DOI:
10.1039/C4RA15704D
(Paper)
RSC Adv., 2015,
5, 33503-33514
3D-nanoflowers of rutile TiO2 as a film grown on conducting and non-conducting glass substrates for in vitro biocompatibility studies with mouse MC3T3 osteoblast and human HS-5 cells
Received
3rd December 2014
, Accepted 25th March 2015
First published on 25th March 2015
Abstract
Thin films of 3D-nanoflowers of rutile TiO2 on conducting (FTO and ITO) and non-conducting (glass) substrates were grown using a surfactant free one-step hydrothermal process. Field emission scanning electron microscopy (FE-SEM) observations confirmed the transformation of TiO2 nanostructures from mesh-like to 3D-nanoflowers with an increase in hydrolysis rate during the growth of the TiO2 films. The X-ray diffraction (XRD) pattern of the TiO2 nanostructures as films grown on different substrates showed that under various conditions, they have a phase pure rutile crystallite structure. The high resolution transmission electron microscopy (HR-TEM) diffraction pattern of the TiO2 nanostructures showed tightly packed assemblies of titanium atoms and a lattice spacing of 0.23 nm along the longitudinal axis direction of rutile TiO2. X-ray photoelectron spectroscopic (XPS) analysis of the TiO2 nanostructures grown as films on glass substrates showed a spectral shift of 0.53 eV in binding energy, which confirms the charge accumulation on the non-conducting substrate, whereas there was no spectral shift observed for TiO2 films with similar structures grown on conducting substrates. The accumulated charge on the conducting surfaces can be easily neutralized, whereas the non-conducting surfaces may retain these accumulated charges. The adhesion, viability and proliferation response of mouse osteoblast (MC3T3) and human stromal (HS-5) cells on the 3D-nanoflowers of TiO2 as films grown on conducting and non-conducting substrates were assessed. The adhesion and proliferation of both the type of cells showed a better response on non-conducting surfaces as compared to conducting surfaces, despite the similar crystallite structures and nanomorphology of TiO2. Stromal cells had potential to prepare extra-cellular matrix scaffolds for the ex vivo expansion/differentiation of stem cells. Therefore, the current findings can be used to prepare 3D TiO2 nanostructure supported cellular scaffolds for regenerative medicine in the future.
1. Introduction
During the past two decades, substantial research work has been carried out to understand the correlation between the different process parameters of various synthesis methods and their effects on tailoring the structural and functional properties of metal and metal oxides.3–8 In the past, the use of metal oxides such as titanium dioxides with tunable nanostructural and chemical properties has been extensively explored for catalytic, energy and biomedical applications.9–13 Different synthesis methods were employed to tailor the structural and functional properties of metal oxides by manipulating the processing conditions to obtain the desired arrangements of the nanocrystallites.13–18 For each synthesis method, detailed studies were carried out to understand the effects of the processing parameters on the modification of basic structural properties; however, to obtain materials with a higher degree of phase purity and tunable surface morphologies with the desired surface chemical functionalities is still a challenge.19–21
A number of researchers have addressed the challenges associated with the tunable surface morphology and synthesis of micro/nanostructured titanium oxides with highly oriented rod-like crystals.22–27 The control over the crystallite phase and morphologies were achieved either by surface treatment or metal ion doping.28–31 Thin films of titanium oxides were deposited with controllable crystallite phases by choosing appropriate metal ion doping and were used for different applications.32,33 Well ordered 1D TiO2 as nanorods, nanowires, nanocorals, hierarchical microspheres and nanotubes were synthesized and extensive studies were performed to use these materials for energy and biosensor applications.23–26 Previously, the biocompatibility of titanium oxides as thin film coatings deposited by plasma sputtering and dip coating was studied with different types of cells.9,12,28 The simultaneous growth of 1D/3D nanorods/nanoflowers is advantageous for better adhesion at the interface of the 1D/3D nanostructures.34 However, the use of 1D and 3D nano-assemblies of TiO2 in biomedical fields has not been fully explored to date.
Herein, we report a surfactant free one-step hydrothermal process for the growth of 1D/3D nano-morphologies of TiO2 under various conditions. The simultaneous growth of 3D nanoflower-like structures on 1D nanorods provides better adhesion at the interface of 1D/3D nanorods. The other advantage of the method used in this study was to achieve uniform coatings on all the sides of the substrates in a single step. The physicochemical properties of the nanostructures of TiO2 grown on different substrates (non-conducting glass and FTO/ITO coated conducting glass) were investigated. The molar ratio of precursor material and reaction time were optimized to grow 1D/3D nanostructures of rutile TiO2 as films on different types of substrates. In this study, we have reported, for the first time, the cellular response of osteoblast and HS-5 cells with TiO2 by changing the conducting and non-conducting nature of the substrates without altering the 3D nanostructures. The use of osteoblast cells for assessing the biocompatibility of metal oxide and polymeric materials is a well-accepted in vitro model system.35–38 To further assess the applicability of the 1D/3D nanostructures, human stromal cells, which have potential for preparing an extra-cellular matrix scaffold were cultured on different types of nano-assemblies of TiO2. In vitro cell adhesion, viability and proliferation assays using human HS-5 cells were performed. The outcomes of this study may have potential in the future use of these substrates to prepare 3D nanostructure supported cellular scaffolds1,2 for application in regenerative medicine.
2. Experimental
2.1 Materials
Titanium isopropoxide (Ti[OCH(CH3)2]4, 97%), hydrochloric acid (HCl, 37 wt%) and isopropyl alcohol were purchased from Sigma-Aldrich (Sigma-Aldrich) and used as received. The plastic wares used for cell culture in this study were obtained from Nunc, USA. Culture media (α-MEM) was obtained from Invitrogen. All other tissue culture reagents and MTT (3-(4,5-dimethylthiazol-2-yl)-2,5-diphenyltetrazolium were purchased from Sigma. All other analytical grade chemicals and solvents were purchased from local companies.
