DOI:
10.1039/C4RA14977G
(Review Article)
RSC Adv., 2015,
5, 36240-36261
Stability of biodiesel, its improvement and the effect of antioxidant treated blends on engine performance and emission
Received
21st November 2014
, Accepted 30th March 2015
First published on 9th April 2015
Abstract
Biodiesel consists of long chain fatty acid esters derived from vegetable oils, animal fats, and used oils. Biodiesel contains different types, amounts, and configurations of unsaturated fatty acids, which are prone to oxidation. Biodiesel stability is affected by its interaction with atmospheric oxygen, light and temperature, storage conditions, and factors causing sediment formation. It can be classified broadly into three types: oxidation stability, thermal stability, and storage stability. Oxidative degradation occurs in biodiesel upon aerobic contact during storage, as well as upon contact with metal contaminants. Thermal instability focuses on the oxidation rate at higher temperatures, which is characterized by the formation of insolubles and increase in the weight of oil and fat. Storage stability is concerned with interaction between the physical and chemical characteristics of biodiesel with environmental factors, such as light, metal contamination, color changes, and sediment formation. Antioxidant concentration greatly influences engine performance and emission. The BSFC of biodiesel fuel with antioxidants is less than that of fuel without antioxidants. Moreover, an antioxidant can significantly reduce NOx formation during engine operation. Among the available synthetic antioxidants, only three antioxidants (TBHQ, PY, and PG) can significantly increase biodiesel stability. This article presents an overview of the stability of biodiesel, including the methods available for the prediction of its different stability properties. Feasible remedies to improve the stability of biodiesel and the effect of antioxidants in stabilized blends on engine performance and emission are also discussed.
1. Introduction
Biodiesel is defined as a vegetable oil- or animal fat-based diesel fuel consisting of long chain alkyl esters. Biodiesel is produced by chemically reacting lipids (e.g., vegetable oil, animal fat) with an alcohol to produce fatty acid esters.1,2 The fatty acid profile of biodiesel corresponds to that of parent oil or fat, which is a key factor that influences its fuel characteristics. The stability of fuel refers to its resistance to the degradation processes that can change its fuel properties and make it inapplicable as a fuel.2,3 A fuel is considered unstable when it undergoes changes, such as oxidation or autoxidation in the presence of oxygen in ambient air, thermal or thermal-oxidative decomposition because of heat, hydrolysis when in contact with water or moisture in tanks and fuel lines, microbial contamination from water droplets containing bacteria or fungi, or migration of dust particles into the fuel.4,5
The stability of biodiesel includes the aspects of oxidation, thermal, and storage stability. Oxidation stability is the tendency of fuels to react with oxygen at ambient temperature.6,7 Biodiesel degradation prior to combustion in diesel engine is affected by different factors, such as nature of the original lipid feedstock, biodiesel production process, storage and handling conditions, fuel additives and impurities, conditions within the fuel tank, and fuel distribution system.8–11 Thermal stability involves the measurement of the tendency of a fuel to produce asphaltenes when exposed to high temperature conditions; asphaltenes are tar-like resinous substances generated in the fuel, and these substances plug the fuel filters of engines when used as fuel.12,13 The temperature has a significant effect on oxidative degradation because it enhances the rate of degradation. Unstable oxidation products can attack elastomers.14 The oxidation of biodiesel prompts the development of hydroperoxides, which can assault elastomers or polymerize to form insoluble gums. Oxidation products, such as hydroperoxides and carboxylic acids, can function as plasticizers of elastomers.15
Storage stability describes the general stability of the fuel under long-term storage. Oxidative degradation is perhaps one of the initial concerns of storage stability, but microbial growth and water contamination are definite issues of storage stability in the long run.16
Several previous studies have already investigated the oxidation, thermal, and storage stability of biodiesel.17–19 Few review articles have analyzed different aspects of biodiesel stability together with the effects of oxidation inhibitors on engine performance and emission.1,20–23 Several test methods have been devised to measure the stability of biodiesel; these methods involve the treatment of fatty oil or ester under elevated temperature, time, and oxygen exposure while measuring one or more oxidation-sensitive properties, such as peroxide value, insolubles, evolution of volatile short chain fatty acids, or heat of reaction.20,24,25 However, no simple stability test or single stability parameter currently exists to sufficiently indicate all the stability features of biodiesel fuel. A single new test that can completely define biodiesel stability is highly improbable because different tests have various functions. The present paper attempts to review the work conducted on the oxidation, thermal, and storage stability of biodiesel, various test methods, and improvement of biodiesel stability. This article focuses on a comprehensive study of three different aspects of biodiesel oxidation stability, the methods applied to improve it, effect of oxidation inhibitors (i.e., antioxidants) on stability, and influence of antioxidant-treated blend on diesel engine performance and emission characteristics.
2. Different aspects of biodiesel stability
Biodiesel stability is affected by interactions with atmospheric oxygen, light, temperature, storage conditions, and factors causing sediment formation.26 Biodiesel produced from vegetable oils and other feedstocks possess lower stability compared with petroleum-based diesel because of the unsaturated fatty acid content, such as linoleic and linolenic acids, on the fatty acid profile of the parent feedstock.27 Biodiesel stability depends on different fatty acid compositions. Most plant-derived fatty oils contain poly-unsaturated fatty acids that are methylene-interrupted rather than conjugated. This structural property is essential to the understanding of the stability. Thus, the instability of biodiesel can be divided into three aspects, namely, oxidative, thermal, and storage instability. The instability of biodiesel is dependent on the quantity and configuration of the olefinic unsaturation in the fatty acid chains.
2.1. Oxidation stability
The oxidation of fatty acid chain is a complex method because of its various applications.28 The oxidation of biodiesel is caused by unsaturation in fatty acid chain and existence of double bonds in the fatty acid molecule, which exhibits high levels of reactivity with O2, particularly when exposed to air or water. Unsaturated fatty compounds are used to mitigate oxidation stability, because low amounts of more highly unsaturated fatty compounds have a disproportionately strong effect in reducing oxidation stability.29 Hence, the oxidation mechanism can be explained by two categories, namely, primary oxidation and secondary oxidation. Numerous researchers have investigated the chemistry of primary and secondary oxidation.2,9,10,14,30–48 Several studies have reported that vinyl polymerization involves higher molecular weight oligomers of fatty oils or ester formation.16,34 Primary oxidation occurs through a set of reactions categorized as initiation, propagation, and termination.20 As shown in Fig. 1, the first set includes the elimination of hydrogen from a carbon atom to make a carbon free radical. If diatomic oxygen is present, the consequent reaction to form a peroxy radical becomes enormously fast, even not allowing substantial alternatives for the carbon-based free radical.35,36 Carbon free radicals are more active than peroxy free radicals. However, peroxy free radical is adequately reactive to fast abstract hydrogen atom to form another carbon radical and hydroperoxide (ROOH). The newly formed free carbon radical can react with diatomic oxygen and continue the propagation cycle.
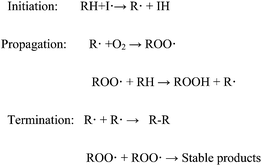 |
| Fig. 1 Basic oxidation reaction. | |
During the induction period, the ROOH residue concentrations remains low until a certain time interval, and the oxidation stability of fatty acid or biodiesel can be determined under stress conditions.5
For the whole oxidation system, the ROOH level increases very quickly until the initial period is reached.2 During the initial period, ROOH can directly or indirectly change the properties of fatty oils and biodiesels.32 The maximum level of ROOH forms at 300–400 meq O2 per kg at any ROOH concentration profile peak, although the level of higher ROOH has been investigated.49 The fatty acid reacts with the molecular oxygen and produces unstable peroxide radical (ROO˙), which further reacts with the original substrate RH. The transfer of a hydrogen atom from fatty acids to a peroxide radical will result in the formation of a fatty acid hydroperoxide (ROOH). The radical chain reaction is shown in eqn (1), in which the reaction with oxygen results in the formation of a new fatty acid radical (R˙), because of the addition of fatty acid hydroperoxide (ROOH) and self-sustaining chain reaction.
|
ROO˙ + RH → ROOH + R˙
| (1) |
The termination step is achieved when two free radicals react and form stable products, as shown in the following equations.
|
ROO˙ + ROO˙ → stable products
| (3) |
When an adequate concentration of radical species is available, the probability of two radicals colliding is very high.11 Peroxyl radicals (ROO˙) can produce peroxyl-linked molecules (R–OO–R) and liberating oxygen as follows in reaction (4)
|
ROO + ROO → R–OO–R + O2
| (4) |
The ROOH concentration remains very low during the primary period of oxidation until a certain time interval, and this time period is often referred to as the induction period (IP), the presence of temperature and oxygen pressure during IP is identified by the comparative exposure to oxidation of TAG or alkyl ester, thereby signaling the onset of rapid oxidation; the ROOH level rapidly increases when the IP has elapsed.11 The hydroperoxide (ROOH) levels can either peak and then decrease or increase and plateau at a steady state as oxidation progresses. Although issues, such as extent of earlier oxidation, temperature, oxygen availability, and incidence of metal catalysts, are likely involved in these phenomena, the explanations for the two different activities remain unclear. ROOH disintegration continues because of a peak in ROOH concentration. Insufficient levels of oxygen can slow or even stop ROOH formation. Similarly, different factors, such as higher temperature and presence of hydroperoxide decomposing metal catalysts (e.g., copper and iron), which increase the ROOH decomposition rate, can influence ROOH concentration. At 300–400 meq O2 per kg, maximum ROOH levels typically form2,11 although higher ROOH levels have been observed11
Numerous secondary oxidation products, including short chain carboxylic acids, alcohols, high molecular weight oligomers, and aldehydes, form even at ambient temperature during the secondary oxidation stages, whereas hydroperoxides (ROOH) continue to decompose and interact.50 The secondary products are produced in different ways. Several studies reported various secondary oxidation products observed from different experiments using biodiesel, such as 25 different aldehyde compounds during vegetable oil oxidation, including hexenals, heptenals, propane, pentane, and 2,4-heptadienal.24,51 Polymeric species form with the involvement of fatty acid chains. Trimers or tetramers are smaller than polymeric spices,51 although the open literature does not explain the reason for this difference. Polymer formation increases viscosity. C–O–C and C–C linkages produce fatty acids, esters, and aliphatic alcohols. Hasenhuettle51 explained the decomposition mechanism of hydroperoxides to shorter chain fatty acids, such as formic acid. Table 1 demonstrates the oxidation stability or IP and unsaturated fatty acid composition for methyl esters of distinctive oils. These data were collected from the literature, and the details of oxidation of a specific biodiesel (ethyl linoleate ester) are illustrated in Fig. 2. Step 1: hydrogen abstraction from the allyl group. Step 2: oxygen attack at either end of the radical center, producing intermediate peroxy radicals. Step 3: monohydroperoxide formation. Step 4: partial decomposition of the initially formed monohydroperoxides into oxo-products and water.
