DOI:
10.1039/C4RA14286A
(Paper)
RSC Adv., 2015,
5, 13239-13245
Controlled synthesis of monodisperse silver nanoparticles supported layered double hydroxide catalyst†
Received
11th November 2014
, Accepted 24th December 2014
First published on 6th January 2015
Abstract
Silver nanoparticles supported layered double hydroxides (Ag NPs–LDH) were synthesized via a facile chemical reduction–deposition method. The successive anion exchange of the carbonate ions of ZnAl-(CO32−) LDH by chloride and citrate ions leads to citrate intercalated LDH (Cit-LDH). On hydration, the citrate anions in Cit-LDH can attract Ag+ ions by a superficial electrostatic force of attraction and reduce them to Ag nanoparticles. The preferential deposition of monodisperse Ag NPs was obtained on the outer surfaces of pillared LDH. The simultaneous formation of large colloidal Ag NPs of 25–30 nm in size in the aqueous phase was also found exterior to the LDH gallery. The nanoparticles deposited on the LDH surfaces are highly dispersed with a narrow size distribution of ∼2.5 nm and are stabilized on the LDH support. The Ag NPs deposited LDH nanosheets were characterized by X-ray diffraction (XRD), transmission electron microscopy (TEM) and Fourier transform infrared (FTIR) spectroscopy. The resulting monodisperse Ag NPs deposited LDH materials offer heterogeneous interactions at the interface and exhibit high catalytic activity on alcohol oxidation.
Introduction
Nanoscale silver particles (Ag NPs) have received considerable attention from researchers as a potential functional material because of their unique optical, electronic and catalytic properties.1–6 Ag NPs exhibit unique surface plasmon resonance due to the excitation of the collective oscillations of conducting electrons in the visible spectral region.7–9 This surface plasmon resonance frequency can effectively be tuned from the visible to the near infrared (NIR) region by controlling the size, shape and dielectric environment of the nanoparticles. Such tunable properties make silver nanoparticles very attractive for a variety of electrocatalytic applications.10,11 In recent years, many efforts have been made to fabricate noble metal based heteronanostructures, such as core–shell, bimetallic alloy or matrix based hybrid nanostructures, with superior performances compared to individual nanoparticles.12–14 In matrix supported metal nanostructures, it is believed that the strong metal–matrix interaction plays a crucial role in controlling the size, dispersion, stability and the resulting catalytic performance. Layered materials, typically layered double hydroxides (LDHs) have proven to be a competent matrix material for the fabrication of host guest hybrid nanostructures.15–19 LDHs are inorganic lamellar compounds with the chemical formula [Ma(II)1−xMb(III)x(OH)2]x+(Ax/n)n− × mH2O, consisting of positively charged brucite-like layers and exchangeable interlayer anions. In the brucite-like [Mg(OH)2] structure, divalent octahedrally co-ordinated M(II) ions are partially substituted by the isomorphous trivalent M(III) ion, which yields positively charged layers. The extra charges in the interlayer on isomorphous replacement are counterbalanced by charge balancing anions. The remaining free space in the interlayer is filled with water molecules.20 In LDH, the hydroxide sheets are stacked with strong interactions due to their high intergallery charge density. Recently, LDHs as a host matrix has found applications in various fields, typically in heterogeneous catalysis, sensors, polymer composites, and optical devices. Due to their properties such as intercalation property, non-toxicity, high stability and biocompatibility.21–27 LDHs have also been used as supports in heterogeneous reaction media for the preparation of nanoparticles. The fabrication of novel nanostructures with highly dispersed nanoparticles of definite shapes and sizes, immobilized on a suitable support, still remains a challenging goal. Different methods have been adopted to prepare nanoparticle supported LDH hybrids e.g., the reduction of intercalated metal ions by polyol and AuCl4 by citrate process, the layer-by-layer (LBL) assembly technique, electrodeposition of metal nanoparticles, and pulse laser deposition.28–32 In all of these techniques, first a metal salt is intercalated between the LDH sheets and then external reducing agents are used for the preparation of nanoparticles.