2.2 Hydrothermal growth of TiO2 nanostructures as films
For the growth of nanostructures of TiO2 as films, a solution of titanium isopropoxide was prepared in HCl. Six different dilution ratios of HCl with water, namely, (i) 20
:
80, (ii) 30
:
70, (iii) 40
:
60, (iv) 50
:
50, (v) 60
:
40 and (vi) 70
:
30, were prepared and used to control the hydrolysis rate of titanium isopropoxide. In the final step, 1 ml of titanium isopropoxide was added to 40 ml of HCl solutions mentioned above ((i)–(vi)). The mixed solutions of titanium isopropoxide and HCl solutions were placed in a Teflon-lined stainless steel autoclave. The temperature of the autoclave was set at 180 °C and the growths of the nanostructures were observed after 3 h on FTO substrates for the conditions (i) to (vi). After the reaction was completed, the autoclave was allowed to cool to room temperature and the TiO2 nanostructures grown on FTO substrates under the different conditions described above were collected and dried in vacuum at 70 °C for 12 h before use.
Fig. 1 shows the FE-SEM images of the TiO2 nanostructures grown on FTO at various dilution conditions of HCl ((i)–(vi)). The TiO2 nanostructures obtained under conditions ((i)–(iii)) with a higher water content in HCl showed round ball-like 3D TiO2 nanostructures (Fig. 1a–c). A very significant change in the surface structure morphology was observed with an increase in the dilution of HCl. The FE-SEM images of the TiO2 nanostructures obtained at higher concentrations of HCl (condition vi) show a mesh-like nanostructure. The mesh-like nanostructures of TiO2 were changed to 3D-nanoflower-like structures with the use of an equal volume to volume ratio of water and HCl. TiO2 nanostructures obtained using an equal volume to volume ratio of water and HCl (condition iv) showed a uniform distribution of 3D-nanoflowers of TiO2 on FTO. Therefore, this optimum condition (iv) was used for the further investigation of the effects of reaction time on TiO2 nanostructure growth as films on FTO. In this study, three different types of substrates (i) non-conducting glass (glass), (ii) ITO coated conducting glass (ITO) and (iii) FTO coated conducting glass (FTO) were used.
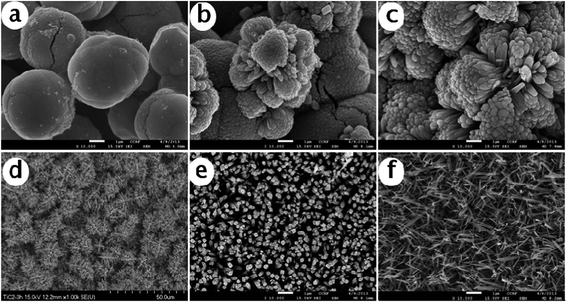 |
| Fig. 1 FE-SEM images of rutile TiO2 nanostructures grown on FTO substrates. (a)–(f) represent the nanostructures grown with different dilutions of HCl. HCl to water ratio: (a) 20 : 80; (b) 30 : 70; (c) 40 : 60; (d) 50 : 50; (e) 60 : 40; (f) 70 : 30 at 180 °C. | |
TiO2 films were grown under four different types of conditions: (a) 1 h on FTO, (b) 3 h on FTO, (c) 3 h on ITO and (d) 3 h on glass substrates for the quantification of the detailed crystallite structure using X-ray diffraction (XRD), surface chemical analysis by X-ray photoelectron spectroscopy (XPS) and cell culture experiments to assess their biocompatibility.
2.3 Cell culture
MC3T3 and HS-5 cells were maintained in α-MEM with 10% fetal bovine serum (FBS) and 100 U per mL penicillin, and incubated at 37 °C in an atmosphere of 5% CO2. Trypsin–EDTA was used to detach the cells. Three samples were used for in vitro cell adhesion, viability and proliferation assays and all the experiments were repeated three times. For statistical analysis, a pairwise comparison of inter-experiment variation was carried out. MS Excel software was used for statistical analysis. Standard deviation values were calculated separately for all the assays. The significance of the statistical analysis values was determined using the Student's t-test, where p-values <0.05 were considered significant. The cell culture experiments were performed on TiO2 3D-nanoflower structures grown on FTO, ITO and glass substrates under condition (iv) for 3 h. In addition, TiO2 nanostructures grown at an early stage (1 h) on the FTO substrate under condition (iv) were selected to perform cell culture experiments and tissue culture plastic surface was used as the control.
2.4 Characterization
The crystallite structure of 1D- and 3D-TiO2 nanostructures grown on different substrates was characterized from the XRD pattern obtained using a XRD-6000 (Japan) X-ray diffractometer in the diffraction angle range of 5°–80° with Cu-Kα radiation (λ = 1.54060 Å). Transmission electron microscopy (TEM) and high-resolution transmission electron microscopy (HRTEM) were performed using a JEM 2010F microscope. TEM images, selected area electron diffraction (SAED) patterns and HRTEM images were obtained using a TECNAI F20 Philips operated at 200 kV. The surface morphology of the samples was studied using field emission scanning electron microscopy (FESEM; S-4700, Hitachi). XPS spectra were obtained on a MultiLab200 with standard MgKα radiation to quantify the elemental composition and surface states of the TiO2 nanostructures. All the spectra were obtained at a working pressure of 10−9 mbar. An XPS survey and high-resolution spectra of C1s, O1s, and Ti2p were collected. The different surface states were obtained in the high resolution Ti2p, O1s and C1s spectra of the samples by specifying line shape, relative sensitivity factor (RSF), peak position, full width at half maxima (FWHM), and area constraints.
2.5 In vitro assays
Cell adhesion and viability assays. These assays were performed by plating 3 × 104 cells in an area of about 1 cm2 TiO2 nanostructures grown substrates placed in tissue culture grade plates. After 4 h, non-adherent cells were washed off and 100 μL of 2 mg mL−1 MTT was added to the remaining cells in each condition, followed by incubation at 37 °C under 5% CO2 for 4 h. After removing the culture media, the reaction was stopped by adding dimethylsulfoxide, and measurements were carried out at 595 nm using an ELISA reader. In the cell viability assay, an MTT assay was performed after 24 h of incubation, as described above, to calculate the percentage of cell viability.
Cell proliferation assay. The assay was performed by plating a similar number of cells for all conditions in triplicate followed by incubation for three different time periods, namely, 24, 48 and 72 h. After each time period, the cell number was calculated using the MTT assay described above.
