011, 133, 17882–17888 CrossRef CAS PubMed.
- P. Cordier, F. Tournilhac, C. Soulie-Ziakovic and L. Leibler, Nature, 2008, 451, 977–980 CrossRef CAS PubMed.
- A. Vidyasagar, K. Handore and K. M. Sureshan, Angew. Chem., Int. Ed., 2011, 50, 8021–8024 CrossRef CAS PubMed.
- S. Burattini, B. W. Greenland, D. H. Merino, W. Weng, J. Seppala, H. M. Colquhoun, W. Hayes, M. E. Mackay, I. W. Hamley and S. J. Rowan, J. Am. Chem. Soc., 2010, 132, 12051–12058 CrossRef CAS PubMed.
- Q. Wang, J. L. Mynar, M. Yoshida, E. Lee, M. Lee, K. Okuro, K. Kinbara and T. Aida, Nature, 2010, 463, 339–343 CrossRef CAS PubMed.
- A. B. South and L. A. Lyon, Angew. Chem., Int. Ed., 2010, 49, 767–771 CrossRef CAS PubMed.
- W. Weng, J. B. Beck, A. M. Jamieson and S. J. Rowan, J. Am. Chem. Soc., 2006, 128, 11663–11672 CrossRef CAS PubMed.
- W. Weng, Z. Li, A. M. Jamieson and S. J. Rowan, Macromolecules, 2009, 42, 236–246 CrossRef CAS.
- W. Weng, Z. Li, A. M. Jamieson and S. J. Rowan, Soft Matter, 2009, 5, 4647–4657 RSC.
- J. Yuan, X. Fang, L. Zhang, G. Hong, Y. Lin, Q. Zheng, Y. Xu, Y. Ruan, W. Weng, H. Xia and G. Chen, J. Mater. Chem., 2012, 22, 11515–11522 RSC.
- G. Hong, H. Zhang, Y. Lin, Y. Chen, Y. Xu, W. Weng and H. Xia, Macromolecules, 2013, 46, 8649–8656 CrossRef CAS.
- M. Burnworth, L. Tang, J. R. Kumpfer, A. J. Duncan, F. L. Beyer, G. L. Fiore, S. J. Rowan and C. Weder, Nature, 2011, 472, 334–338 CrossRef CAS PubMed.
- M. Zhang, D. Xu, X. Yan, J. Chen, S. Dong, B. Zheng and F. Huang, Angew. Chem., Int. Ed., 2012, 51, 7011–7015 CrossRef CAS PubMed.
- Y. Chen, A. M. Kushner, G. A. Williams and Z. Guan, Nat. Chem., 2012, 4, 467–472 CrossRef CAS PubMed.
- J. Hentschel, A. M. Kushner, J. Ziller and Z. Guan, Angew. Chem., Int. Ed., 2012, 51, 10561–10565 CrossRef CAS PubMed.
- Y. Chen and Z. Guan, Chem. Commun., 2014, 50, 10868–10870 RSC.
- T. Wang, S. Zheng, W. Sun, X. Liu, S. Fu and Z. Tong, Soft Matter, 2014, 10, 3506–3512 RSC.
- Z. Xu, J. Peng, N. Yan, H. Yu, S. Zhang, K. Liu and Y. Fang, Soft Matter, 2013, 9, 1091–1099 RSC.
- U. Gulyuz and O. Okay, Soft Matter, 2013, 9, 10287–10293 RSC.
- G. Akay, A. Hassan-Raeisi, D. C. Tuncaboylu, N. Orakdogen, S. Abdurrahmanoglu, W. Oppermann and O. Okay, Soft Matter, 2013, 9, 2254–2261 RSC.
- F. Maes, D. Montarnal, S. Cantournet, F. Tournilhac, L. Corte and L. Leibler, Soft Matter, 2012, 8, 1681–1687 RSC.
- Y. Li, L. Li and J. Sun, Angew. Chem., Int. Ed., 2010, 49, 6129–6133 CrossRef CAS PubMed.