Table 1 Fatty acid composition for different biodiesel and their stabilities
Sl. no. |
Type of biodiesel |
Stability (h) |
Saturated fatty acid |
Unsaturated fatty acid |
Ref. |
1 |
Palm |
4.0 |
44.7 |
55.3 |
54 |
2 |
Olive |
3.3 |
15.7 |
84.4 |
54 |
3 |
Peanut |
2.0 |
15.6 |
88.4 |
40 |
4 |
Rape |
2.0 |
6.5 |
93.6 |
40 |
5 |
Soybean |
1.3 |
15.3 |
84.7 |
40 |
6 |
Sunflower |
0.8 |
11.1 |
88.9 |
40 |
7 |
Grape |
0.5 |
11.3 |
88.5 |
40 |
8 |
H.O. sunflower |
1.2 |
9.3 |
90.5 |
40 |
9 |
Almond |
3.0 |
13.9 |
86.4 |
40 |
10 |
Corn |
1.2 |
8 |
91.7 |
40 |
11 |
Crude palm |
25.7 |
50.6 |
43.4 |
55 |
12 |
Distilled palm |
3.52 |
48.73 |
51.27 |
41 |
13 |
Used frying |
3.42 |
20.1 |
79.9 |
56 |
14 |
Spent bleaching earths |
14.59 |
41.4 |
58.6 |
42 |
15 |
Palm |
23.33 |
83.8 |
9.9 |
42 |
16 |
Croton |
4.04 |
9.6 |
90.3 |
57 |
17 |
Moringa |
5.05 |
21.6 |
78.3 |
43 |
18 |
Jatropha |
10.43 |
22.4 |
77.6 |
43 |
19 |
Undistilled rapseed |
9.1 |
9.69 |
90.32 |
58 |
20 |
Distilled rapseed |
3.4 |
6.23 |
93.64 |
44 |
21 |
Sunflower |
3.4 |
11.7 |
87.92 |
44 |
22 |
Distilled sunflower |
1.2 |
11.42 |
88.58 |
44 |
23 |
Undistilled used frying |
5.9 |
20.25 |
77.6 |
44 |
24 |
Distilled used |
3.5 |
17.17 |
80.94 |
44 |
25 |
Beef tallow |
1.2 |
41.11 |
55.76 |
44 |
26 |
Distilled tallow |
0.4 |
50.4 |
45.01 |
44 |
27 |
Safflower |
0.86 |
10.9 |
89.1 |
59 |
28 |
Jatropha |
3.23 |
21.1 |
78.9 |
48 |
29 |
Pongamia |
2.35 |
16 |
84 |
48 |
30 |
Safflower |
1.73 |
11.6 |
88.4 |
48 |
31 |
Soyabean |
3.8 |
15.5 |
84.5 |
48 |
32 |
Soyabean |
4.87 |
23.3 |
76.7 |
60 |
33 |
Palm |
13.37 |
43.4 |
56.6 |
49 |
34 |
Crude palm |
25.70 |
50.6 |
49.22 |
49 |
35 |
Palm |
15.4 |
83.8 |
9.9 |
61 |
36 |
Soy |
1.3 |
15.3 |
84.7 |
50 |
37 |
Rapseed |
7.8 |
64.5 |
29.7 |
62 |
38 |
Ground nut |
2.0 |
15.6 |
84.4 |
51 |
39 |
Corn |
1.2 |
8.0 |
91.7 |
51 |
40 |
Pongamia |
2.54 |
16.0 |
84 |
51 |
41 |
Castor |
3.18 |
3.49 |
94.58 |
51 |
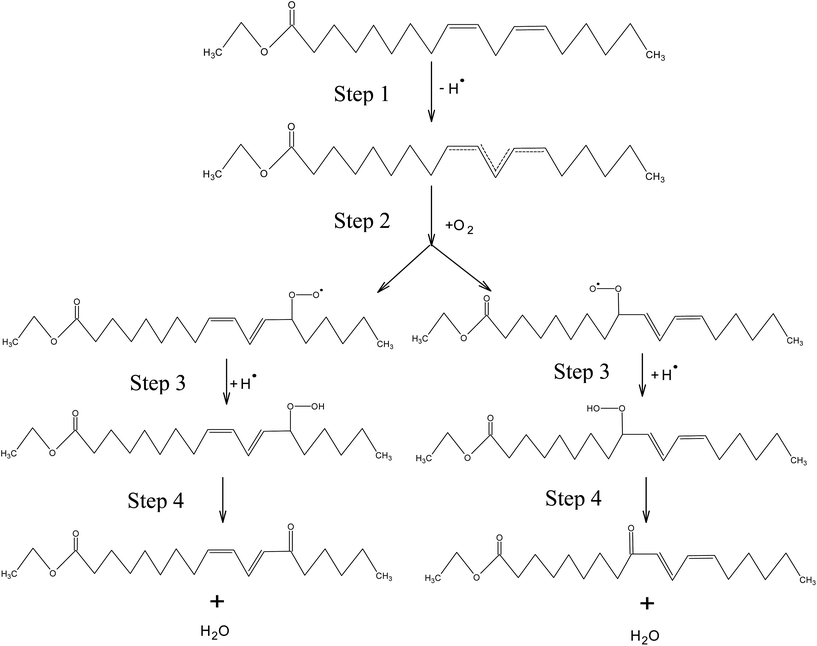 |
| Fig. 2 Scheme of radical oxidation of ethyl linoleate ester.52,53 | |
2.1.1. Characterization of oxidation stability. A number of different matrices are characterized for oxidation stability. Different test processes have been developed to determine oxidation stability. Such test methods are categorized based on their application. A single method for identifying the stability of biodiesel is currently unavailable. Some methods can determine the tendency to oxidize materials, and others can specify the level of oxidation products.2 The relative resistance to oxidation of a fuel can be assessed over a measured time by monitoring oxidation product levels. Additional elaborate tests to accelerate fuel, such as oxidation, are usually conducted to control oxygen exposure at higher temperatures.63 For example, acid levels may be continuously monitored and quantities of filterable insoluble materials may be measured in such tests. Moreover, the rate of the progression of oxidation can be determined.Different techniques can be used for the characterization of oxidation stability depending on the parameter being measured, such as physical properties, initial fatty oil composition, other parameters indicative of relative stability, primary oxidation products, and secondary oxidation products.2 To characterize the oxidation stability of biodiesel, the following techniques can be used2 compositional analysis (gas or liquid chromatography), free and total glycerol content, FFA, various structural indices (such as APE, OX, iodine value, BAPE, and electromagnetic spectroscopy), product levels of primary oxidation (peroxide value), product levels of secondary oxidation (anisidine value, aldehyde content, attendance of quantities of filterable insoluble materials, total acid number and polymer levels), physical properties (density and viscosity), and accelerated oxidation (Rancimat IP or oil stability index and pressurized differential scanning calorimetry). To monitor oxidation progression, few measurements are suitable because the peroxide value initially increases but decreases upon further oxidation as the peroxide reacts to form secondary products. In most cases, the peroxide value increases with time.39 No perfect test method can identify the characteristics of biodiesel, and the likelihood that any one new test will be able to completely define biodiesel stability is very low.11 Therefore, several measurement test methods are used to adequately characterize oxidation stability, and these methods are discussed below.
(a) Analysis of the IR spectra. The usual determination methods of oxidation stability are slow, tedious, and time consuming, as evident from the aforementioned discussions. Comparatively, IR is an easy, simple, and fast technique for sample analysis. Furlan et al.64 investigated biodiesel oxidation using IR spectroscopy.52 In their report, the IR spectra were highly affected by degradation because hydroxyperoxides, alcohol, acids, aldehydes, and ketones formed during oxidation. Given the additional formation of carbonyl groups upon oxidation, monitoring of the band associated with the second harmonic of the carbonyl in the region between 3500 and 3400 per centimeter is helpful to evaluate biodiesel stability. The oxidation of soybean and crambe biodiesel has been analyzed by FTIR measurements, and more carbonyls are formed in soybean biodiesel compared with crambe biodiesel, which shows the less stable nature of soybean biodiesel to thermal stress.9Conceicüão et al.65 investigated the thermal and oxidative degradation of castor oil biodiesel53 based on thermogravimetric and calorimetric profiles. Their group also analyzed spectroscopic data. Castor oil and biodiesel contain ricinoleic acid as the major component, which contains a hydroxyl group and displays additional IR bands at 3440, 850, and 1000 per centimeter. The IR spectrum of degraded samples at 210 °C indicates oxidation by displaying a decrease in the intensity of the bands at 3007 and 724 per centimeter because of the decrease in unsaturation, representing oxidative polymerization. Both near IR (NIR) and middle IR (MIR) spectroscopy are successful in monitoring the quality and stability of biodiesel and its blends with diesel fuel.66–70 In connection with multivariate calibration, MIR and NIR are employed in analyzing the quality of pure biodiesel (B100) and the transesterification reaction.71–75 Multivariate NIR spectroscopy has been used to evaluate biodiesel stability by analyzing various fuel properties, such as the IV, water content, CFPP, kinematic viscosity, methanol content, density, and AV.47,51
(b) The active oxygen method. AOM is widely used in measuring the oxidation stability parameters. It is most suitable method to measure the peroxide value (PV). In this method, the sample is heated at a certain temperature and continuously bubbled at a particular flow rate. The specific PV is measured at a suitable time when oxidation is initiated and exposed to air. AOM76 is one of the oldest methods to determine the stability of biodiesel. It has been used for 60 years with various modifications.77–82 AOM uses a very simple system, in which the oil specimen is heated at an arranged temperature while bubbling waterless air at a fixed rate. The trend in PV varies with time because the peroxides undergo rapid degradation. Thus, PV measurement is not a suitable method for monitoring the oxidation stability. However, AOM is very labor intensive, requires chlorinated solvents, and provides inconsistent findings.
(c) Rancimat method (EN 14112). The Rancimat method is one of the most effective methods for determining the oxidation stability of biodiesel. For the Rancimat method, FAMEs of the samples are initially oxidized to peroxides as the primary oxidation products. To form the secondary oxidation products, the peroxides are completely decomposed. The decomposition products are mainly composed of formic acid, acetic acid, volatile organic compounds, and low molecular weight organic acids. Based on the American Oil Chemists' Society (AOCS), the Rancimat method is also the usual and official method for determining the oxidative stability of oils and fats. In this method, the temperature range is usually limited to a maximum of 130 °C.83 In a brief experiment, the sample is first heated at 110 °C. In this process, the oxidation of the sample occurs because the air is bubbled in the sample, thereby releasing some gases with the air. Subsequently, deionized water is passed in the flask. The flask is connected to an electrode to measure the conductivity of the solution. The IP is measured in this process. In this case, the IP is noted as the time at which the conductivity starts to increase very quickly. The continuous measurement of conductivity results in an oxidation curve. The point of inflection in this curve is known as the IP. Volatile acidic gases, such as formic acid, acetic acid, and other acids, are produced by oxidation and absorbed in water, which is the main reason for the increment in conductivity and IP measurement.17,82,84 A modified Rancimat test can also be used for the determination of the storage stability of samples.85Several writers have investigated the work conducted on oxidation stability using the Rancimat test. The BIOSTAB86 project compared ASTM 2274 and Rancimat test, as show in Fig. 3.
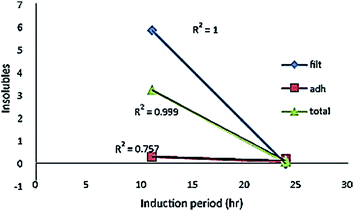 |
| Fig. 3 Comparison between ASTM 2274 and Rancimat induction period.82 | |
This project demonstrated the relationship between the filterable adherent and total insolubles with the IP. Fig. 3 indicates that the total and filterable insolubles exhibit good agreement with the Rancimat IP. For this reason, both methods can be used effectively and interchangeably.
(d) Petro OXY method (ASTM D 7545). Oxidation stability was determined using Petro OXY equipment from Petro test Instruments GmbH & Co. The experimental conditions were the same as those specified by the ASTM D 7545 method (temperature, 140 °C; initial oxygen pressure, 700 kPa; and sample volume, 5 mL). In this method, the oxidation stability of the fuel is directly measured by the time needed to achieve a fixed pressure drop.8
(e) Low pressurized differential scanning calorimetry. The oxidative induction time of biodiesel blends with antioxidants was measured using a pressure differential scanning calorimeter (PDSC; model P-20 Q-DSC from TA Instruments) that was previously calibrated using indium metal as standard.8 PDSC analyses were conducted using an open 110l L platinum pan for sample and reference. Approximately 3.0 mg of sample was employed in each analysis, with static air at 80 psi (551 kPa). The samples were heated from ambient temperature to 110 °C at a heating rate of 10 °C min−1. An isotherm was applied at this temperature until a significant oxidation step of the material occurred.49 This step was indicated by a significant exothermic DSC peak, whose respective onset time is called the oxidation induction time.59
2.2. Thermal stability
Thermal stability refers to the stability of a molecule at high temperatures; a highly stable molecule has more resistance to decomposition or oxidation at high temperatures.3,8,14,26,87,88 At significantly high temperatures, the methylene-separated polyunsaturated olefin structure will begin to isomerize to a more static conjugated structure. Isomerization forms a cyclohexene ring, in which a linked diene group from one fatty acid chain can react with a single olefinic group from another fatty acid chain.89,90 The Diels Alder reaction is a reaction between a conjugated di-olefin and mono-olefin group Fig. 4 that becomes significant at 250–300 °C or higher, and the reaction products formed are called dimers.2,91,92
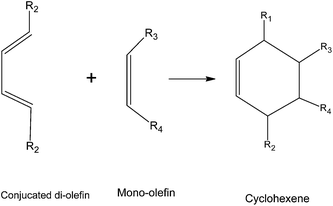 |
| Fig. 4 Diels Alder reaction.93 | |
Hence, the Diels Alder reaction also forms trimers by thermal polymerization with the reaction of an isolated double bond in a dimer side chain with a conjugated diene from another fatty oil.69 However, a recent study found evidence supporting the non-Diels Alder coupling of two side-chain olefin groups from a dimer and fatty oil molecule.32,68 Thermal polymerization is characterized by rapid reduction in total unsaturation as all the three olefin groups become one. At 300 °C, initial polymerization resulted in the dramatic reduction in total unsaturation as measured by IV when linseed oil was thermally polymerized. However, no increase in molecular weight was observed because of an intramolecular Diels Alder reaction between two fatty acid chains in the same triacylglyceride molecule. At temperatures higher than 300 °C, biodiesel produced from used cooking oils when recycled in high-pressure cookers may lead to transesterification to methyl esters and retained linkages. Under such thermal stress, intermolecular dimers also form. Moreover, a di-ester with a molecular weight about twice that of a normal biodiesel ester molecule will be produced. If such biodiesels (i.e., yellow greases) are not purified, these dimers would be present in the final fuel. Nevertheless, no work has reported the incidence of such dimers in recycled cooking oils and if so, their results are mainly on fuel properties of similar non-purified biodiesel fuels. The published literature pertaining to U.S. biodiesel production does not contain the impending existence of such dimeric types in non-purified yellow grease biodiesel.69 Thermal polymerization may be of limited importance in biodiesel, which is heated continuously by the engine in the fuel tank before definite combustion. The storage stability of biodiesel is not influenced by thermal polymerization.12
2.2.1. Characterization of thermal stability. Different methods from various industries, most notably the fuel and lubricant industries, have been used to assess the oxidative and thermal instability of fatty oils. To determine the thermal stability of biodiesel, the following methods are commonly used: ASTM D 6468, which is the standard test method for high temperature stability of middle distillate fuels,94 Rancimat test, which is the procedure specially modified for evaluating thermal stability95 and thermogravimetric analysis/thermal differential analysis (TGA/DTA), which is precise, sensitive, fast, and requires small amounts of samples to measure the thermal stability parameters.96 The following test methods are widely used to characterize the thermal stability of oils.