3. Results and discussion
3.1 Surface morphology and crystallite structure characterization
Fig. 2 shows the FE-SEM images of the time dependent growth of TiO2 nanostructures on FTO grown after 1 h and 3 h. The high resolution FE-SEM images of the TiO2 nanostructures grown after 1 h showed two distinct regions, namely, 1D-nanorod and 3D-nanoflower nanostructures. The top view of the FE-SEM image of the TiO2 nanostructures grown after 3 h showed a uniform coverage of 3D-nanoflowers. From these observations, it appears that 1D-nanorods of TiO2 grow on the substrates as films on which the simultaneous growth of 3D-nanoflowers were initiated. The cross-sectional view of the FE-SEM images of nanostructures obtained after 1 h and 3 h of TiO2 growth demonstrates the formation of 3D-nanoflowers on the vertically aligned 1D-nanorods of TiO2. The surface of the TiO2 nanostructures obtained after 1 h of growth had both 1D-nanorods and 3D-nanoflowers, whereas after 3 h of growth, the surfaces were completely covered by 3D-nanoflowers.
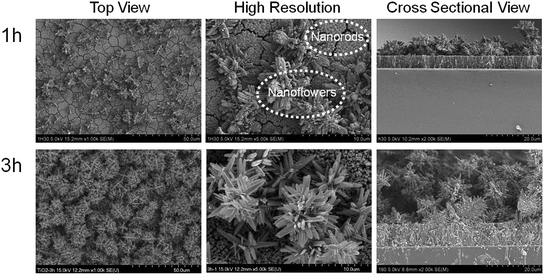 |
| Fig. 2 FE-SEM images of rutile TiO2 nanostructures grown on FTO substrates for two different times (namely, 1 h and 3 h) with a HCl to water ratio of 50 : 50 at 180 °C. | |
The effect of different types of substrates on the growth of the TiO2 nanostructures as films was quantified by the FE-SEM images of the nanostructures grown on glass, ITO and FTO substrates after 3 h. In the FE-SEM images, a uniform distribution of 3D-nanoflowers of TiO2 as films were seen for all the three different types of substrates, as shown in Fig. 3a–c. The higher resolution FE-SEM images of the 3D nano-flower structures show tips of nano-rods of about 300–500 nm on the surface, which are very tightly packed, as shown in Fig. 3d–f. The cross-sectional view of the FE-SEM image shows the formation of a stable interface of 3D nanoflower-like structures on these substrates.
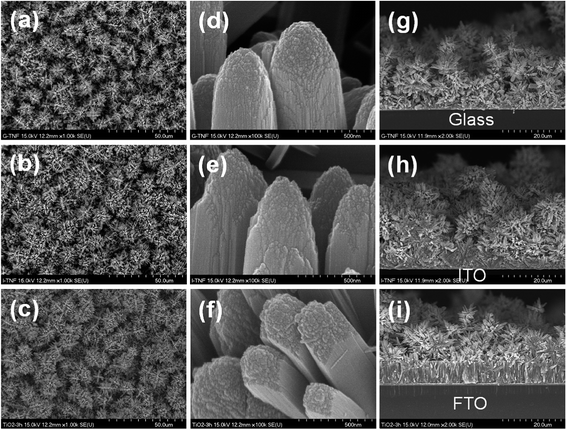 |
| Fig. 3 FE-SEM images of rutile TiO2 nanostructures grown on glass (a), FTO (b) and ITO (c) substrates with a HCl to water ratio of 50 : 50 and reaction time of 3 h at 180 °C. | |
A TEM image of a tip of the TiO2 3D-nanoflowers on FTO substrates was obtained at different magnification, and the results are shown in Fig. 4. The presence of densely packed TiO2 as nanorods was seen in TEM images. The diffraction pattern of TiO2 was obtained and the lattice spacing (d) of TiO2 was calculated. The results showed d001 = 0.23 nm along the longitudinal axis direction pertaining to the d-spacing of the rutile TiO2 (001) crystal planes.
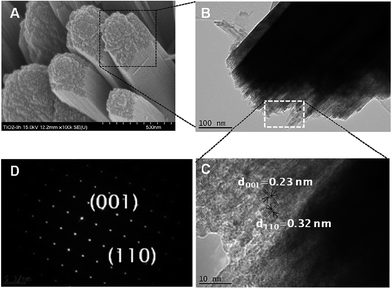 |
| Fig. 4 (A) FE-SEM image of TiO2 nanostructures grown on FTO substrates with a HCl to water ratio of 50 : 50 at 180 °C for 3 h. TEM images (B and C) of the top-view of the TiO2 nanostructures, as seen in the high resolution FE-SEM image (A). (D) Diffraction pattern of TiO2 nanostructures grown under similar conditions. | |
Fig. 5A shows the XRD pattern of the TiO2 nanostructures grown on FTO substrates after 1 h and 3 h. The FTO substrate shows several strong peaks for Sn at the (110), (002), (112), (202) and (113) planes in the XRD spectra (Fig. 5A(a)), which is in accordance with previously obtained spectra.39 The XRD pattern of the FTO substrate and TiO2 nanostructures as films grown on FTO after 1 h was similar. This may be observed due to the fact that the TiO2 film does not grow considerably; thus, the TiO2 content was not detected by XRD in the presence of a strong background of FTO substrate. TiO2 nanostructures obtained at higher time points (at 3 h) showed XRD peaks of TiO2 at 2 theta values of 27.62, 36.28, 41.42, 44.16, 54.62, 56.72, 63.02, and 69.28. These peaks were assigned the (hkl) values of (110), (101), (111), (210), (211), (220), (002) and (301), respectively. All the diffraction peaks were marked to the pure tetragonal rutile phase of TiO2 (JCPDS no. 21-1276). The XRD patterns of the TiO2 nanostructures grown on FTO, ITO and glass substrates are shown in Fig. 5B. The XRD peaks appear at 2 theta values of 27.62, 36.28, 41.42, 44.16, 54.62, 56.72, 63.02, and 69.28. The XRD pattern of TiO2 nanostructures as films grown on the three substrates after 3 h seem to be identical. These peaks were assigned the (hkl) values of (110), (101), (111), (210), (211), (220), (002) and (301), respectively.