- T.-S. Wong, S. H. Kang, S. K. Y. Tang, E. J. Smythe, B. D. Hatton, A. Grinthal and J. Aizenberg, Nature, 2011, 477, 443–447 CrossRef CAS PubMed.
- E. Palleau, S. Reece, S. C. Desai, M. E. Smith and M. D. Dickey, Adv. Mater., 2013, 25, 1589–1592 CrossRef CAS PubMed.
- M. Zhu, M. Z. Rong and M. Q. Zhang, Polym. Int., 2014, 63, 1741–1749 CrossRef CAS.
- Z. Suo, MRS Bull., 2012, 37, 218–225 CrossRef CAS.
- S. J. Benight, C. Wang, J. B. H. Tok and Z. Bao, Prog. Polym. Sci., 2013, 38, 1961–1977 CrossRef CAS.
- T. Sekitani, H. Nakajima, H. Maeda, T. Fukushima, T. Aida, K. Hata and T. Someya, Nat. Mater., 2009, 8, 494–499 CrossRef CAS PubMed.
- S.-K. Lee, B. J. Kim, H. Jang, S. C. Yoon, C. Lee, B. H. Hong, J. A. Rogers, J. H. Cho and J.-H. Ahn, Nano Lett., 2011, 11, 4642–4646 CrossRef CAS PubMed.
- S. Bae, H. Kim, Y. Lee, X. Xu, J.-S. Park, Y. Zheng, J. Balakrishnan, T. Lei, H. Ri Kim, Y. I. Song, Y.-J. Kim, K. S. Kim, B. Ozyilmaz, J.-H. Ahn, B. H. Hong and S. Iijima, Nat. Nanotechnol., 2010, 5, 574–578 CrossRef CAS PubMed.
- Y. Zhang, M. Yu, D. E. Savage, M. G. Lagally, R. H. Blick and F. Liu, Appl. Phys. Lett., 2010, 96, 111904 CrossRef.
- K. Park, D.-K. Lee, B.-S. Kim, H. Jeon, N.-E. Lee, D. Whang, H.-J. Lee, Y. J. Kim and J.-H. Ahn, Adv. Funct. Mater., 2010, 20, 3577–3582 CrossRef CAS.
- D.-Y. Khang, H. Jiang, Y. Huang and J. A. Rogers, Science, 2006, 311, 208–212 CrossRef CAS PubMed.
- B. Y. Lee, K. Heo, J. H. Bak, S. U. Cho, S. Moon, Y. D. Park and S. Hong, Nano Lett., 2008, 8, 4483–4487 CrossRef CAS PubMed.
- H. U. Khan, M. E. Roberts, W. Knoll and Z. Bao, Chem. Mater., 2011, 23, 1946–1953 CrossRef CAS.
- D.-H. Kim, R. Ghaffari, N. Lu, S. Wang, S. P. Lee, H. Keum, R. D'Angelo, L. Klinker, Y. Su, C. Lu, Y.-S. Kim, A. Ameen, Y. Li, Y. Zhang, B. de Graff, Y.-Y. Hsu, Z. Liu, J. Ruskin, L. Xu, C. Lu, F. G. Omenetto, Y. Huang, M. Mansour, M. J. Slepian and J. A. Rogers, Proc. Natl. Acad., 2012, 109, 19910–19915 CrossRef CAS PubMed.
- B. C.-K. Tee, C. Wang, R. Allen and Z. Bao, Nat. Nanotechnol., 2012, 7, 825–832 CrossRef CAS PubMed.
- C. Hou, T. Huang, H. Wang, H. Yu, Q. Zhang and Y. Li, Sci. Rep., 2013, 3, 3138 Search PubMed.
- M. Kaltenbrunner, M. S. White, E. D. Głowacki, T. Sekitani, T. Someya, N. S. Sariciftci and S. Bauer, Nat. Commun., 2012, 3, 770 CrossRef PubMed.