(a) ASTM D 6468-08. ASTM D 6468-08 is a method that is highly prominent for the high temperature stability determination of middle distillate fuels (including biodiesel). In this method, the sample is aged at 150 °C in open tubes with air contact for approximately 90 or 180 min. After the aging process, the sample is cooled. The insoluble sediments are then filtered and estimated by the light reflectance method of filter paper. For comparison purposes, a blank is prepared without the sample using an unused filter pad.82,97 The filter paper used for this method has a nominal porosity of 11 μm, so it cannot capture all the sediments formed during aging. However, it allows differentiation over a broad range of particle sizes for the sediments. Reflectance measurements can be affected by the color of the filterable insoluble, and they may not be successfully correlated with the mass of the material that is filtered. Thus, the accuracy of this method is not 100%. This method can provide an estimate of the stability of fuel when exposed to high temperatures in certain situations, including a recirculating engine or burner fuel delivery system, and under other high temperature conditions with limited exposure to air. In addition, the test method is also helpful in the study of operational problems related to fuel thermal stability. This method is not suitable for fuels with a flash point less than 38 °C. This test method is also not suitable for fuels containing residual oil, so it is only suitable in estimating the high temperature stability of biodiesel with a very high FAME content. This method can be useful for the observation of operational problems linked to fuel thermal stability. Reflectance decreases with increasing amounts of elements. Thus, the increased quantities of biodiesel polymers result in little or no variation in reflectance because the shapes of particles and polymers have almost no observable color with biodiesel. The test provides correct results when the biodiesel particles/polymers are measured gravimetrically.70,73
(b) Rancimat method. The Rancimat method is one of the most effective methods in measuring the thermal stability of biodiesel. In this method, the sample is heated at 200 °C; after 6 h, an 8 g sample is obtained to measure the polymer elements without an airflow.62,71 ASTM D 6468 is one of the most familiar tests for thermal stability, in which the sample is heated at 150 °C for either 90 or 180 min. The sample is then cooled and clarified via a mode similar to ASTM D 227490 to determine the filterable via a total reflectance meter or gravimetrically. This method is easy to handle and suitable for use in terms of repeatability.62 This process also requires less time. Given that it is modified from D6468-08, it results in comparatively less errors and results in better repeatability. Polymers are used as stability parameters, but no national or international standards are available.62,71 Chemical structures determine the thermal stability of oils. Saturated oils are more stable than unsaturated fatty acids at high proportion.72 Recently, the chemical reaction and thermal stability have been successfully utilized to study the physical properties of oils.72
(c) Thermo gravity analytical methods (TGA or DTA). TGA or DTA refers to a set of methods in which the thermal activities or thermal properties of a material are measured as a function of temperature. Temperature plays a significant role in the stability of biodiesel, particularly for oxidation stability. In the TGA measurements, the change in value of any parameter is measured with temperature at different conditions. TGA can be performed either in the presence or absence of oxygen. TGA analysis is used for the determination of some of the properties of triglycerides and their derivatives, such as thermo-oxidative behavior, stability, specific heat, degree of unsaturation from melting, crystallization oil profile curves, and high pressure oxidation – IP measurements. The onset temperatures measured from TGA analysis provide the resistance of the sample to thermal oxidative degradation, and a direct correlation exists between the onset temperature and oxidizability of the sample. The sample becomes easily oxidized with the onset temperature.98–101 The equipment continuously observes a loss in model weight when the sample is heated in isothermal or energetic situations. To identify different properties, such as specific heat, thermal degeneration stimulation energy, thermo-oxidative activities, and stability, thermal analysis systems have been used for the classification of edible oils and fats,102–106 temperature and enthalpy of illustration,107–109 effects of antioxidants on thermal stability of oils106,110 analysis of period of unsaturation from melting and end-product of oil111 and high-pressure oxidation phase measurements.112 Based on the high precision and sensitivity of the method, TGA/DTA is widely employed for determining the thermal stability and thermo-oxidation behavior of oil and biodiesel. Thermal stability is directly correlated with the chemical structure of the sample, and the samples with highly unsaturated fatty acids are less stable than the saturated molecules.
2.2.2. Thermal degradation reaction of biodiesel. Oxidation and thermal instability can result in the degradation of biodiesel fuel properties and harm engine performance. Instability is a fundamental consequence of fatty acid chain unsaturation (carbon double bonds C
C). Many polyunsaturated fatty acid chains in vegetable oil are methylene interrupted rather than conjugated. The twofold obligation of unsaturated fats limits the revolution of the hydrogen molecules joined to them. In this way, an unsaturated fatty acid with a twofold bond can exist in two forms, namely, the cis structure, in which the two hydrogen atoms are on the same “side,” and the trans structure, in which the hydrogen atoms are on the inverse sides. Trans unsaturated fatty acid, or trans fats, are robust fats produced unintentionally by warming fluid vegetable oils in the presence of metal catalysts and hydrogen. This methodology is termed halfway hydrogenation because carbon particles bond in a straight aspect and stay in a strong state at room temperature.113 Physical properties that are sensitive to the effects of fatty oil oxidation include viscosity, refractive index, and dielectric constant. Fig. 5 indicates the mechanism of peroxy radical formation on a methylene group. In oxidative instability, the methylene group (–CH2–) carbons between the olefinic carbons are initially attacked.114 The formation of hydroperoxide follows a noted peroxidation chain system. Oxidative lipid adjustments occur through lipid peroxidation systems, in which free radicals and responsive oxygen species produce a methylene hydrogen atom from polyunsaturated unsaturated fatty acids, creating a carbon-focused lipid radical.
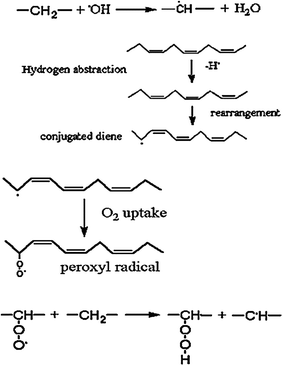 |
| Fig. 5 Mechanism of peroxy radical formation on methylene group.9 | |
Viscosity is one of the crucial properties of biodiesel. The effects of viscosity can be observed in the nature of atomization, ignition, and engine wear. The nature of fuel atomization is fundamentally influenced by viscosity.115,116 During thermal degradation, the viscosity of biodiesel increases because of the trans-isomer arrangement on twofold bonds. Decomposition of biodiesel and its related fatty acids smoothly increase from 293 K to 625 K. The densities of biodiesel fuels diminish linearly from 293 K to 575 K.115 Heat from biodiesel combustion increases when the degree of thermal degradation increases.116 Thermal polymerization of fatty acids is not crucial up to 525 K. Two fatty acid chains are connected by a cyclohexene ring, and thermal polymerization occurs via the Diels Alder reaction. Thus, thermal polymerization of biodiesel can increase its viscosity.
2.3. Storage stability
Storage stability can be defined as the ability of liquid fuel to resist shifts in its physical and chemical properties because of its interactions with its environment.117 Given that oxidation reactions give rise to substances, the stability of biodiesel in storage is critical because it can degrade different parts and materials used in biofuel storage systems. Several writers have observed the activities of biodiesel stored for long time periods in contact with various materials, when exposed and not exposed to light and air. Generally, changes have been reported regarding the IVs, PVs, and AVs, as well as the viscosity, methyl ester content, oxidative stability, and content of insoluble material. The main features for presenting biodiesel and its blends into the market and storage stabilities are characteristic assurance, customer receiving, and standardization. Common diesel fuels are less stable than biodiesel during storage. Feasibility, sustainability, and acceptance are vital issues during storage to protect biodiesel from oxidative degradation. The fuel quality and engine performance deteriorate during long-term storage, interaction with atmospheric air, and other pro-oxidizing situations because of long-term storage oxidation of unsaturated esters in biodiesel.89 Upon interacting with variations in color, light, factors caused by residue formation, waste product, and other changes, storage stability may be affected, thereby decreasing the transparency of the fuel.12 Different writers have studied the effect of biodiesel on the physical properties of the fuel with respect to time, as well as the effects of long-term storage on biodiesel quality.117–123 When stored for two years, the heat from combustion decreases but the PV, viscosity, density, and AV of biodiesel increases.25,117,119,120,124 After a year, the viscosity and AV dramatically transforms with changes in the Rancimat IP on the feedstock. Significant growths in the viscosity, PV, free fatty acid, anisidine value, and UV absorption were observed during 90 days storage tests.27,30,89,125 Moreover, the oxidizability of fatty oils increases with the presence of certain metals, such as Fe, Sn, Ni, Cu, and brass.126 Knothe and Dunn98 identified that rapeseed oil (even in 70 ppm) greatly increases the oxidizability of the fuel containing Cu. However, Fe or Ni does not greatly reduce the oxidation stability index (OSI) of methyl oleate compared with Cu. Bondioli et al.38 found that Fe at 40 °C than Fe at 20 °C is a very effective hydroperoxide decomposer, and its effect on methyl esters in rapeseed oil is highly pronounced. Bessee et al.127 specified that copper does not increase the total acid number (TAN) of soya methyl ester as much as Fe.
2.3.1. Test methods to characterize storage stability.
(a) ASTM D 4625. Standard test method ASTM D 4625 is the most broadly accepted test method for determining the storage stability of central distillate petroleum fuels. The fuel is stored at 43 °C for periods up to 24 weeks. One week of storage in this test is generally accepted as equivalent to one month of storage at 17 °C (65 °F). Normally, a sample is filtered weekly to determine the total insolubles. Whatman GF/F filters (47 mm diameter) are used to test biodiesel. After filtering the aged sample, the filtrate is analyzed for the TAN (ASTM D 664) and kinematic viscosity at 40 °C (ASTM D 445).2,89To estimate the long-term storage stability of central distillate petroleum fuels, ASTM D 4625 is a very effective method. One week of storage at 43 °C is widely accepted as equivalent to four weeks at 15 °C (underground, ambient storage). When the same relationship has to be confirmed for B100, most investigators are inclined to accept that the relationship holds. Thus, D4625 is an excellent inquiry method, but it is not acceptable as a specification test.
(b) Modifying Rancimat test for storage stability. The Rancimat test may also be used for testing the storage stability of biodiesel. To assess storage stability, the test is amended by the BIOSTAB project.128,129 In this test, samples of 3 g (neat biodiesel) and 7.5 g (biodiesel blends) are held at 110 °C with a constant air flow of 10 L h−1 passing through the fuel and into a vessel containing distilled water. The formation of volatile organic acids (mainly formic and acetic acids) in the sample is indicated by an increase in conductivity in the measuring vessel. The time that elapses until the secondary oxidation products are detected is known as the IP. In the modified Rancimat method, several parameters change mainly because of the higher volatility of diesel fuels compared with that of methyl esters, which leads to higher sample evaporation. The modified Rancimat test is suitable for use in terms of repeatability, significance, and feasibility.Table 2 shows that the increase in storage time will increase the PV, AV, density, and viscosity, whereas IP decreases in most cases. Therefore, for long-term storage, newly generated biodiesel components upon degradation should be acidic in nature. Such components will always have higher density and viscosity. The optimal storage time of different biodiesels generally varies from one month to 12 months. Further studies should be conducted to clarify the proper storage time that can stabilize various biodiesel properties.
Table 2 Effect of storage time on of different biodiesel fuel properties (↑ (increase), ↓ (decrease))a
Name of biodiesel |
Time of storage, month |
Measured parameters (PV, IV, AV, V, D, IP, purity, FAME) |
Storage limit (month) |
Method |
Remarks |
Ref. |
JCB: Jatropha curcas biodiesel, RME: rapseed oil methyl ester, SBME: soybean oil methyl ester, HOSME: high oleic sunflower methyl ester, MOME: mahua oil methyl ester, SOEE: sunflower oil methyl ester, SOME: sunflower oil methyl ester, JOME: Jatropha oil methyl ester, POME: palm oil methyl ester, WCME: winter variety C. sativa oil methyl ester, SCME: spring variety C. sativa oil methyl ester. |
RME |
14 |
PV↑, AV↑, V↑, D↑ |
11 |
EN 14112 |
RME biofuel in the course of storage less worse than the properties of fuel containing C. sativa oil methyl |
130 |
SCME |
8 |
PV↑, AV↑, V↑, D↑ |
11 |
EN 14112 |
102 |
WCME |
19 |
PV↑, AV↑, V↑, D↑ |
11 |
EN 14112 |
102 |
Karanja oil |
6 |
IP↓ other constant |
4 |
— |
Significant effect in IP |
131 |
Rice bran oil |
24 |
PV↑, IV↓, AV↑, V↑ |
1 |
Rancimat |
When the test temperature increases a drastic decrease in the induction period |
132 |
POME |
3 |
AV↑, IP↓, V↑ |
1 |
DSC |
Thermal & oxidation stability higher |
133 |
JOME |
3 |
AV↑, IP↓, V↑ |
1 |
DSC |
Coconut biodiesel |
3 |
AV↑, IP↓, V↑ |
1 |
|
SOME |
12 |
IP↓ other constant |
6 |
AACC 58-15 |
Both V and AV shown the greatest potential in terms of timely and relative ease of measurement |
134 |
SOEE |
1.3 month (40 days) |
IP↓ other constant |
— |
EN 14112 |
Low oxidation stability have shown a contamination by some metal ions |
135 |
MOME |
12 |
PV↑, V↑, other constant |
— |
— |
PV &V has significant effect |
136 |
Canola oil |
6.4 |
FAME concentration↓ other remain same |
0.5 |
— |
|
137 |
Hi-olec sunflower oil |
30 |
PV↑, AV↑, IV↓,V↑ |
12 |
— |
Friction and wear increase with increasing temperature |
138 |
High erucic brassica oil |
Low erucic brassica oil |
Linseed oil |
2 |
IP↓ |
1.4 |
PDSC |
The oxidation stability of biodiesel are deeply impacted by several extrinsic factors and others residual contaminants from the synthesis and storage process |
139 |
Neat edible rapeseed oil |
12 |
Purity of biodiesel↓ |
5 |
TGA |
The lowest thermal stability in synthetic air |
140 |
Tallow oil |
2 |
IP↓ |
1 |
Supercritical |
After the exposure for biodiesel initially oxidation stability became better high in peroxide value |
141 |
Lard |
2 |
IP↓ |
1 |
SBME |
6 |
IP↓ |
1 |
EN 14112 |
Oxidation stability increase by using antioxidants additives |
10 |
HOSME |
6 |
RME |
6 |
JCB |
6 |
IP↑ |
— |
EN 14112 |
To maintain the IP of 6 h for predefined period of time conforming to biodiesel standard specifications concentration of antioxidants required to be added to biodiesel |
142 |
Soybean |
6 |
IP↓ |
6 |
Rancimat |
|
143 |
3. Review of different stabilities of biodiesel
3.1. Oxidation stability studies of biodiesel
Sarin et al.25 observed the oxidation stability of biodiesel in the presence of metal contamination in Jatropha biodiesel, and described a similar influence on the oxidation stability in the presence of small or large metal contamination concentrations, but copper showed the strongest detrimental and catalytic effect on the oxidation stability.