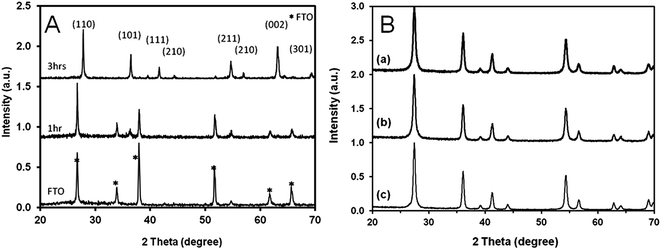 |
| Fig. 5 (A) XRD pattern of TiO2 crystallite growth on FTO with a HCl to water ratio of 50 : 50 at 180 °C for two different times (1 h and 3 h). Prominent diffraction peaks in the XRD pattern of FTO substrate are marked with ‘*’. (B) XRD pattern of TiO2 crystallite growth on glass (a), FTO (b) and ITO (c) substrates with a HCl to water ratio of 50 : 50 at 180 °C for 3 h. | |
3.2 Characterization of the surface chemical functionalities
The XPS spectra of the TiO2 nanostructure surfaces were obtained after 1 h and 3 h on FTO for the quantification of the surface elemental analysis. The %proportions of oxygen (O) to titanium (Ti) atoms were obtained from the wide scan XPS spectra, and the results are shown in Fig. 6. The O/Ti value was 3.1 for the TiO2 nanostructure surfaces obtained after 1 h, which was reduced to 2.7 for the nanostructure obtained after 3 h. The reduction in O/Ti values after a longer reaction time may correspond to a decrease in the %proportion of oxygen atom on the surface. This observation also indicates that titania atoms are tightly packed on the surface, which was also confirmed by FE-SEM and TEM observations.
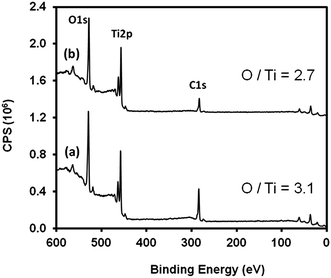 |
| Fig. 6 Wide scan XPS spectra of TiO2 nanostructure growth on FTO substrates with a HCl to water ratio of 50 : 50 at 180 °C for two different reaction times of (a) 1 h and (b) 3 h. | |
High resolution Ti2p and O1s XPS spectra are shown in Fig. 7A and C. The details for the peak fitting parameters for Ti2p and O1s are given in Fig. 7B and D.16 A small increase in the Ti4+ surface states of Ti2p was observed on the surface of TiO2 obtained after 3 h. The relative increase in the proportion of Ti2p surface states indicates the relative decrease in the number of oxygen atoms surrounding the Ti atoms in these nanostructure assemblies. The relative proportion of the different oxidation states of oxygen in the O1s XPS spectra at the surface of TiO2 were obtained after 1 h and 3 h, and the results are shown in Fig. 7. Oxygen atoms as O = (O2−) surface states were increased with an increasing reaction time. These observations are consistent with the atomic %proportion obtained from wide scan XPS spectra.
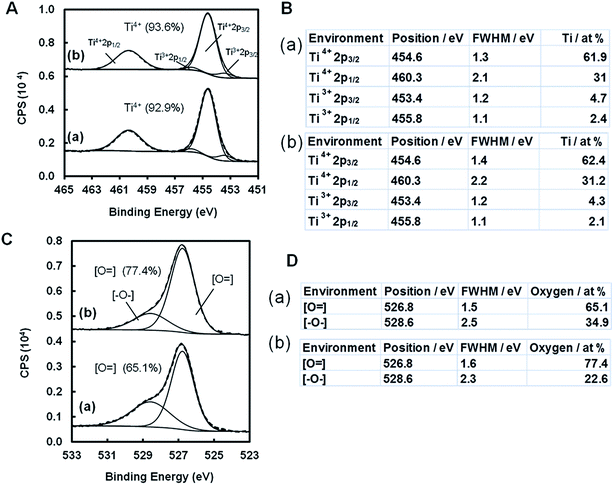 |
| Fig. 7 (A) High resolution peak fitted Ti2p XPS spectra and (C) high resolution peak fitted O1s XPS spectra for TiO2 nanosurfaces prepared with a HCl to water ratio of 50 : 50 at 180 °C for two different reaction times. (B) Peak fitting parameter for Ti2p high resolution XPS spectra and (D) peak fitting parameter for O1s high resolution XPS spectra. (a) and (b) represent 1 h and 3 h of TiO2 nanostructure growth on FTO substrates, respectively. | |
The XPS spectra of the nanostructures grown as films on ITO, FTO and glass substrates were obtained to further quantify the chemical nature of the nanostructures. The wide scan XPS spectra of TiO2 on non-conducting (glass) and conducting (ITO and FTO) substrates are shown in Fig. 8. Detailed elemental analysis and peak fitting for surface state quantification from C1s and Ti2p were carried out as described in our previous studies,27 and the results are shown in Fig. 9 and 10. The higher resolution C1s XPS spectra of the TiO2 film surface prepared on glass and ITO substrates was fitted with six peaks corresponding to different carbon environments including hydrocarbons (C–H/C–C) at 284.6 ± 0.2 eV, (C–C(
O)OX) at 285 ± 0.1 eV, (C–OX) at 286.1 ± 0.2 eV, (C
O/O–C–O) at 287.6 ± 0.2 eV, (C(
O)OX) at 289.2 ± 0.2 eV and C–Ti at 283 ± 0.2 eV. The TiO2 film surface prepared on FTO coated glass substrates was fitted with five peaks corresponding to different carbon environments including hydrocarbons (C–H/C–C) at 284.6 ± 0.2 eV, (C–C(
O)OX) at 285 ± 0.1 eV, (C–OX) at 286.1 ± 0.2 eV, (C
O/O–C–O) at 287.6 ± 0.2 eV and (C(
O)OX) at 289.2 ± 0.2 eV. The TiO2 films on glass showed a spectra shift of 0.53 eV towards the lower binding energy side, which was adjusted to its original position. The bombardment of X-ray photons during XPS analysis caused a positive charge to accumulate on the non-conducting surface. This charge accumulation corresponds to an energy shift of 0.53 eV in the XPS spectra for the TiO2 films grown on non-conducting substrates. There was no spectral shift observed for TiO2 nanostructures grown on conducting (ITO and FTO) glass substrates.