- D. J. Lipomi, B. C. K. Tee, M. Vosgueritchian and Z. Bao, Adv. Mater., 2011, 23, 1771–1775 CrossRef CAS PubMed.
- H. Wang, B. Zhu, W. Jiang, Y. Yang, W. R. Leow, H. Wang and X. Chen, Adv. Mater., 2014, 26, 3638–3643 CrossRef CAS PubMed.
- L. Lin, S. Liu, Q. Zhang, X. Li, M. Ji, H. Deng and Q. Fu, ACS Appl. Mater. Interfaces, 2013, 5, 5815–5824 CAS.
- Y. Hong and M. Su, ACS Appl. Mater. Interfaces, 2012, 4, 3759–3764 CAS.
- K. A. Williams, A. J. Boydston and C. W. Bielawski, J. R. Soc., Interface, 2007, 4, 359–362 CrossRef CAS PubMed.
- Y. Li, S. Chen, M. Wu and J. Sun, Adv. Mater., 2012, 24, 4578–4582 CrossRef CAS PubMed.
- S. A. Odom, S. Chayanupatkul, B. J. Blaiszik, O. Zhao, A. C. Jackson, P. V. Braun, N. R. Sottos, S. R. White and J. S. Moore, Adv. Mater., 2012, 24, 2578–2581 CrossRef CAS PubMed.
- B. J. Blaiszik, S. L. B. Kramer, M. E. Grady, D. A. McIlroy, J. S. Moore, N. R. Sottos and S. R. White, Adv. Mater., 2012, 24, 398–401 CrossRef CAS PubMed.
- S. A. Odom, M. M. Caruso, A. D. Finke, A. M. Prokup, J. A. Ritchey, J. H. Leonard, S. R. White, N. R. Sottos and J. S. Moore, Adv. Funct. Mater., 2010, 20, 1721–1727 CrossRef CAS.
- C. Wang, H. Wu, Z. Chen, M. T. McDowell, Y. Cui and Z. Bao, Nat. Chem., 2013, 5, 1042–1048 CrossRef CAS PubMed.
- H. Sun, X. You, Y. Jiang, G. Guan, X. Fang, J. Deng, P. Chen, Y. Luo and H. Peng, Angew. Chem., 2014, 126, 9680–9685 CrossRef.
- G. Kraus, Reinforcement of elastomers by carbon black in Fortschritte der Hochpolymeren-Forschung, Springer, Berlin Heidelberg, 1971, vol. 8, pp. 155–237 Search PubMed.
- D. J. Kohls and G. Beaucage, Curr. Opin. Solid State Mater. Sci., 2002, 6, 183–194 CrossRef CAS.
- R. P. Wool, Soft Matter, 2008, 4, 400–418 RSC.
- H. Daimon, H. Okitsu and J. Kumanotani, Polym. J., 1975, 7, 460–466 CrossRef CAS.
- E. Penzel, J. Rieger and H. A. Schneider, Polymer, 1997, 38, 325–337 CrossRef CAS.
- G. Heinrich, M. Kluppel and T. A. Vilgis, Curr. Opin. Solid State Mater. Sci., 2002, 6, 195–203 CrossRef CAS.
- C. G. Robertson and C. M. Roland, Rubber Chem. Technol., 2008, 81, 506–522 CrossRef CAS.
- Q.-H. Zhang and D.-J. Chen, J. Mater. Sci., 2004, 39, 1751–1757 CrossRef CAS.
- J. Liang and Q. Yang, J. Appl. Phys., 2007, 102, 083508 CrossRef.
Footnotes |
† Electronic supplementary information (ESI) available: 1H NMR spectra of the two monomers, stress–strain curves, SAXS patterns, and DMA were included. See DOI: 10.1039/c4ra16449k |
‡ X. T. and H. Z. contributed equally to this work. The authors declare no competing financial interest. |
|
This journal is © The Royal Society of Chemistry 2015 |