Xin et al.59 reported the oxidation stability of rapeseed biodiesel using the supercritical methanol method. Their group utilized rapeseed biodiesel as a representative biodiesel to observe the effect of temperature on the tocopherol content in biodiesel. At 270 °C/17 MPa, 300 °C/20 MPa, 330 °C/37 MPa, and 360 °C/47 MPa for 30 min, rapeseed biodiesel was exposed to supercritical methanol, and the remaining tocopherol content of rapeseed biodiesel was measured. Their results demonstrated that the remaining tocopherol decreased with the increase in temperature above 300 °C. The increase in temperature significantly reduced the tocopherol content. They concluded that tocopherol is not stable at temperatures above 300 °C. Biodiesel has lower stability in supercritical method compared with other methods, such as transesterification.12,26,117,144
Knothe and Dunn126 investigated the effect of Cu, Fe, and Ni on the biodiesel stability via IP measurement of methyl oleate at 90 °C, in which copper resulted in the smallest IP compared with the other metal-contaminated methyl oleate samples.114 Many researchers have studied the effect of metal contamination on the stability of biodiesel at different conditions and various biodiesels. Jain and Sharma145 recently investigated the metal contamination effect of Jatropha biodiesel with and without antioxidant. Their group used different metals, such as Fe, Ni, Mn, Co, and Cu, in varying concentrations. These different metal concentrations were mixed with biodiesel, and their storage stability was analyzed. The concentration of antioxidants that effectively increases the stability of samples containing different metal contaminants differs. In this study, pyrogallol (PY) was used because it is one of the best antioxidants for stabilizing biodiesel without metal contaminants.
3.2. Thermal stability studies of biodiesel
Many studies have investigated the thermal stability of biodiesel, as well as the effects of temperature on the stability of biodiesel. Dunn4 studied the effects of biodiesel temperature on the OSI, and described that the oxidation reaction occurs rapidly by increasing the temperature and decreasing the OSI of FAME. Monyem et al.14 inspected the thermal stability of biodiesel under different conditions, and reported an increase in the viscosity of the fuel because the oxidation process accelerated with increasing temperature. Polavka et al.146 studied the oxidation IP, which is dependent on temperature. Using the Arrhenius equation, they found that the IP of oxidation varies with temperature. Conceicüão et al.65 evaluated the thermal stability, and found that only methyl linolenate undergoes a slight change in cis–trans isomerization at 270 °C/17 MPa without affecting the biodiesel yield, and poly-unsaturated fatty acid methyl esters, such as methyl linoleate (18
:
2) and methyl linolenate (18
:
3), are extensively decomposed at 350 °C/43 MPa, accompanied with isomerization of cis-type double bonds into trans-type at 3508 C/43 MPa in supercritical methanol. Dunn147 observed the effects of oxidation under accelerated conditions on the fuel properties of soyate, and found that the increase in various biodiesel properties, such as viscosity, PV, and AV, is caused by the increasing temperature. Hence, very little effect was observed for the cold flow properties at temperatures up to 150 °C on the specific gravity. As the reaction temperature increases, the viscosity also increases linearly. The Diels Alder reaction results in the formation of polymers at high temperatures, so the viscosity increases. Meanwhile, AV decreases linearly with increasing reaction temperature, and thermal degradation is responsible for the increase in AV with increasing temperature. The PV decreases linearly, whereas the reaction temperature increases, possibly because of the absence of oxygen or accelerated decomposition of hydroperoxides with increasing temperature.
Dunn4 studied the temperature effect on the stability of biodiesel, and described that temperature significantly affects the OSI. By increasing the temperature, the oxidation reaction occurs rapidly and OSI decreases. Dunn reported that the polymer formation rate increases, and the viscosity and AV increase, whereas the PV decreases at higher temperatures, resulting in decreased OSI.121 Nzikou et al.133 evaluated the thermal stability of vegetable oils while frying, and found that the content of linoleic acid decreased with the increase in time of frying oil, which occurred because of rapid oxidation. The formation of high molecular weight polymers causes the viscosity of oil to increase with the increase in frying hour. A high viscosity in frying oil results in a high degree of deterioration105 this result is in agreement with the findings of Dunn.134
Xin et al.28 observed the temperature effect on safflower oil during IP, and found that the IP decreases rising temperature. Bondioli et al.40 analyzed the storage stability of biodiesel at different temperatures. During their experiment, samples were kept at two different temperatures (20 °C and 40 °C), and their results demonstrated that the PV was higher at lower temperatures at the same container.
Therefore, based on these lines of evidence, supercritical methanol treatment lower than 3008 °C, preferably 2708 °C with a pressure higher than 8.09 MPa, was concluded to be appropriate in maintaining the maximal yield and thermal stabilization of biodiesel.
3.3. Storage stability studies of biodiesel
Bondioli et al.40 and Thompson et al.125 have investigated the corrosion of methyl esters in rapeseed oil, and found that AV, PV, and viscosity increase with time under various storage conditions.89 Different authors98 have studied natural antioxidants, such as tocopherol, and a relationship between the oxidation stability and quantity of tocopherol was found. Bouaid et al.117 studied the long-term storage stability of biodiesel from high oleic sunflower oil using frying oil, and found that the IV decreases with rising storage time, whereas the AV, PV, and viscosity increase. Meanwhile, McCormick et al.47 found that polyunsaturated contents have the longest effects on biodiesel stability because of the increased insoluble formation and reduction in the generation period.
Das et al.27 observed the oxidative stability of karanja oil ME (KOME) via storing the sample inside a room at open air and exposing it to metal and air. They found that the viscosity and PV decrease with increasing storage time, thereby decreasing the oxidative stability of KOME and increasing oxidative degradation, despite the high PV and viscosity. Antioxidants significantly affect KOME. By increasing the concentration of the antioxidant [propyl gallate (PG), butylated hydroxyanisole (BHA), and butylated hydroxytoluene (BHT)] with changing load, the stability of KOME increases, and PG is the best antioxidant for KOME, followed by BHA and BHT. Geller et al.120 investigated the storage stability of poultry fat and diesel fuel, which are liable for the corrosive effect of fuels on various metals, with respect to the separation, dynamic viscosity, sedimentation accumulation, and specific gravity. At a storage time of over one year, the viscosity and specific gravity slightly change, and the addition of 100% antioxidant minimizes the physical properties and sedimentation.
For perfect mixing followed by homogenization, mixing should be very fast and within the suggested time before the fuels are utilized. Regarding the corrosive properties, brass and copper were found to be susceptible to attack by biofuels, whereas 316 stainless steel and carbon steel were not attacked by biofuels. Sarin et al.119 reported that the concentration of metal contamination (more or less) in Jatropha biodiesel has the same influence on oxidation stability; the oxidation stability of copper shows the strongest detrimental and catalytic effects.
4. Techniques to improve the stability of biodiesel
4.1. Purifying during production
To improve the biodiesel stability, some processes are necessary to meet the biodiesel's specification for stability under various conditions. Biodiesel consists of fatty acid monoalkyl ester, which is normally produced by transesterification reaction.148 Technology development should be geared toward better process innovations and the processing of biodiesel.149 Raw materials and other elements first have to be managed to confirm the quality of biodiesel. Some crude vegetable oils contain phospholipids, which are harmful to biodiesel, thus, such oils have to be eliminated via hydration processes.75 Deodorization is the most effective refining process in removing unwanted odor and test forms in oil. Therefore, free fatty acids, ketones, aldehydes, and unsaturated hydrocarbons, all of which can cause undesirable smells and flavors of the oil, can be removed via deodorization.150 Iodine as a catalyst can reduce the high AV of free fatty acids. Transesterification is catalyzed by either homogeneous reagent or heterogeneous reagent. Homogeneous reagents include potassium hydroxide, hydrochloric acid, sodium hydroxide, and sulfuric acid, whereas heterogeneous reagents are enzymes, heterogenized on organic polymers, alkaline earth metal compounds, anion exchange resins titanium silicates, and guanidine. During storage with air, the alkali homogeneous catalysts are extremely hygroscopic.151 Thus, the alkali homogeneous catalysts should be appropriately handled. The alcohol materials, such as methanol, amyl alcohol, ethanol, propanol, and butanol, are used in the transesterification process. At the end of transesterification, alcohol and glycerol are eliminated from the selected product esters by water washing.124,152 The use of membrane technology for the separation and refining of biodiesel eliminates water washing and results in a realistic amount of time and energy depletion.153 Cooke et al.154 observed that ion interchange resin can eliminate impurities. Gabelman and Hwang155 found that hollow fiber membrane abstraction can be used effectively to eliminate contaminants. These methodologies effectively avoid losses in the biodiesel yield, decrease the manufacturing steps, and increase the properties of fuel.
4.2. Adding of different additives
Several researchers14,156–158 have studied biodiesel stability in the presence of additives. Two types of antioxidants are generally used: chain breakers and hydroperoxide decomposers. Phenol and amine are the two most familiar types of chain-breaking antioxidants.132 Generally, antioxidants are highly effective for maintaining biodiesel stability under different conditions. Antioxidants can prevent the oxidation process, and they are well recognized for maintaining the oxidation of biodiesel. For instance, antioxidant (AH) intercepts the peroxide radical (RCOO˙) to prevent it from generating another radical by the autoxidation appliance. The associated mechanism is as follows:2
Radical tapping stage: R–COO˙ + AH = R–COOH + A˙ |
Radical termination stage: A˙ + A˙ = A–A or non-radical materials |
Most studies on the stability of fatty oil and ester uses are restricted to the phenolic type of antioxidants. The necessity of phenolic antioxidants depends on the number of hydroxyl/phenolic groups involved to its ortho and para positions, i.e., 1 and 2 or 1 and 4 positions in an aromatic ring. For the suspension of the oxidation rate, protons, which are delivered by an active hydroxyl group, can prevent the formation of free radicals or interject the dissemination of free radicals. Antioxidants are described by their molecular structure. Therefore, the effectiveness of tert-butylhydroquinone (TBHQ), PG, and PY can be described based on their molecular structure. These additives possess two OH sets attached to the aromatic ring, when both BHT and BHA possess one OH group on the aromatic ring. Thus, based on their electro negativities, TBHQ, PG, and PY offer more sites to form a complex between the free radical and antioxidant radical for the stabilization of the ester chain.159
Antioxidants are reused for delaying oxidation. From the literature reviews, antioxidants effectively improve the stability of biodiesel under various conditions. Different additives are used in various conditions for the improvement of biodiesel stability.4 Many antioxidants can markedly increase biodiesel stability. Among them, TBHQ is the most effective antioxidant for soybean, Jatropha, palm, and sunflower biodiesel. BHT, PG, and BHA are also found to be effective for most biodiesels.160 The application of several antioxidants can enhance the fuel stability up to the standard specification. The additive concentration may vary at different conditions because of the unsaturation concentration and composition of various biodiesel and biodiesel blends.161 However, many possible scopes of study can be explored to understand the effectiveness of different additives in enhancing corrosion resistance and stability, as well as the fuel properties of different biodiesels.
4.2.1. Chemistry of antioxidant. Antioxidants play a significant role in biodiesel degradation. As indicated by their mode of activity, antioxidants can be classified as follows: primary antioxidants, such as free radical terminators; oxygen scavengers that react with oxygen in closed frameworks; or metal particle chelators that catalyze lipid oxidation.162 To change them to more steady primary antioxidants, they should react with highly vital lipid radicals. Hence, the group of free radical eliminators, such as the phenolic antioxidant (AH), is the most utilized antioxidant. Secondary antioxidants function by retarding the rate of chain initiation by decomposing hydroperoxides. TenáHave et al.163 first investigated the activity of antioxidants, in which they hypothesized reactions (5) and (6) with the expectation of complimentary radical terminators. In oxidation process reactions (5) and (6), the free radical terminators have the most capable hydrogen atoms, which are very fast compared with peroxyl.164 The latter reactions, (7) and (8), compete with the chain propagation reaction. Naturally, these reactions are exothermic. With the increase in separation vitality of A–H and R–H, the initiation vitality of these reactions increases. As the bond quality of A–H diminishes, the productivity of antioxidants increases.165 |
ROO˙ + AH → ROOH + A˙
| (5) |
Reaction (9) shows the valence bond of isomers, which is the stabilization of phenoxy radical and occurs because of the delocalization of unpaired electrons around the aromatic ring. Hydrogen substitution by alkyl assembles in ortho- and para-position, and expands the electron density of the OH moiety by inductive impact.165
|
 | (9) |
The antioxidant activity is increased by an ethyl or n-butyl group, rather than a methyl group at the para-position.166 The stability of the phenoxy radical is increased by the bulky groups at the ortho-position as in BHA.167 These substituents increase the stearic hindrance in the region of the radicals, as well as reduce the rate of possible propagation reactions, which are shown in reactions (10), (11), and (12).
|
AOO˙ + RH → AOOH + R˙
| (11) |
BHT, as a white crystalline compound, is momentarily accessible as white waxy drops.162 BHT and BHA are strongly soluble in fats and insoluble in water. As mono-phenols, they both produce radical intermediates with moderate reverberation delocalization. The tert-butyl gatherings of BHT do not permit radical shapes for it to be involved in different reactions.168 A lipid peroxyl radical may join the molecule of BHT, which is shown in reaction (13).165
|
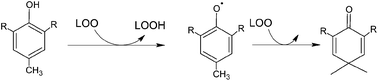 | (13) |
TBHQ is economically accessible as a beige-hued powder, and it is a sufficient solvent in fats. As a diphenolic antioxidant, TBHQ reacts with peroxyl radicals to structure a semiquinone reverberation half and half. The semiquinone-organized radical intermediates may experience distinct responses to structure more steady items. They can also react with each other to deliver dimers, dismutate, and recover semiquinone. They can react with an alternate peroxyl radical, as indicated in reactions (14), (15), and (16).165
|
 | (14) |
|
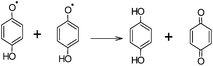 | (15) |
|
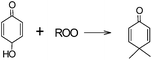 | (16) |
Based on their labile hydrogen, the phenolic antioxidant can be positioned as BHA ≈ BHT < DTBHQ ≈ TBHQ < PG ≈ PY, which is relevant to some edible oil-based biodiesels. An alternate crucial antioxidant is amine, which can be categorized as a free radical terminator.