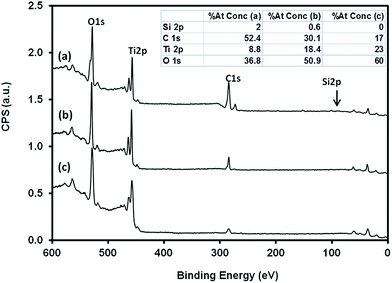 |
| Fig. 8 Wide scan XPS spectra of TiO2 nanosurfaces prepared with a HCl to water ratio of 50 : 50 at 180 °C for 3 h on glass (a), FTO (b) and ITO (c) substrates. | |
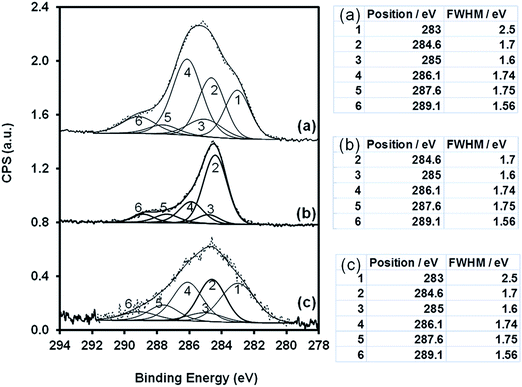 |
| Fig. 9 High resolution C1s peak fitted XPS spectra of TiO2 nanosurfaces prepared with a HCl to water ratio of 50 : 50 at 180 °C for 3 h on glass (a), FTO (b) and ITO (c) substrates. | |
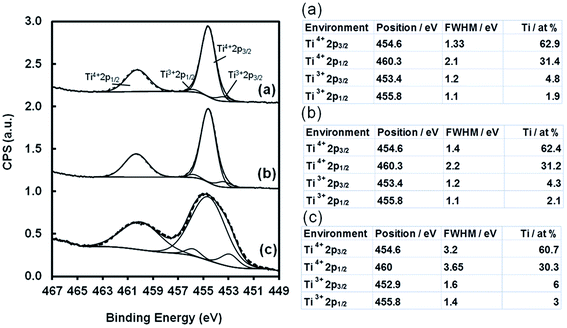 |
| Fig. 10 High resolution Ti2p XPS spectra of the TiO2 nanosurfaces prepared with a HCl to water ratio of 50 : 50 at 180 °C for 3 h on glass (a), FTO (b) and ITO (c) substrates. | |
Different titanium oxidation states were quantified in the high resolution Ti2p XPS spectra of the TiO2 nanostructures grown on ITO, FTO and glass substrates. The spectra were fitted with four peaks including Ti3+2p3/2 at 453.2 ± 0.4 eV, Ti4+2p3/2 at 454.6 ± 0.2 eV, Ti3+2p1/2 at 455.8 ± 0.4 eV and Ti4+2p1/2 at 460.3 ± 0.2 eV. There was not much change in the surface states of Ti2p obtained for the TiO2 nanostructures grown on glass and FTO substrates. TiO2 nanostructures grown on an ITO substrate showed a significant increase in the Ti3+ surface state of Ti2p.
The relative variation in the %proportion of the different surface states of C1s and Ti2p with the different types of nanostructured film prepared on ITO, FTO and glass substrates are shown in Fig. 11. Mainly, five different types of carbon surface states, namely, C/C–H, C–C(
O)OX, C–OX, C
O and C(
O)OX, were observed in the TiO2 films on all the three substrates. Herein, we are interested to see the relative variation for three active functional groups, namely, C–OX, C
O and C(
O)OX, present on the nanosurface obtained on ITO, FTO and glass substrates. The %proportion of hydroxyl functional groups on the surface of the TiO2 nanostructures was significantly increased when the substrates were changed to glass, FTO and ITO for the growth of TiO2. There was no significant change in the %proportion of carbon atoms as C
O in C1s at the surface of the TiO2 nanostructures grown on the different types of substrates. The TiO2 nanostructures grown on glass substrates showed the highest levels of carboxylic functional groups. There was not much difference in the %proportion of carboxylic functionality on the TiO2 nanostructured surfaces on ITO and FTO substrates. An increase in the Ti3+ surface states of Ti2p was obtained from the TiO2 nanostructures grown on ITO substrates. Moreover, there was not much difference in the Ti3+ surface states of Ti2p of TiO2 nanostructures obtained on glass substrates and FTO coated conducting glass substrates.
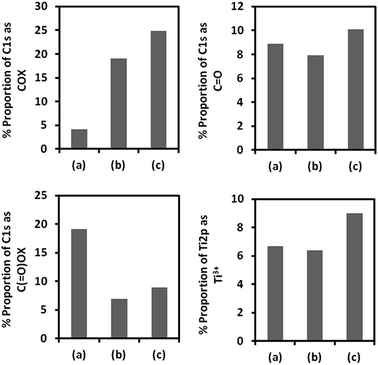 |
| Fig. 11 Relative variation in the %proportion of (A) COX in C1s, (B) CO in C1s, (C) C( O)OX in C1s and (D) Ti3+ in the Ti2p XPS spectra of the TiO2 nanosurfaces prepared with a HCl to water ratio of 50 : 50 at 180 °C for 3 h on glass (a), FTO (b) and ITO (c) substrates. | |
3.3 Biocompatibility of TiO2 nanostructures
Cell adhesion, viability and proliferation assays using MC3T3 osteoblast and HS-5 cells were carried out on the TiO2 nanostructure surfaces obtained on FTO after 1 h and 3 h, and the results are shown in Fig. 12. An increase in %cell adhesion on both the types of nanostructures was obtained as compared to the control (tissue culture plastic surfaces). A combination of 1D-nanorod and 3D-nanoflower-like structures of TiO2 provide better entrapping of cells, and thus the highest percentage of cell adhesion was obtained. The cell viability did not show a significant variation on the two different types of nanostructures. The cell proliferation was also a little less on these structures as compared to the control. The percentage of cell adhesion of HS-5 cells was similar on both the types of TiO2 nanostructures. The viability of HS-5 cells was increased on the TiO2 nanostructure with 1D-nanorod/3D-nanoflowers as compared to the control condition. However, the cell proliferation was reduced on these two types of nanostructures.