4.2.2. Antioxidants effect on stability of biodiesel. Several methods are available to prevent oxidation or decelerate its rate. By avoiding contact with oxidizing conditions, as well as decreasing air contact, storage in a dark room in stable containers and low temperatures at inert conditions are highly effective for the prevention of biodiesel oxidation.169 However, the above conditions are difficult to achieve, and the use of chemicals is of considerable concern. The use of antioxidants is the most active and commercial method available to effectively increase the oxidative stability of biodiesel and its blends. Through the extension of the IP, antioxidants have an enhanced role in delaying oxidation. The most common antioxidants are chain breakers (peroxide radical quenchers) and hydroperoxide decomposers (reducing agents).135 Hydroperoxides react with the antioxidants of hydroperoxide decomposers and then convert them to alcohols. Hence, harmless oxidation forms from the antioxidant. Metal chelating agents and acid neutralizers are other categories of antioxidants.170 The synthetic antioxidants provide higher effectiveness compared with tocopherols when they are added to biodiesel. Another effective natural antioxidant is cardanol oil, which is extracted from cashew nut shell liquid171 citric acid and caffeic acid are other naturally derived antioxidants that are used as biodiesel stabilizers.172 Photo-oxidation is influenced by quenchers of singlet oxygen, such as β-carotene. The major synthetic antioxidants are BHT, BHA, TBHQ, PY (i.e., 1,2,3-trihydroxybenzene), and PG (i.e., propyl 3,4,5-trihydroxybenzoate).166 Baynoxs is a commercially available synthetic antioxidant widely used for the storage of biodiesel173 BHA, BHT, PG, and vitamin E are chain breakers, whereas sulfides and phosphides are peroxide destroyers (Fig. 6).174
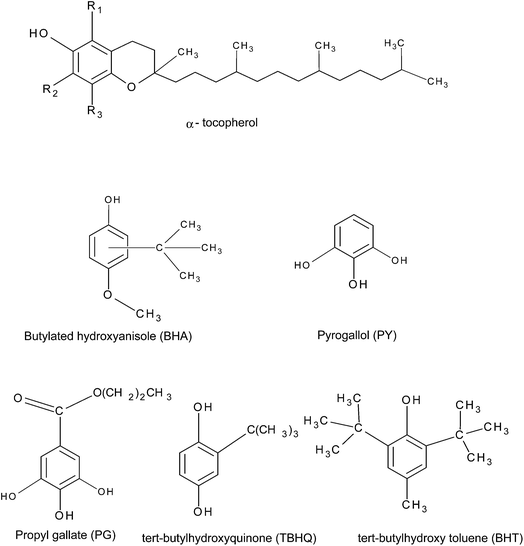 |
| Fig. 6 Chemical structures of different antioxidants.175 | |
Antioxidant occurs naturally, such as vitamin E (i.e., tocopherols and tocotrienols). The four species in each α, β, γ, and δ exist or the synthetic antioxidant materials are deliberately added, such as PG, BHT, TBHQ, PY, and BHA. The refining process influences the level of natural antioxidants in vegetable oil. Early reports stated that the most effective synthetic antioxidants are pyrimidinols, which have strong electron-donating dialkylamino groups.176 To interrupt the chain reaction, the phenol and amine antioxidants donate the hydrogen atom; phenols go to quinones and react with a radical in an additive fashion. For proper solubility and utility, the levels of synthetic antioxidant that are normally used are in the range of 100–200 ppm, and the antioxidant with the lowest solubility is PG. Different factors, such as storage, amount of naturally occurring antioxidant, oil fatty acid profile, and other conditions, influence the utility of antioxidants. Primary and secondary antioxidants are used in the system; they can influence their effectiveness via synergistic effects between the antioxidant. Meanwhile, amino acid, citric acid, ethylenediaminetetraacetic acid, and phosphoric acid function as secondary antioxidants and help in removing metal ions with different materials, including chelating agents. Oxygen scavengers or reducing agents, such as ascorbic acid, which can regenerate spent antioxidants, and singlet oxygen quenchers, such as β-carotene, are also used as oxidation inhibitors. The activity of each antioxidant on biodiesel is different with various feedstocks, FAME composition, and oxidative stability.177,178 Biodiesel stability increases with the addition of antioxidants and varies with the antioxidant concentration. Metal contamination also influences stability. Hence, various metal containers are used for biodiesel storage. To increase the oxidation stability of biodiesel, various techniques have been used, such as fractional crystallization or hydrogenation, for reducing the unsaturated fatty acid content in oil. With the variations in the molecular weight structure, BHA and BHT are less effective compared with PY, TBHQ, and GA. The effects of the antioxidants on the oxidative stability of biodiesel mainly depend on the group; maximum antioxidant has two hydroxyl groups, but BHT and BHA have only one group with an aromatic ring. Thus, BHT and BHT are less effective on biodiesel stability.179 Meanwhile, TBHQ and PY are strongly effective on the biodiesel stability, and they are more active in the development of a complex between the free radical of the sample antioxidant.180,181 Methyl ester stabilization in biodiesel is responsible for its complexity. Antioxidants also influence the viscosity of biodiesel. During storage of oxidized compounds, viscosity increases because of the addition of antioxidants in biodiesel; thus, for long-term storage, viscosity in biodiesel affects stability. However, the addition of proper concentrations of antioxidants, such as PG, BHT, and BHA (1000 ppm), causes the viscosity of biodiesel to remain under the ASTM limit (1.9–6 cSt).182 Table 3 lists the selected studies on the evaluation of biodiesel oxidation stability upon antioxidant addition. It also shows that the most effective synthetic antioxidant is TBHQ, followed by PY and PG. In the literature, BHA and BHT are found to be less effective. However, based on the table, synthetic antioxidants are more significantly effective than natural antioxidants, such as different tocopherols and vitamin E. Much work has been conducted on the mechanism of oil and fat degradation based on the above literature review.
Table 3 Impact of the use of antioxidant for the oxidation stability of biodiesel
Biodiesel |
Antioxidant order |
Stability effect |
Concentration (ppm) |
Remarks |
Ref. |
Karanja oil |
PY > PG > BHA > BHT > TBHQ |
Oxidation stability ↑with ↑of antioxidant |
300, 500, 700, 1000 |
PY retain for all concentration |
145 |
Neat Jatropha |
TBHQ > BHT > TBP > OBPA > α-T |
Oxidative stability ↑with ↑of antioxidant |
1000 |
600 ppm Of natural AO α-T is needed for improve IP |
116 |
Jatropha curcas |
PY > PG > TBHQ > BHT > BHA |
Thermal stability ↑with ↑of antioxidant |
1000 |
To meet EN 14112 specification 100 ppm of PY is required minimum concentration |
73 |
Jatropha oil |
PG > TBHQ > BHA |
PG is suitable for long term oxidation |
|
For 150 ppm PG decrease the IP |
183 |
(Rapseed oil, sunflower oil, frying oil, beef tallow oil) (undistilled & distilled) |
TBHQ > PG > PY > BHA > BHT, PY > PG > BHA > TBHQ > BHT, PY > PG > TBHQ > BHA > BHT, PY > PG > TBHQ > BHA > BHT, PY > PG > TBHQ > BHA > BHT, TBHQ > PG > BHA > PY > BHT, PY > TBHQ > PG > BHA > BHT, PG > PY > TBHQ > BHT > BHA |
BHT has no effect except tallow ME (distilled) |
1000 |
Antioxidant concentration were varied from 100 to 1000 mg kg−1 |
184 |
Rapseed oil & tallow |
BHA ∼ BHT & BHT > BHA |
IP↑ with ↑of antioxidant concentration |
400 |
Rate of deactivation increase with unsaturation in the oil |
185 |
Mahua oil |
PG > BHA > BHT |
The use of antioxidants improved the stability significantly |
1000 |
PV & viscosity increase over the time |
186 |
Soyaben oil, soyaben oil |
BHT > BHA > TBHQ, TBHQ > BHT > BHA |
TBHQ displayed a greater stabilizing potential when used in higher concentrations. |
1000, 7000 |
From 200 to 7000 ppm BHT displayed the greatest efficacy in concentrations ranging |
187 |
Distilled & crude palm oil |
TBH > BHT > α-tocopherol. |
BHT and TBHQ are found to be more effective than natural antioxidant |
— |
At the same dosage TBHQ is more effective compared to BHT |
188 |
Waste cooking oil |
BPH > BHT |
Oxidation stability ↑ with ↑of antioxidant concentration |
600 |
BPH was more effective than BHT and could be used as a antioxidant |
189 |
Terminalia oil |
PG > PY > TBHQ > BHT > BHA > α-tocopherols |
Storage stability depends mainly on the antioxidant type and concentration |
1500 |
Stability can be upgraded up to 12 times by 1000 ppm PG |
190 |
Croton oil |
PY > PG > BHT |
↑ in dosage of antioxidants ↑oxidation stability |
1000 |
To improve the oxidation stability of COME synthetic antioxidants was required. |
191 |
Cotton seed oil |
TBHQ |
Oxidative stability increases linearly with the amount of antioxidant |
1000 |
The addition of 300 mg kg−1 TBHQ was sufficient to attend the oxidation stability parameter |
192 |
4.3. Modification of storage conditions
The stability of biodiesel can be increased by modifying storage conditions. Biodiesel can be stored in different ways, and different factors influence biodiesel stability during storage, such as oxidative stability, solvency of fuel, and compatibility of materials.156,157,193,194 Lee et al.158,195 studied the storage condition of biodiesel in different ways, and concluded that the storage temperature should be between 7 °C and 10 °C to protect pure biodiesel from oxidation. By contrast, the extreme cold weather underground storage of pure biodiesel needs an optimized storage temperature to avoid crystal formation.159,196 Biodiesel should be stored appropriately because the environment affects the different elements that can degrade biodiesel and reduce its stability. For more than six months of storage, pure biodiesel and its blends require appropriate antioxidants because antioxidants have different characteristics that can increase biodiesel stability. Contamination from water may lead to biological progress in the fuel, so biocides that can prevent water contamination in the fuel should be used. Aluminum, steel, polypropylene or fluorinated polyethylene, and Teflon are the main containers for biodiesel storage.197 Aluminum is the most suitable container for biodiesel storage because it has no catalytic effect on biodiesel. In summary, biodiesel degradation can be affected by temperature and air coverage, which are major important features affecting degradation. The degradation rate increases significantly when biodiesel is stored in environments with high temperature and ambient air exposure. However, the temperature or air exposure alone has minimal effects on biodiesel degradation. The water content in biodiesel will increase biodiesel degradation because of hydrolysis, but its consequence is much less than that of the other factors. The aforementioned factors should be studied in detail, particularly for long-term biodiesel storage.157 The tanks should minimize the presence of water impurities, and should be thoroughly cleaned prior to use for biodiesel storage.23
5. Effect of stabilized blends on engine performance & emission
Numerous studies on the performance and emission analyses of biodiesel or mixed biodiesel-fueled diesel engine are accessible.160–163,198–201 However, reports on the management of the performance and emission of antioxidant prevention agent in biodiesel-fueled engines are limited. Antioxidants demonstrate slight (either expand or abatement) effects on the engine performance and fuel properties. Biodiesel is attracting interest as an eco-accommodating fuel, so elements influencing its ignition and emission are essential. Investigations on the part of antioxidants in compound contamination of biodiesel-fueled engines demonstrated that the determination of the antioxidant additive substance to biodiesel is imperative in evaluating pollutants. In the report of Suyin Gan and Hoon Kiat Ng, the effect of antioxidant addition to biodiesel on engine emission was examined in the mixtures of palm oil biodiesel with diesel (B10 and B20).164,202 Among the examined antioxidants (i.e., BHT, BHA, and TBHQ), decreased NO emission has been shown by BHA and TBHQ; however, their high concentration in the fuel blends expands the NO levels. Additionally, BHA can even decrease CO emission, but the two other antioxidants are unable to do so. Kivevele et al. observed that the addition of 1000 ppm PY antioxidants to croton oil methyl ester exerts a negligible influence on engine exhaust emissions.165,203 The emission of CO, NOx, total hydrocarbons, and smoke decreases or expands based on the applied load. The effect of antioxidants BHA, BHT, TBHQ, and 2-ethylhexyl nitrate (EHN) on the engine performance and fume gas outflow of canola oil methyl ester mix (B20) was researched by Erol Ileri and Gunnur Koçar.166,204 NOx emission was observed to decrease upon antioxidant addition, whereas the CO level expanded. EHN was the most effective in decreasing NOx, and TBHQ showed minimal evolution of CO. Jain and Sharma167,205 analyzed the emission of Jatropha biodiesel-fueled diesel engine, and found that the additive PY has minimal effects on the emission. The exhaust of CO and THC slightly decreases, whereas NOx formation slightly increases or decreases with engine load.167 Analysis on the emission of soybean biodiesel-fueled engine showed that the antioxidants have no effects on the engine exhaust.168,206 The use of antioxidant additives is one of the most cost-effective ways to lessen the emission of NOx from biodiesel-fueled engines.169,207 However, in their study, the formation of CO and THC increased. The antioxidants selected for their study were EDA, p-PDA, BHT, α-tocopherol, and L-ascorbic acid; p-PDA showed the best activity in reducing NOx emission. In most studies on antioxidant added biodiesel emission analysis, a reduction in NOx and slight increase in CO and THC are observed. The amounts of CO and THC are lower than that emitted by diesel fuel.5,170,208 Compared with the emission from diesel fuel ignition, NOx formation is the main imperfection of biodiesel fuel. Based on the aforementioned studies, antioxidants can assume a real part in decreasing NOx.209,210 The establishment of NOx is caused by the presence of free radicals in fuel, as effectively specified in this article. The fuel radicals react with nitrogen from the air to form NOx. The presence of antioxidants can end the radical reaction and decrease NOx formation.
From Table 4 it can be concluded that addition of antioxidant in biodiesel significantly effect on engine performance and emission hence decrease the NOx and most of the cases CO, HC, increase but engine performance increase and good improve BSFC and BP compare that of without antioxidant. Addition of BHA, BHT, TBHQ increase CO and HC due to the reduction of oxidative free radicals.