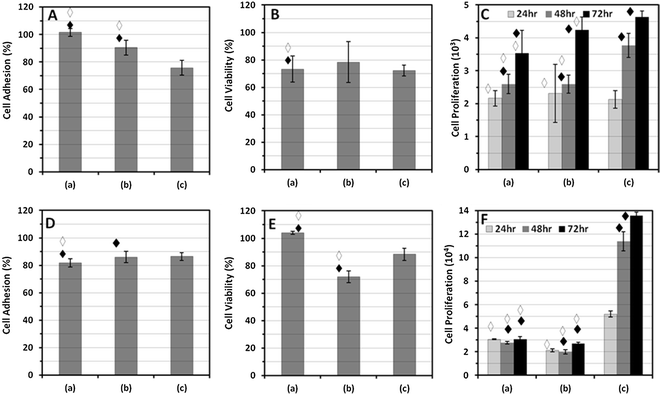 |
| Fig. 12 Cell adhesion assay carried out using MTT on TiO2 nanostructures grown at (a) 1 h and (b) 3 h on FTO substrates and (c) tissue culture plastic surfaces. (A) The percentage of osteoblast cell adhesion. The significance values between the groups were determined: ◆ represents p < 0.05 for conditions (a) and (b) with respect to condition (c); ◇ represents p < 0.05 for condition (a) with respect to condition (b). (B) The percentage of osteoblast cell viability. ◆ represents p < 0.05 for condition (a) with respect to condition (c); ◇ represents p < 0.05 for condition (a) with respect to condition (b). (C) Osteoblast cell proliferation. ◆ represents p < 0.05 for conditions (a), (b) and (c) at different times of cell proliferation. ◇ represents p < 0.05 for condition (a) and condition (b) with respect to condition (c) at the same cell proliferation time points. (D) The percentage of HS-5 cell adhesion. ◆ represents p < 0.05 for condition (a) and condition (b) with respect to condition (c); ◇ represents p < 0.05 for condition (a) with respect to condition (b). (E) The percentage of HS-5 viability. ◆ represents p < 0.05 for condition (a) with respect to condition (c); ◇ represents p < 0.05 for condition (a) with respect to condition (b). (F) HS-5 cell proliferation. ◆ represents p < 0.05 for conditions (a), (b) and (c) at different cell proliferation times. ◇ represents p < 0.05 for conditions (a) and (b) with respect to condition (c) at the same cell proliferation time. | |
MC3T3 cell adhesion, viability and proliferation assays were carried out on TiO2 nanostructure films grown on FTO, ITO and glass substrates, and the results are shown in Fig. 13. An overall increase in %cell adhesion on the TiO2 nanostructures grown on all three different types of substrates was observed as compared to the tissue culture plastic surface. The highest %cell adhesion was obtained on 3D nanoflower structures of TiO2 grown on a glass surface. There was a relative decrease in the %cell adhesion on TiO2 3D-nanoflowers grown on ITO and FTO as compared to the response observed on nanostructures grown on glass. A similar response was observed for %cell viability. Osteoblast cell proliferation on TiO2 3D nanoflowers grown on ITO and FTO was less as compared to the control, despite having a higher %cell adhesion. The XPS analysis of TiO2 films on non-conducting glass substrates showed a spectral shift towards the lower binding energy side. This observation indicates that the TiO2 films grown on non-conducting glass surfaces can retain the charge, whereas the TiO2 films grown on conducting (ITO and FTO) substrates do not show charge accumulation. TiO2 3D-nanoflowers grown on the non-conducting glass substrate showed a higher cell proliferation rate as compared to the control conditions, whereas a decrease in cell proliferation was observed on TiO2 3D nanoflowers grown on ITO and FTO. Therefore, the charge nature of substrate plays an important role in determining the cellular response, despite the similar nanostructure of the same material.
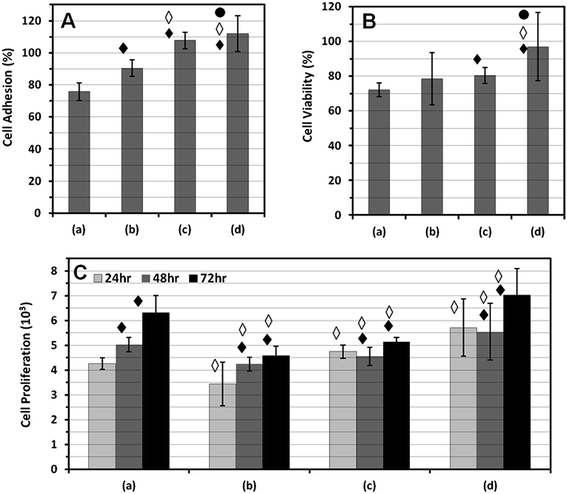 |
| Fig. 13 Osteoblast cell response were observed on (a) tissue culture plastic surfaces and 3D nanoflower-like TiO2 nanostructures grown on (b) FTO, (c) ITO and (d) glass substrates at 3 h. (A) Adhesion%, the significance values between the groups were determined: ◆ represents p < 0.05 for conditions (b), (c) and (d) with respect to condition (a); ◇ represents p < 0.05 for conditions (c) and (d) with respect to condition (b); ● represents p < 0.05 for condition (d) with respect to condition (c). (B) Viability%, ◆ represents p < 0.05 for conditions (b), (c) and (d) with respect to condition (a); ◇ represents p < 0.05 for conditions (c) and (d) with respect to condition (b); ● represents p < 0.05 for condition (d) with respect to condition (c). (C) Cell proliferation, ◆ represents p < 0.05 for conditions (a), (b), (c) and (d) with respect to the change in cell proliferation time. ◇ represents p < 0.05 for conditions (b), (c) and (d) with respect to condition (a) at the same cell proliferation time. | |
HS-5 cell adhesion, viability and proliferation assays were carried out on TiO2 nanostructured film grown on FTO, ITO and glass substrates, and the results are shown in Fig. 14. The HS-5 cell adhesion assay showed a lower %cell adhesion on the TiO2 nanostructures grown on FTO substrates as compared to nanostructures grown on ITO and glass substrates. TiO2 nanostructures on non-conducting glass substrates showed the highest %cell adhesion and proliferation for HS-5 cells.