Table 4 Comparison performance & emission with and without antioxidanta
Test fuel/blend |
Engine specification |
Operating condition |
Comparison of emission with and without antioxidant |
Comparison of performance with and without antioxidant |
Antioxidant concentration |
Ref. |
NOx |
CO |
HC |
Smoke |
BSFC |
BP |
1C: single cylinder, 4S: four stroke, IP: injected pressure, RP: rated power, CR: compression ratio, TDI: turbocharged direct injection, WC: water cooled, DI: direct injection. |
Soybean (SB100) |
4C, 4S, CR: 22, RP: 80 kW at 4000 rpm, IP = 150 bar |
Constant speed and different load |
Slightly higher than without AO |
Lower than |
Lower than without AO |
Lower than without AO |
↓ |
— |
500–2000 ppm |
172 |
Canola (B100, B50, B20, B10) |
2C, 4S, DI, CR: 16.5 : 1, RP: 11.2 kW at 1800 rpm, IP = 22 bar |
Constant speed & different load |
↓up to 5% but slightly ↑at low load and medium condition |
Max ↓about 19% |
Max ↓upto 65% at B100 |
— |
↑6% with ↑blend ratio and AO |
— |
— |
211 |
Kerosene (B100, B50, B20, B10) |
2C, 4S, DI, CR: 16.5 : 1, RP: 11.2 kW at 1800 rpm, IP = 22 bar |
Constant speed & different load |
↓up to 5% but slightly ↑at low and medium load condition |
Max ↓about 19% |
Max ↓upto 65% at B100 |
— |
↑6% with ↑blend ratio and AO |
— |
— |
173 |
Neem oil (B100) |
1C, 4S, DI, CR: 12 : 1 to 18 : 1, RP: 3.5 kW at 1500 rpm |
Constant speed & different load |
↓up to 19.99% at full load condition |
↑slightly 4.3% |
↑slightly 1.62% |
↑up to 2.02% |
Same |
Same |
100–400 ppm |
212 |
Coconut (B20) & Jatropha (B20) |
4C, DI |
Different speed & constant load |
↓2.6–5.0% |
↑4.9–20.8% |
↑23.2–40.2% |
— |
↓0.55–0.79% |
↑ |
175.8–184.9 ppm |
213 |
Canola (B20) |
4C, 4S, CR: 19.5 : 1, WC, TDI |
Constant load & different speed |
↓4.3% |
↑ |
— |
— |
↓10.19% |
— |
1000 ppm |
214 |
Calophyllum (B20) |
DI, 4C, RP: 55 kW |
Constant load & different speed |
↓1.6–3.6% |
↑39.14% |
↑26.5% |
— |
↓up to 1.5% |
↑ |
1000–2000 ppm |
161 |
Palm (B20) |
DI, 4C, RP: 42 kW |
Constant load & different speed |
↓upto 9.8–12.6% |
↑8.6–12.3% |
↑9.1–12.0% |
— |
↓0.18–0.64% |
Lower up to 0.68–1.02% |
1000 ppm |
160 |
Jatropha (B5, B10, B15, B20) |
4C, 4S, DI, RP: 55 kW |
Full load & different speed |
↓up to 8.03–16.54% for all blends |
↑significantly |
↓↑up to 9.33–44% for all blends |
— |
↑higher at 1000 rpm |
↓ |
— |
170 |
Croton megalocarpus (B100) |
4C, TDI, CR: 195 : 1, RP: 66 kW at 1900 rpm |
Different load & constant speed |
Slightly higher at 1000 ppm |
Slightly higher at 1000 ppm |
Slightly higher at 1000 ppm |
Slightly higher at full load condition |
↓14.24% |
— |
1000 ppm |
215 |
Soybean (B20, B100) |
1C, 4S, DI, RP: 4.4 kW, IP: 200 bar |
Constant speed |
↓up to 4.06–9.35% |
↑over 9.09% for SB20 and 14.28% for SB100 |
↑about 10.52–16.92% |
↑significantly |
— |
↑slightly |
1000 ppm |
171 |
Jatropha (B100) |
1C, 4S, DI, RP: 4.4 kW, IP: 200 bar |
Constant speed & different load |
↓5.86–43.44% for all AO |
↑for all AO |
↑ |
↑ |
Slightly ↑at BHT, α-tocopherol |
↑for all load |
0.025% m |
169 |
6. Conclusion
Different parameters are affected by biodiesel stability, which can be categorized into oxidation, thermal stability, and storage stability. The present review has covered the various types of fuel stabilities, mechanisms of occurrence, effect of different parameters on the stability of biodiesel, test methods, stability measurements, and improvement of biodiesel stability. The following conclusions were drawn:
1. IP, PV, AV, IV, viscosity, and density are the main parameters related to the stability of biodiesel; among these parameters, IP and BAPE are the major factors.
2. Various tests have been applied to determine the oxidation stability; among them, IR analysis is an effective, fast, easy, and non-destructive method.
3. To determine thermal stability, the Rancimat test, D 5304-06, and TGA/DTA are commonly used. Among these various tests, Rancimat is the most effective method to investigate the thermal stability of oils, biodiesel fuels, and fats.
4. More effective and important research and development areas are needed to enhance and improve the stability of biodiesel for future use in existing engines as a substitute to petroleum diesel. Studies on the effect of antioxidants in improving the oxidation stability revealed that the activity of each antioxidant differs depending on the type of biodiesel feedstock used, antioxidant concentration, blending percentage with petrodiesel, and storage conditions.
5. The effectiveness of these antioxidants can be written in the following order: TBHQ > PY > PG. Among the different antioxidants, PG is the best antioxidant for most biodiesel samples; an additional advantage is that, the decreased NOx emission from the biodiesel-fueled diesel engines.
6. Aluminum is the best storage container for biodiesel because it does not exert any catalytic effect on biodiesel oxidation.
7. Synthetic antioxidants are more effective on the engine performance and emission; the addition of antioxidants in biodiesel significantly affects engine performance and emission, thereby decreasing NOx and increasing CO and HC. However, engine performance increases, indicating good improvement. BSFC and BP decrease without antioxidants, and BHA, BHT, and TBHQ increase CO and HC because of the reduction in oxidative free radicals.
8. Additional research is required to investigate the effect of biodiesel stability on engine performance, as well as the effect on emissions. Further studies are required to investigate and confirm that the use of antioxidants will ensure the long-term storage stability of biodiesel fuel. More experimental research is required to clarify the effect of antioxidant on biodiesel emission formation, as well as analyze the effectiveness of other antioxidants under wider operating conditions.
Nomenclature
ASTM | American standard test method |
AV | Acid value |
AOM | Active oxygen method |
APE | Allylic position equivalent |
AH | Antioxidant |
AACC | American association of central chemists |
BHA | Butylated hydroxyanisole |
BHT | Butylated hydroxytoluene |
BAPE | Bis-allylic position equivalent |
BP | Break power |
BSFC | Break fuel specific fuel consumption |
CO | Carbon monoxide |
CA | Citric acid and caffeic acid |
DI | Direct injection engine |
DPF | Diesel particulate filter |
D | Density |
DSC | Differential scanning calorimetry |
EDTA | Ethylenediaminetetraacetic acid |
EHN | Ethylhexyl nitrate |
FAME | Fatty acid methyl ester |
FFA | Free fatty acid |
HC | Hydro carbon |
IV | Iodine value |
IP | Induction period |
NOx | Nitrogen oxides |
OT | Oxidation temperature |
OX | Oxidizability |
OSI | Oil stability index |
PDSC | Pressure differential scanning calorimetry |
PAO | Polyalpholefins |
PY | Pyrogallol |
PG | Propyl gallate |
PV | Peroxide value |
RIP | Rancimat induction period |
TAN | Total acid value |
TGA | Thermogravity analysis |
TBHQ | tert-Butylhydroquinone |
TDI | Turbocharged direct injection |
THC | Total hydro carbon |
V | Viscosity |
Acknowledgements
The authors would like to thank University of Malaya for financial support through High Impact Research grant titled: “Development of alternative and renewable energy carrier (DAREC)” UM.C/HIR/MOHE/ENG/60.
References
- H. Rashedul, H. Masjuki, M. Kalam, A. Ashraful, S. A. Rahman and S. Shahir, Energy Convers. Manage., 2014, 88, 348–364 CrossRef CAS PubMed.
- S. Jain and M. P. Sharma, Renewable Sustainable Energy Rev., 2010, 14, 667–678 CrossRef CAS PubMed.
- I. Hiroaki, M. Eiji, H. Shusaku and S. Saka, Fuel, 2008, 87(1), 1–6 CrossRef PubMed.
- R. O. Dunn, Biofuels, Bioprod. Biorefin., 2008, 2, 304–318 CrossRef CAS PubMed.
- H. K. Rashedul, H. H. Masjuki, M. A. Kalam, A. M. Ashraful, M. M. Rashed, I. Sanchita and T. Shaon, RSC Adv., 2014, 4, 64791–64797 RSC.
- J. Pullen and K. Saeed, Renewable Sustainable Energy Rev., 2012, 16, 5924–5950 CrossRef CAS PubMed.
- B. R. Moser, G. Knothe and S. C. Cermak, Energy Environ. Sci., 2010, 3, 318–327 CAS.
- K. Yamane, K. Kawasaki, K. Sone, T. Hara and T. Prakoso, Int. J. Engine Res., 2007, 8, 307–319 CrossRef CAS.
- K. Arisoy, Energy Sources, Part A, 2008, 30, 1516–1522 CrossRef CAS.
- W. E. Neff, M. A. El-Agaimy and T. L. Mounts, J. Am. Oil Chem. Soc., 1994, 71, 1111–1116 CrossRef CAS.
- A. M. Ashraful, H. H. Masjuki, M. A. Kalam, H. K. Rashedul, H. Sajjad and M. J. Abedin, Energy Convers. Manage., 2014, 87, 48–57 CrossRef CAS PubMed.
- S. Schober and M. Mittelbach, Eur. J. Lipid Sci. Technol., 2005, 107, 268–271 CrossRef CAS PubMed.
- M. Mofijur, H. Masjuki, M. Kalam and M. Shahabuddin, Energy Educ. Sci. Technol., Part A, 2012, 30, 737e748 Search PubMed.
- A. Monyem, M. Canakci and J. H. Van Gerpen, Appl. Eng. Agr., 2000, 16, 373–378 CrossRef.
- R. L. McCormick, Empirical Study of the Stability of Biodiesel and Biodiesel Blends, DIANE Publishing, 2009, pp. 1–85 Search PubMed.
- J. V. Gerpen, B. Pruszko, R. Clements and G. D. Knothe, Biodiesel Production Technology, National Renewable Energy Laboratory, 2004, NREL/SR-510-36244 Search PubMed.
- M. L. M. Valle, R. S. Leonardo and J. Dweck, J. Therm. Anal. Calorim., 2014, 116, 113–118 CrossRef PubMed.
- W. T. Wazilewski, R. A. Bariccatti, G. I. Martins, D. Secco, S. N. M. D. Souza, H. A. Rosa and L. I. Chaves, Ind. Crops Prod., 2013, 43, 207–212 CrossRef CAS PubMed.
- M. Serrano, A. Bouaid, M. Martínez and J. Aracil, Fuel, 2013, 113, 50–58 CrossRef CAS PubMed.
- M. Mofijur, H. H. Masjuki, M. A. Kalam and A. E. Atabani, Energy, 2013, 55, 879–887 CrossRef CAS PubMed.
- M. Mofijur, H. Masjuki, M. Kalam, M. Hazrat, A. Liaquat, M. Shahabuddin and M. Varman, Renewable Sustainable Energy Rev., 2012, 16, 5007–5020 CrossRef PubMed.
- M. Mofijur, A. Atabani, H. Masjuki, M. Kalam and B. Masum, Renewable Sustainable Energy Rev., 2013, 23, 391–404 CrossRef CAS PubMed.
- I. Shancita, H. Masjuki, M. Kalam, I. R. Fattah, M. Rashed and H. Rashedul, Energy Convers. Manage., 2014, 88, 794–807 CrossRef CAS PubMed.
- J. A. Waynick, Characterization of biodiesel oxidation and oxidation products, National Renewable Energy Laboratory, SwRI® Project No. 08-10721, 2005 Search PubMed.
- A. Sarin, N. P. Singh, R. Sarin and R. K. Malhotra, Energy, 2010, 35, 4645–4648 CrossRef CAS PubMed.
- R. O. Dunn, Energy Fuels, 2008, 22, 657–662 CrossRef CAS.
- L. M. Das, D. K. Bora, S. Pradhan, M. K. Naik and S. N. Naik, Fuel, 2009, 88, 2315–2318 CrossRef CAS PubMed.
- J. Xin, H. Imahara and S. Saka, Fuel, 2009, 88(2), 282–286 CrossRef CAS PubMed.
- G. Knothe, Fuel Process. Technol., 2005, 86(10), 1059–1070 CrossRef CAS PubMed.
- M. W. Formo, E. Jungermann, F. Noris and N. O. V. Sonntag, Bailey's industrial oil and fat products, John Wiley and Sons, 1979, no. 1, vol. 4, pp. 698–711 Search PubMed.
- D. F. Church and W. A. Pryor, Environ. Health Perspect., 1985, 64, 111–126 CrossRef CAS.
- F. D. Gunstone and T. P. Hilditch, J. Chem. Soc., 1945, 836–841 RSC.
- G. Knothe, J. Am. Oil Chem. Soc., 2002, 79, 847–854 CrossRef CAS.
- W. E. Neff, T. L. Mounts, W. M. Rinsch and H. Konishi, J. Am. Oil Chem. Soc., 1993, 70, 163–168 CrossRef CAS.
- A. A. Refaat, Int. J. Environ. Sci. Technol., 2009, 6, 677–694 CrossRef CAS.
- M. Canakci, A. Monyem and J. Van Gerpen, Trans. ASAE, 1999, 42, 1565–1572 CrossRef CAS.
- M. Mittelbach and S. Gangl, J. Am. Oil Chem. Soc., 2001, 78, 573–577 CrossRef CAS.
- P. Bondioli, A. Gasparoli, L. Della Bella and S. Tagliabue, Eur. J. Lipid Sci. Technol., 2002, 104, 777–784 CrossRef CAS.
- P. Bondioli, A. Gasparoli, L. Della Bella, S. Tagliabue and G. Toso, Eur. J. Lipid Sci. Technol., 2003, 105, 735–741 CrossRef CAS PubMed.
- P. Bondioli, A. Gasparoli, A. Lanzani, E. Fedeli, S. Veronese and M. Sala, J. Am. Oil Chem. Soc., 1995, 72, 699–702 CrossRef CAS.
- Y. Takei, I. Miyata, K. Tsurutani and M. Okada, SAE paper 2004-01-3031, 2004 Search PubMed.
- K. Andersson and H. Lingnert, J. Am. Oil Chem. Soc., 1998, 75, 1041–1046 CAS.
- L. M. Du Plessis, J. B. M. De Villiers and W. H. Van Der Walt, J. Am. Oil Chem. Soc., 1985, 62, 748–752 CrossRef CAS.
- C. Y. Lin, H. A. Lin and L. B. Hung, Fuel, 2006, 85, 1743–1749 CrossRef CAS PubMed.
- G. Yildiz, R. L. Wehling and S. L. Cuppett, J. Am. Oil Chem. Soc., 2003, 80(2), 103–107 CrossRef CAS PubMed.
- R. A. Korus, T. L. Mousetis and L. Lloyd, American Society of Agricultural Engineering 1982, pp. 18–223 Search PubMed.
- R. L. McCormick and S. R. Westbrook, Energy Fuels, 2009, 24, 690–698 CrossRef.
- J. C. Cowan, Encyclopedia of chemical technology, Wiley Interscience, 3rd edn, 1979, vol. 8, pp. 130–150 Search PubMed.
- M. I. Gurr, J. L. Harwood and K. Frayn, Lipid biochemistry, Blackwell Science Ltd, 5th edn, 2002 Search PubMed.
- R. L. McCormick, M. Ratcliff, L. Moens and R. Lawrence, Fuel Process. Technol., 2007, 88, 651–657 CrossRef CAS PubMed.
- H. Hasenhuettle, Food emulsifiers and their applications, Chapman and Hall, New York, 1997 Search PubMed.
- J. N. Chacón, P. Gaggini, R. S. Sinclair and F. J. Smith, Chem. Phys. Lipids, 2000, 107, 107–120 CrossRef.
- V. M. L. dos Santos, J. A. B. da Silva, L. Stragevitch and R. L. Longo, Fuel, 2011, 90, 811–817 CrossRef PubMed.
- M. J. Ramos, C. M. Fernández, A. Casas, L. Rodríguez and Á. Pérez, Bioresour. Technol., 2009, 100, 261–268 CrossRef CAS PubMed.
- Y. C. Liang, C. Y. May, C. S. Foon, M. A. Ngan, C. C. Hock and B. Yusof, Fuel, 2006, 85, 867–870 CrossRef CAS PubMed.
- S.-K. Loh, S.-M. Chew and Y.-M. Choo, J. Am. Oil Chem. Soc., 2006, 83, 947–952 CrossRef CAS.
- T. Kivevele, A. K. Agarwal, T. Gupta and M. Mbarawa, Oxidation Stability of Biodiesel Produced from Non-Edible Oils of African Origin, SAE Technical Paper, 2011 Search PubMed.
- M. Mittelbach and S. Schober, J. Am. Oil Chem. Soc., 2003, 80, 817–823 CrossRef CAS.
- J. Xin, H. Imahara and S. Saka, Fuel, 2009, 88, 282–286 CrossRef CAS PubMed.
- A. K. Domingos, E. B. Saad, W. W. Vechiatto, H. M. Wilhelm and L. P. Ramos, J. Braz. Chem. Soc., 2007, 18, 416–423 CrossRef CAS PubMed.
- A. Fröhlich and S. Schober, J. Am. Oil Chem. Soc., 2007, 84, 579–585 CrossRef.
- R. Sarin, M. Sharma, S. Sinharay and R. K. Malhotra, Fuel, 2007, 86, 1365–1371 CrossRef CAS PubMed.
- A. Sarin, Biodiesel: Production and Properties, The Royal Society of Chemistry, 2012, pp. 51–79 Search PubMed.
- P. Y. Furlan, P. Wetzel, S. Johnson, J. Wedin and A. Och, Spectrosc. Lett., 2010, 43, 580–585 CrossRef CAS.
- M. Conceicüão, V. J. Fernandes Jr, A. Araújo, M. Farias, I. Santos and A. Souza, Energy Fuels, 2007, 21, 1522–1527 CrossRef.
- J. S. Oliveira, R. Montalvão, L. Daher, P. A. Z. Suarez and J. C. Rubim, Talanta, 2006, 69, 1278–1284 CrossRef CAS PubMed.
- G. Knothe, J. Am. Oil Chem. Soc., 2001, 78, 1025–1028 CrossRef CAS PubMed.
- F. C. C. Oliveira, C. R. R. Brandão, H. F. Ramalho, L. A. F. da Costa, P. A. Z. Suarez and J. C. Rubim, Anal. Chim. Acta, 2007, 587, 194–199 CrossRef CAS PubMed.
- I. P. Soares, T. F. Rezende, R. C. Silva, E. V. R. Castro and I. C. P. Fortes, Energy Fuels, 2008, 22, 2079–2083 CrossRef CAS.
- P. Baptista, P. Felizardo, J. C. Menezes and M. J. N. Correia, Anal. Chim. Acta, 2008, 607, 153–159 CrossRef CAS PubMed.
- P. Felizardo, P. Baptista, J. C. Menezes and M. J. N. Correia, Anal. Chim. Acta, 2007, 595, 107–113 CrossRef CAS PubMed.
- P. Baptista, P. Felizardo, J. C. Menezes and M. J. Neiva Correia, Talanta, 2008, 77, 144–151 CrossRef CAS PubMed.
- G. Knothe, J. Am. Oil Chem. Soc., 1999, 76, 795–800 CrossRef CAS PubMed.
- G. Knothe, J. Am. Oil Chem. Soc., 2000, 77, 489–493 CrossRef CAS PubMed.
- G. F. Zagonel, P. Peralta-Zamora and L. P. Ramos, Talanta, 2004, 63, 1021–1025 CrossRef CAS PubMed.
- AOCS Official and Tentative Methods: Cd 12–57, Fat stability-active oxygen method (AOM) reapproved, 1997.
- S. G. Morris, P. Magidman, F. E. Luddy and R. W. Riemenschneider, J. Am. Oil Chem. Soc., 1956, 33, 353–355 CrossRef CAS.
- E. R. Sherwin, J. Am. Oil Chem. Soc., 1971, 49(8), 468–472 CrossRef.
- E. R. Sherwin and B. M. Luckadoo, J. Am. Oil Chem. Soc., 1970, 47, 19–23 CrossRef CAS.
- M. W. Läubli and P. A. Bruttel, J. Am. Oil Chem. Soc., 1986, 63, 792–795 CrossRef.
- S. E. Hill and E. G. Perkins, J. Am. Oil Chem. Soc., 1995, 72, 741–743 CrossRef CAS.
- S. Jain and M. Sharma, Renewable Sustainable Energy Rev., 2010, 14, 1937–1947 CrossRef CAS PubMed.
- ASTM Standard D6468 2008, (2013), Standard test method for high temperature stability of middle distillate fuels, ASTM International, West Conshohocken, PA; 2003, DOI:10.1520/D6468, http://www.astm.org, (2014).
- Z. Yaakob, B. N. Narayanan, S. Padikkaparambil, K. S. Unni and P. M. Akbar, Renewable Sustainable Energy Rev., 2014, 35, 136–153 CrossRef CAS PubMed.
- Determining the oxidation stability of biodiesel, European standard EN 14112.
- D. Berthiaume and A. Tremblay, Study of the Rancimat Test Method in Measuring the Oxidation Stability of Biodiesel Ester and Blends, Oleotek Inc., November 2006 Search PubMed.
- Prepared by System Lab Services Division of Williams Pipe Line Company, February 1997.
- K. Yamane, K. Kawasaki, K. Sone and T. Prakoso, Int. J. Engine Res., 2007, 8, 307–319 CrossRef CAS.
- N. T. Joyner and J. E. McIntyre, Oil Soap, 1938, 15, 184–186 CAS.
- M. Formo, E. Jungermann, F. Norris and N. Sonntag, Bailey's Industrial Oil and Fat Products, John Wiley & Sons, Inc., Canada, Fourth Edn, 1979, vol. 1 Search PubMed.
- O. C. Johnson and F. A. Kummerow, J. Am. Oil Chem. Soc., 1957, 34, 407–409 CrossRef CAS.
- H. Wexler, Chem. Rev., 1964, 64, 591–611 CrossRef CAS.
- S. Jain and M. P. Sharma, Renewable Sustainable Energy Rev., 2011, 15, 438–448 CrossRef CAS PubMed.
- Standard test method for high temperature stability of middle distillate fuels, ASTM standard D 6468–08.
- H. Prankl, F. Lacoste, M. MIttelbach and J. Blassnegger, Stability of biodiesel used as a fuel for diesel engines and heating systems, presentation of BIOSTAB project results, Graz, Austria, July 3, 2003 Search PubMed.
- W. B. Wan Nik, F. N. Ani and H. H. Masjuki, Energy Convers. Manage., 2005, 46, 2198–2215 CrossRef CAS PubMed.
- S. Jain and M. P. Sharma, Fuel, 2012, 93, 252–257 CrossRef PubMed.
- C. A. Wilkie, Polym. Degrad. Stab., 1999, 66(3), 301–306 CrossRef CAS.
- T. D. Fornes, P. J. Yoon and D. R. Paul, Polymer, 2003, 44(24), 7545–7556 CrossRef CAS PubMed.
- T. Fornes, P. Yoon and D. Paul, Polymer, 2003, 44, 7545–7556 CrossRef CAS PubMed.
- X. H. Li, Y. Z. Meng, Q. Zhu and S. C. Tjong, Polym. Degrad. Stab., 2003, 81, 157–165 CrossRef CAS.
- I. Buz'as, E. Kurucz and J. Holl'o, J. Am. Oil Chem. Soc., 1979, 56, 685–688 CrossRef.
- Y. H. Roos, J. Therm. Anal. Calorim., 2003, 71, 197–203 CrossRef CAS.
- J. Magoshi, M. A. Becker, Z. Han and S. Nakamura, J. Therm. Anal. Calorim., 2003, 70(3), 833–839 CrossRef.
- B. Kowalski, J. Therm. Anal., 1988, 34, 1321–1326 CrossRef CAS.
- B. Kowalski, Thermochim. Acta, 1991, 184, 49–57 CrossRef CAS.
- H. Gloria and J. M. Aguilera, J. Agric. Food Chem., 1998, 46, 1363–1368 CrossRef CAS.
- C. P. Tan and Y. B. Che Man, Food Chem., 1999, 67, 177–184 CrossRef CAS.
- P. Relkin, S. Sourdet and P. Y. Fosseux, J. Therm. Anal. Calorim., 2003, 71, 187–195 CrossRef CAS.
- M. L. Felsner and J. R. Matos, An. Assoc. Bras. Quim., 1998, 47(4), 308–312 CAS.
- C. P. Tan and Y. B. Che Man, J. Am. Oil Chem. Soc., 2000, 77, 143–155 CrossRef CAS PubMed.
- E. Gimzewski, Thermochim. Acta, 1990, 170, 97–105 CrossRef CAS.
- M. B. Katan, P. L. Zock and R. P. Mensink, Annu. Rev. Nutr., 1995, 15, 473–493 CrossRef CAS PubMed.
- D. E. Williard, T. L. Kaduce, S. D. Harmon and A. A. Spector, J. Lipid Res., 1998, 39, 978–986 CAS.
- R. E. Tate, K. C. Watts, C. A. W. Allen and K. L. Wilkie, Fuel, 2006, 85, 1010–1015 CrossRef CAS PubMed.
- T. Fujii, P. Khuwijitjaru, Y. Kimura and S. Adachi, Food Chem., 2006, 94, 341–347 CrossRef CAS PubMed.
- A. Bouaid, M. Martinez and J. Aracil, Fuel, 2007, 86, 2596–2602 CrossRef CAS PubMed.
- P. Bondioli and A. Gasparoli, Eur. J. Lipid Sci. Technol., 2003, 735–741 CrossRef CAS PubMed.
- A. Sarin, R. Arora, N. P. Singh, M. Sharma and R. K. Malhotra, Energy, 2009, 34, 1271–1275 CrossRef CAS PubMed.
- D. P. Geller, T. T. Adams, J. W. Goodrum and J. Pendergrass, Fuel, 2008, 87, 92–102 CrossRef CAS PubMed.
- L. Das, D. K. Bora, S. Pradhan, M. K. Naik and S. Naik, Fuel, 2009, 88, 2315–2318 CrossRef CAS PubMed.
- E. O. Aluyor, K. O. Obahiagbon and M. Ori-Jesu, Sci. Res. Essays, 2009, 4, 543–548 Search PubMed.
- B. K. Sharma, P. A. Z. Suarez, J. M. Perez and S. Z. Erhan, Fuel Process. Technol., 2009, 1265–1271 CrossRef CAS PubMed.
- F. D. Gunstone, The chemistry of fats and oilsBlackwell Publishing, CRC Press, Oxford, UK, 2004, pp. 150–168 Search PubMed.
- J. C. Thompson, C. L. Peterson, D. L. Reece and S. M. Beck, Trans. ASAE, 1998, 41, 931–939 CrossRef CAS.
- G. Knothe and R. O. Dunn, J. Am. Oil Chem. Soc., 2003, 80, 1021–1026 CrossRef CAS.
- G. Bessee and J. Fey, SAE paper 971690, 1997 Search PubMed.
- H. Prankl, Stability of biodiesel, Presentation of BIOSTAB project results, Amsterdam, The Netherlands, 2002 Search PubMed.
- D. Berthiaume and A. Tremblay, Test Concept for Advanced Oxidation Techniques, Oleotek Inc., 2006 Search PubMed.
- V. Makarevičienė, S. Lebedevas, P. Rapalis, M. Gumbyte, V. Skorupskaite and J. Žaglinskis, Fuel, 2014, 120, 233–239 CrossRef PubMed.