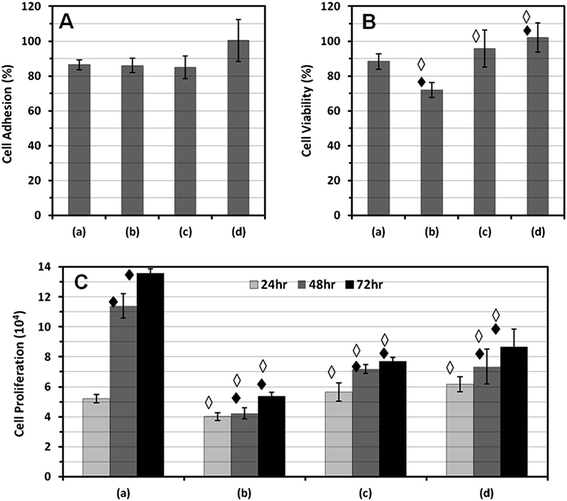 |
| Fig. 14 HS-5 cell response were observed on (a) tissue culture plastic surfaces and 3D nanoflower-like TiO2 nanostructures grown on (b) FTO, (c) ITO and (d) glass substrates at 3 h. (A) Adhesion%, the significance values between the groups were determined: ◆ represents p < 0.05 for conditions (b), (c) and (d) with respect to condition (a); ◇ represents p < 0.05 for conditions (c) and (d) with respect to condition (b); ● represents p < 0.05 for condition (d) with respect to condition (c). (B) Viability%, ◆ represents p < 0.05 for conditions (b), (c) and (d) with respect to condition (a); ◇ represents p < 0.05 for conditions (c) and (d) with respect to condition (b); ● represents p < 0.05 for condition (d) with respect to condition (c). (C) Cell proliferation, ◆ represents p < 0.05 for conditions (a), (b), (c) and (d) with respect to the change in cell proliferation time. ◇ represents p < 0.05 for conditions (b), (c) and (d) with respect to condition (a) at the same cell proliferation time. | |
Despite the fact that the similar nanostructure and crystallite structures of TiO2 were grown on the conducting and non-conducting surfaces, the improved adhesion and proliferation response of both the cell types on the non-conducting surfaces confirms that the cells prefer a non-conducting surface. This may enable the cells to create and sustain a local surface charge environment, which is induced during the cell–surface interactions, for better cell growth on non-conducting surfaces; this is not possible on conducting surfaces.
4. Conclusions
The ordered growth of nanorod (1D)/nanoflower (3D) structures of TiO2 was achieved on non-conducting and conducting (FTO and ITO coated) glass substrates. We used a surfactant-free method for the simultaneous growth of 1D/3D nanorod and nanoflower structures with better adhesion at the interface. TiO2 nanostructures obtained at higher time points (after 3 h) on FTO, ITO and glass substrates exhibit a rutile phase. The findings show that the mixed 1D-nanorod/3D-nanoflower-like structures show better cell adhesion as compared to only 3D-nanoflower-like structures. Cell viability and proliferation rate was higher on TiO2 3D-nanoflowers grown on a non-conducting surface as compared to that grown on a conducting substrate.
Acknowledgements
KHP thanks the Basic Science Research Program for supporting this research through the National Research Foundation of Korea (NRF), funded by the Ministry of Science, ICT and Future Planning (2012R1A1A3010655). MD is thankful to the network project (Bioceramics) of Council of Scientific and Industrial Research (CSIR), India for financial support. We are thankful to Dr Vijay Kumar (NIMS, Hyderabad) for permitting the use of an ELISA reader.
References
- A. Tiwari, M. L. Tursky, D. Mushahary, S. Wasnik, F. M. Collier, K. Suma, M. A. Kirkland and G. Pande, Ex vivo expansion of haematopoietic stem/progenitor cells from human umbilical cord blood on acellular scaffolds prepared from MS-5 stromal cell line, J. Tissue Eng. Regener. Med., 2013, 7(11), 871–883 CrossRef CAS PubMed.
- M. Goerner, B. Roecklein, B. Torok-Storb, S. Heimfeld and H. P. Kiem, Expansion and transduction of nonenriched human cord blood cells using HS-5 conditioned medium and FLT3-L, J. Hematother. Stem Cell Res., 2000, 9(5), 759–765 CrossRef CAS PubMed.
- C. N. R. Rao and A. K. Cheetham, Science and technology of nanomaterials: current status and future prospects, J. Mater. Chem., 2001, 11, 2887–2894 RSC.
- S. T. Aruna and A. S. Mukasyan, Combustion synthesis and nanomaterials, Curr. Opin. Solid State Mater. Sci., 2008, 12(3–4), 44–50 CrossRef CAS PubMed.
- P. Yang, D. Zhao, D. I. Margolese, B. F. Chmelka and G. D. Stucky, Generalized syntheses of large-pore mesoporous metal oxides with semicrystalline frameworks, Nature, 1998, 396, 152–155 CrossRef CAS PubMed.
- J. Li, S. Tang, L. Lu and H. C. Zeng, Preparation of Nanocomposites of Metals, Metal Oxides, and Carbon Nanotubes via Self-Assembly, J. Am. Chem. Soc., 2007, 129(30), 9401–9409 CrossRef CAS PubMed.
- S. V. Agrawal, S. S. Reddy and M. Dhayal, Ultra small gold nanoparticles synthesis in aqueous solution and their application in fluorometric collagen estimation using bi-ligand functionalisation, RSC Adv., 2014, 4(35), 18250–18256 RSC.
- H. S. Nalwa, Encyclopedia of nanoscience and nanotechnology, American Scientific Publisher, USA, 2011 Search PubMed.
- M. Dhayal, R. Kapoor, P. G. Sistla, C. Kant, R. R. Pandey, Govind, K. K. Saini and G. Pande, Ni doped TiO2 thin films on borosilicate glass enhance in vitro growth and differentiation of osteoblasts, J. Biomed. Mater. Res., Part A, 2012, 100(5), 1168–1178 CrossRef PubMed.
- A. J. Maira, K. L. Yeung, C. Y. Lee, P. L. Yue and C. K. Chan, Size effects in gas-phase photo-oxidation of trichloroethylene using nanometer-sized TiO2 catalysts, J. Catal., 2000, 192, 185–196 CrossRef CAS.
- R. Carbone, I. Marangi, A. Zanardi, L. A. Giorgetti, E. Chierici, G. Berlanda, A. Podesta, F. Fiorentini, G. Bongiorno, P. Piseri, P. G. Pelicci and P. Milani, Biocompatibility of cluster-assembled nanostructured TiO2 with primary and cancer cells, Biomaterials, 2006, 27, 3221–3229 CrossRef CAS PubMed.
- M. Dhayal, R. Kapoor, P. G. Sistla, R. R. Pandey, S. Kar, K. K. Saini and G. Pande, Strategies to prepare TiO2 thin films, doped with transition metal ions, that exhibit specific physicochemical properties to support osteoblast cell adhesion and proliferation, Mater. Sci. Eng., C, 2014, 37, 99–107 CrossRef CAS PubMed.
- W. Zhao, C. Chen, W. Ma, J. Zhao and Z. Shuai, Efficient degradation of toxic organic pollutants with Ni2O3/TiO(2−x)Bx under visible irradiation, J. Am. Chem. Soc., 2004, 126, 4782–4783 CrossRef CAS PubMed.
- M. Mazaheri, A. M. Zahedi, M. Haghighatzadeh and S. K. Sadrnezhaad, Sintering of titania nanoceramic: Densification and grain growth, Ceram. Int., 2009, 35, 685–691 CrossRef CAS PubMed.