- C. Haşimoǧlu, M. Ciniviz, I. Özsert, Y. Içingür, A. Parlak and M. Sahir Salman, Renewable Energy, 2008, 33, 1709–1715 CrossRef PubMed.
- M. Lapuerta, J. Rodríguez-Fernández, A. Ramos and B. Álvarez, Fuel, 2012, 93, 391–396 CrossRef CAS PubMed.
- J. M. Nzikou, L. Matos, J. E. Moussounga, C. B. Ndangui, N. P. Pambou-Tobi, E. M. Bandzouzi, A. Kimbonguila, M. Linder and S. Desobry, Res. J. Appl. Sci., 2009, 4, 94–100 CAS.
- R. O. Dunn, J. Am. Oil Chem. Soc., 2002, 79, 915–920 CrossRef CAS.
- S. Tagliabue, A. Gasparoli, L. Della Bella and P. Bondioli, Influenza della contaminazione mettalica sulla stabilità térmico ossidativa del biodiesel, La Rivista Italiana Delle Sostanze Grasse LXXXII (marzo/aprile 2005), 2005, pp. 93–96 Search PubMed.
- Y. F. Wang, K. L. Huang, C. T. Li, H. H. Mi, J. H. Luo and P. J. Tsai, Atmos. Environ., 2003, 37, 4637–4643 CrossRef CAS PubMed.
- N. O. V. Sonntag, Composition and Characteristics of Individual Fats and Oils, 1979, p. 289 Search PubMed.
- A. S. M. A. Haseeb, S. Y. Sia, M. A. Fazal and H. H. Masjuki, Energy, 2010, 35, 1460–1464 CrossRef CAS PubMed.
- A. Dos Reis Albuquerque, J. Maul, J. Pereira Dos Santos, I. Maria Garcia Dos Santos and A. Gouveia De Souza, Fuel, 2012, 102, 585–591 CrossRef PubMed.
- C. C. Garcia, P. I. B. M. Franco, T. O. Zuppa, N. R. A. Filho and M. I. G. Leles, J. Therm. Anal. Calorim., 2007, 87, 645–648 CrossRef CAS.
- J. Xin, H. Imahara and S. Saka, Fuel, 2008, 87, 1807–1813 CrossRef CAS PubMed.
- S. Jain and M. P. Sharma, Fuel, 2011, 90, 3014–3020 CrossRef CAS PubMed.
- A. Sarin, R. Arora, N. P. Singh, R. Sarin and R. K. Malhotra, Energy, 2010, 35, 3449–3453 CrossRef CAS PubMed.
- L. M. J. M. Nzikou, J. E. Moussounga, C. B. Ndangui, N. P. Pambou-Tobi and E. M. Bandzouzi, et al., Res. J. Appl. Sci., 2009, 4(2), 94–100 Search PubMed.
- S. Jain and M. P. Sharma, Fuel, 2013, 109, 379–383 CrossRef CAS PubMed.
- J. Polavka, J. Paligová, J. Cvengroš and P. Šimon, J. Am. Oil Chem. Soc., 2005, 82, 519–524 CrossRef CAS PubMed.
- R. O. Dunn, J. Am. Oil Chem. Soc., 2009, 79, 915–920 CrossRef.
- I. M. Atadashi, M. K. Aroua, A. R. Abdul Aziz and N. M. N. Sulaiman, Renewable Sustainable Energy Rev., 2012, 16, 3456–3470 CrossRef CAS PubMed.
- P. P. Oh, H. L. N. Lau, J. Chen, M. F. Chong and Y. M. Choo, Renewable Sustainable Energy Rev., 2012, 16, 5131–5145 CrossRef CAS PubMed.
- A. Demirbaş and H. Kara, Energy Sources, Part A, 2006, 28, 619–626 CrossRef.
- D. Y. C. Leung and Y. Guo, Fuel Process. Technol., 2006, 87, 883–890 CrossRef CAS PubMed.
- Y. C. Chen, H. E. Si and J. Cheng, J. Yangtze Univ., Nat. Sci. Ed., Sci. Eng., 2007, 1, 015 Search PubMed.
- I. M. Atadashi, M. K. Aroua and A. A. Aziz, Renewable Sustainable Energy Rev., 2010, 14, 1999–2008 CrossRef CAS PubMed.
- B. S. Cooke, C. Abrams and B. Bertram, Purification of biodiesel with adsorbent materials, CA Pat. CA2,541,327, 2005.
- A. Gabelman and S. T. Hwang, J. Membr. Sci., 1999, 159, 61–106 CrossRef CAS.
- R. McCormick, M. Ratcliff, L. Moens and R. Lawrence, Fuel Process. Technol., 2007, 88 Search PubMed.
- G. Knothe, Fuel Process. Technol., 2007, 88, 669–677 CrossRef CAS PubMed.
- A. Sarin, R. Arora, N. Singh, R. Sarin and R. Malhotra, Energy, 2010, 35, 3449–3453 CrossRef CAS PubMed.
- G. Karavalakis and S. Stournas, Energy Fuels, 2010, 24, 3682–3686 CrossRef CAS.
- I. M. Rizwanul Fattah, H. H. Masjuki, M. A. Kalam, M. Mofijur and M. J. Abedin, Energy Convers. Manage., 2014, 79, 265–272 CrossRef CAS PubMed.
- I. M. Rizwanul Fattah, H. H. Masjuki, M. A. Kalam, M. A. Wakil, A. M. Ashraful and S. A. Shahir, Energy Convers. Manage., 2014, 83, 232–240 CrossRef CAS PubMed.
- F. Shahidi, P. Janitha and P. Wanasundara, Crit. Rev. Food Sci. Nutr., 1992, 32, 67–103 CrossRef CAS PubMed.
- P. TenáHave, Trans. Faraday Soc., 1947, 43, 201–210 RSC.
- Z. Yang, B. P. Hollebone, Z. Wang, C. Yang and M. Landriault, Fuel Process. Technol., 2013, 106, 366–375 CrossRef CAS PubMed.
- I. M. Rizwanul Fattah, H. H. Masjuki, M. A. Kalam, M. A. Hazrat, B. M. Masum, S. Imtenan and A. M. Ashraful, Renewable Sustainable Energy Rev., 2014, 30, 356–370 CrossRef CAS PubMed.
- M. Gordon, in Food antioxidants, Springer, 1990, pp. 1–18 Search PubMed.
- G. Miller and F. Quackenbush, J. Am. Oil Chem. Soc., 1957, 34, 249–250 CrossRef CAS.
- J.-h. Lee, J.-I. Choe, S.-w. Jung and S.-W. Ham, Bull. Korean Chem. Soc., 2006, 27, 33–34 CrossRef CAS.
- J. Sheehan, V. Camobreco, J. Duffield, M. Graboski and H. Shapouri, Life Cycle Inventory of Biodiesel and Petroleum Diesel for Use in an Urban Bus, National Renewable Energy Laboratory, Golden, CO, 1998, pp. 206–261 Search PubMed.
- A. Wojdyło, J. Oszmiański and R. Czemerys, Food Chem., 2007, 105, 940–949 CrossRef PubMed.
- D. Lomonaco, F. J. N. Maia, C. S. Clemente, J. P. F. Mota, A. E. Costa Jr and S. E. Mazzetto, Fuel, 2012, 97, 552–559 CrossRef CAS PubMed.
- V. C. Ramalho and N. Jorge, Antioxidantes utilizados em óleos, gorduras e alimentos gordurosos, Quim. Nova, 2006, 29(4), 755–760 CrossRef CAS PubMed.
- http://www.biofuelsystems.com/other/baynox.pdf, 2014.
- http://www.istc.illinois.edu/research/092910symposium/0905.pdf, 2014.
- S. Schober and M. Mittelbach, Eur. J. Lipid Sci. Technol., 2004, 106, 382–389 CrossRef CAS PubMed.
- M. Wijtmans, D. A. Pratt, J. Brinkhorst, R. Serwa, L. Valgimigli, G. F. Pedulli and N. A. Porter, J. Org. Chem., 2004, 69, 9215–9223 CrossRef CAS PubMed.
- Y. H. Chen and Y. M. Luo, Fuel Process. Technol., 2011, 92, 1387–1393 CrossRef CAS PubMed.
- C. D. Bannister, C. J. Chuck, M. Bounds and J. G. Hawley, Proc. Inst. Mech. Eng., Part D, 2011, 225, 99–114 CrossRef CAS.
- L. Haya, A. M. Mainar, J. I. Pardo and J. S. Urieta, Phys. Chem. Chem. Phys., 2014, 16, 1409–1414 RSC.
- G. Karavalakis, D. Hilari, L. Givalou, D. Karonis and S. Stournas, Energy, 2011, 36, 369–374 CrossRef CAS PubMed.
- Z. Wang, F. Yang, H. Zheng, X. Qin, J. Luo, Y. Li and D. Xiao, Analyst, 2014, 139, 3622–3628 RSC.
- A. K. Agarwal and D. Khurana, Fuel Process. Technol., 2013, 106, 447–452 CrossRef CAS PubMed.
- D. Chaithongdee, J. Chutmanop and P. Srinophakun, Kasetsart J.: Nat. Sci., 2010, 44, 243–250 CAS.
- M. Mittelbach and S. Schober, J. Am. Oil Chem. Soc., 2003, 80, 817–823 CrossRef CAS.
- A. Fröhlich and S. Schober, J. Am. Oil Chem. Soc., 2007, 84, 579–585 CrossRef.
- D. K. Bora, L. M. Das and M. K. G. Babu, Journal of Scientific and Industrial Research, 2009, 68, 149–152 CAS.
- A. K. Domingos, E. B. Saad, W. W. D. Vechiatto, H. M. Wilhelm and L. P. Ramos, J. Braz. Chem. Soc., 2007, 18 Search PubMed.
- Y. C. Liang, C. Y. May, C. S. Foon, M. A. Ngan, C. C. Hock and Y. Basiron, Fuel, 2006, 85, 867–870 CrossRef CAS PubMed.
- P. Udomsap, N. Chollacoop, S. Topaiboul and T. Hirotsu, International Journal of Renewable Energy, 2009, 4, 47–59 Search PubMed.
- M. Chakraborty and D. C. Baruah, Fuel Process. Technol., 2012, 98, 51–58 CrossRef CAS PubMed.
- T. T. Kivevele, M. M. Mbarawa, A. Bereczky, T. Laza and J. Madarasz, Fuel Process. Technol., 2011, 92, 1244–1248 CrossRef CAS PubMed.
- D. M. Fernandes, D. S. Serqueira, F. M. Portela, R. M. N. Assunção, R. A. A. Munoz and M. G. H. Terrones, Fuel, 2012, 97, 658–661 CrossRef CAS PubMed.
- S. M. Mudge and G. Pereira, Spill Sci. Technol. Bull., 1999, 5, 353–355 CrossRef CAS.
- D. Y. C. Leung, B. C. P. Koo and Y. Guo, Bioresour. Technol., 2006, 97, 250–256 CrossRef CAS PubMed.
- I. Lee, L. A. Johnson and E. G. Hammond, J. Am. Oil Chem. Soc., 1995, 72, 1155–1160 CrossRef CAS.
- J. Van Gerpen, B. Shanks, R. Pruszko, D. Clements and G. Knothe Biodiesel production technology. Report for the National Renewable Energy Laboratory USA: Department of Energy, 2004, vol. 30, p. 42 Search PubMed.
- G. Knothe, Energy Environ. Sci., 2009, 2, 759–766 CAS.
- D. Laforgia and V. Ardito, Bioresour. Technol., 1995, 51, 53–59 CrossRef CAS.
- A. Monyem, J. H. Van Gerpen and M. Canakci, Trans. ASAE, 2001, 44, 35–42 CrossRef CAS.
- C. Peterson and D. Reece, Trans. ASAE, 1996, 39, 805–816 CrossRef CAS.
- K. McDonnell, S. Ward, J. J. Leahy and P. McNulty, J. Am. Oil Chem. Soc., 1999, 76, 539–543 CrossRef CAS.
- S. Gan and H. K. Ng, Energy Convers. Manage., 2010, 51, 1536–1546 CrossRef CAS PubMed.
- T. T. Kivevele, L. Kristóf, A. Bereczky and M. M. Mbarawa, Fuel, 2011, 90, 2782–2789 CrossRef CAS PubMed.
- E. Ileri and G. Koçar, Energy Convers. Manage., 2013, 76, 145–154 CrossRef CAS PubMed.
- S. Jain and M. P. Sharma, Fuel, 2013, 106, 152–156 CrossRef CAS PubMed.
- K. Ryu, Bioresour. Technol., 2010, 101, S78–S82 CrossRef CAS PubMed.
- K. Varatharajan, M. Cheralathan and R. Velraj, Fuel, 2011, 90, 2721–2725 CrossRef CAS PubMed.
- S. M. Palash, M. A. Kalam, H. H. Masjuki, M. I. Arbab, B. M. Masum and A. Sanjid, Energy Convers. Manage., 2014, 77, 577–585 CrossRef CAS PubMed.
- K. Varatharajan and M. Cheralathan, Fuel Process. Technol., 2013, 106, 526–532 CrossRef CAS PubMed.
- K. Ryu, Bioresour. Technol., 2010, 101, S78–S82 CrossRef CAS PubMed.
- M. M. Roy, W. Wang and M. Alawi, Energy Convers. Manage., 2014, 84, 164–173 CrossRef CAS PubMed.
- G. Balaji and M. Cheralathan, Energy Sources, Part A, 2013, 35, 831–839 CrossRef CAS.
- I. M. Rizwanul Fattah, H. H. Masjuki, M. A. Kalam, M. A. Wakil, H. K. Rashedul and M. J. Abedin, Ind. Crops Prod., 2014, 57, 132–140 CrossRef CAS PubMed.
- A. Atmanlı, E. İleri and B. Yüksel, Energy Convers. Manage., 2014, 81, 312–321 CrossRef PubMed.
- T. Kivevele, L. Kristof, A. Bereczky and M. Mbarawa, Fuel, 2011, 90, 2782–2789 CrossRef CAS PubMed.
|
This journal is © The Royal Society of Chemistry 2015 |