- M. Dhayal, S. D. Sharma, C. Kant, K. K. Saini and S. C. Jain, Role of Ni doping in surface carbon removal and photo catalytic activity of nano-structured TiO2 film, Surf. Sci., 2008, 602, 1149–1154 CrossRef CAS PubMed.
- J. Jun, J. H. Shin and M. Dhayal, Surface state of TiO2 treated with low ion energy plasma, Appl. Surf. Sci., 2005, 252(10), 3871–3877 CrossRef PubMed.
- S. I. Shah, W. Li, C. P. Huang, O. Jung and C. Ni, Study of Nd3+, Pd2+, Pt4+, and Fe3+ dopant effect on photoreactivity of TiO2 nanoparticles, Proc. Natl. Acad. Sci. U. S. A., 2002, 99(2), 6482–6486 CrossRef CAS PubMed.
- Y. Cheng, H. Sun, W. Jin and N. Xu, Photocatalytic degradation of 4-chlorophenol with combustion synthesized TiO2 under visible light irradiation, J. Chem. Eng., 2007, 128, 127–133 CrossRef CAS PubMed.
- P. X. Ma, Biomimetic materials for tissue engineering, Adv. Drug Delivery Rev., 2008, 60, 184–198 CrossRef CAS PubMed.
- X. Liu and C. Ding, Plasma sprayed wollastonite/TiO2 composite coatings on titanium alloys, Biomaterials, 2002, 23, 4065–4077 CrossRef CAS.
- D. S. Kommireddy, S. M. Sriram, Y. M. Lvov and D. K. Mills, Stem cell attachment to layer-by-layer assembled TiO2 nanoparticle thin films, Biomaterials, 2006, 27, 4296–4303 CrossRef CAS PubMed.
- H. Zhang, Y. Han, X. Liu, P. Liu, H. Yu, S. Zhang, X. Yao and H. Zhao, Anatase TiO2 microspheres with exposed mirror-like plane {001} facets for high performance dye-sensitized solar cells (DSSCs), Chem. Commun., 2010, 46, 8395–8397 RSC.
- R. R. Pandey, K. K. Saini and M. Dhayal, Using Nano-arrayed structures in sol–gel derived Mn2+ doped TiO2 for high sensitivity urea biosensor, J. Biosens. Bioelectron., 2010, 1, 101 CAS.
- Y. Li, Q. Sun, X. Sun and L. Dong, Synthesis of nanocoral structured TiO2 and its photoelectrical performance in dye sensitized solar cells, ECS Trans., 2013, 53, 57–63 CrossRef PubMed.
- L. L. Li, Y. J. Chen, H. P. Wu, N. S. Wang and E. W. G. Diau, Detachment and transfer of ordered TiO2 nanotube arrays for front-illuminated dye-sensitized solar cells, Energy Environ. Sci., 2011, 4, 3420–3425 CAS.
- J. Qu and C. Lai, One-dimensional nanostructures as photoanodes for dye-sensitized solar cells, J. Nanomater., 2013, 2013, 762730 Search PubMed.
- M. Adachi, Y. Murata, J. Takao, J. Jiu, M. Sakamoto and F. Wang, Highly efficient dye-densitized solar cells with a titania thin-film electrode composed of a network structure of single-crystal-like TiO2 nanowires made by the “oriented attachment” mechanism, J. Am. Chem. Soc., 2004, 126, 14943–14949 CrossRef CAS PubMed.
- M. Dhayal, S. I. Cho, J. Y. Moon, S. J. Cho and A. Zykova, S180 cell growth on low ion energy plasma treated TiO2 thin films, Appl. Surf. Sci., 2008, 254, 3331–3338 CrossRef CAS PubMed.
- K. N. Pandiyaraj, V. Selvarajan, M. Pavese, P. Falaras and D. Tsoukleris, Investigation on surface properties of TiO2 films modified by DC glow discharge plasma, Curr. Appl. Phys., 2009, 9, 1032–1037 CrossRef PubMed.
- I. Ichinose, H. Senzu and T. Kunitake, Stepwise adsorption of metal alkoxides on hydrolyzed surfaces: A surface sol–gel process, Chem. Lett., 1996, 25, 831–832 CrossRef.
- B. D. Boyan, T. W. Hummert, D. D. Dean and Z. Schwartz, Role of material surfaces in regulating bone and cartilage cell response, Biomaterials, 1996, 17, 137–146 CrossRef CAS.
- R. R. Randey, M. Dhayal and K. K. Saini, Engineering electrochemical response of TiO2-based enzymatic biosensors by aliovalent cation doping, Adv. Electrochem. Sci. Eng., 2013, 1(1), 62–66 CrossRef PubMed.
- R. R. Randey, M. Dhayal and K. K. Saini, Modification of titanium surface states by ruthenium to enhance electrochemical response of TiO2 thin films for biosensor application, Adv. Electrochem. Sci. Eng., 2013, 1(2), 55–61 CrossRef PubMed.
- K. H. Park and M. Dhayal, Simultaneous growth of rutile TiO2 as 1D/3D nanorod/nanoflower on FTO in one-step process enhances electrochemical response of photoanode in DSSC, Electrochem. Commun., 2014, 49, 47–50 CrossRef CAS PubMed.
- B. Vagaska, L. Bacakova, E. Filova and K. Balik, Physiol. Res., 2010, 59, 309–322 CAS.
- W. H. Chen, Y. Tabata and Y. W. Tong, Curr. Pharm. Des., 2010, 16, 2388–2394 CrossRef CAS.
- C. J. Wilson, R. E. Clegg, D. I. Leavesley and M. J. Pearcy, Tissue Eng., Part A, 2005, 11, 1–18 CrossRef CAS PubMed.
- M. Trinadh, G. Kannan, T. Rajasekhar, A. V. S. Sainath and M. Dhayal, Synthesis of glycopolymers at various pendant spacer lengths of glucose moiety and their effects on adhesion, viability and proliferation of osteoblast cells, RSC Adv., 2014, 4(70), 37400–37410 RSC.
- K. H. Park, R. R. Pandey, C. K. Hong, K. K. Saini and M. Dhayal, Electrochemical characterization of enzymatic organo-metallic coating of TiO2 nanoparticles, Sens. Actuators, B, 2014, 196, 589–595 CrossRef CAS PubMed.
|
This journal is © The Royal Society of Chemistry 2015 |