In this work, a new reduction–deposition method is reported for the synthesis of Ag NPs supported LDH. The carbonate ions present in the intergallery region of ZnAl-LDH was replaced successively with chloride ions and citrate ions through the ion exchange method to synthesize citrate intercalated LDHs. The citrate ion containing LDHs were used as a reducing support to produce a Ag nanoparticle deposited LDH matrix. The schematic of the synthesis is given in Fig. 1. The structural and morphological characterisation of the ion exchanged LDH and Ag NP deposited LDH were performed by X-ray diffraction (XRD), transmission electron microscopy (TEM), Fourier-transform infrared spectroscopy (FTIR) and UV-vis analysis. The catalytic activity of the resulting AgNPs–LDH materials was investigated by alcohol oxidation.
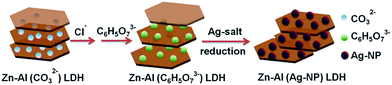 |
| Fig. 1 Schematic representation of the fabrication of Ag nanoparticle supported ZnAl-LDH nanosheets by the reduction deposition method. | |
Experimental section
Materials
Zinc nitrate hexahydrate (Zn(NO3)2·6H2O), aluminium nitrate nonahydrate (Al(NO3)3·9H2O), silver nitrate (AgNO3) and sodium carbonate (Na2CO3) were purchased from Sigma-Aldrich Chemical Co. All the chemicals were ACS reagent grade chemicals. Sodium hydroxide (NaOH) and tri-sodium citrate (Na3C6H5O7) were acquired from Alfa Aesar Chemical Co. All chemicals were used without further purification. All solutions were prepared with deionized water from a Milli-Q Millipore system (>18.0 MΩ cm) and was used in all the experimental processes. The other chemicals used were of analytical grade.
Preparation of ZnAl-(CO32−) LDH
The two dimensional ZnAl-(CO32−) LDH was prepared following a standard co-precipitation and thermal crystallization method of mixed metal ions from an alkaline solution. In a typical preparation, a 50 ml aqueous solution of a stoichiometric amount of Zn(NO3)2·6H2O (5.95 g, 0.02 mol) and Al(NO3)3·9H2O (3.75 g, 0.01 mol) was slowly added into 50 ml of NaOH (2 g, 0.05 mol) and Na2CO3 (1.059 g, 0.01 mol) aqueous solution. During the addition, the solution's pH and temperature was adjusted to 9 ± 1 with 1 mol L−1 of NaOH at 80 °C, respectively. The resulting white precipitate was then aged for 18 h with continuous stirring. Afterward, the residue was filtered and washed with deionised water until the pH of the supernatant solution was neutral. Thus prepared product was first dried in air at room temperature for 24 h and then in an oven at 70 °C for 24 h to form a white powder.
Anion exchange of ZnAl-(CO32−) LDH by Cl−
The anion exchange to ZnAl-(CO32−) LDH was performed by the de-intercalation of the carbonate ions from the as prepared LDH by treating it with a salt–acid mixed solution.33 2 g of the ZnAl-(CO32−) LDH sample was added into 70 ml of aqueous solution containing 5.58 g of NaCl and 0.12 g of HCl, after which the mixture was purged with Ar gas and stirred for 12 h at room temperature. It was then filtered, washed with deionised water and anhydrous ethanol several times and kept in a desiccator for 24 h.
Anion exchange of ZnAl-(Cl−) LDH by citrate (C6H5O73−)
The citrate ion exchanged ZnAl-LDH was prepared similarly to the chloride exchange method. The inorganic citrate anion (C6H5O73−) readily replaces the weak chloride ion. 1 g of the ZnAl-(Cl−) LDH sample was added into 70 ml of aqueous solution containing 8.823 g of tri-sodium-citrate (0.03 mol) and was stirred for 10 h at room temperature. It was then filtered, washed with deionised water and anhydrous ethanol several times and finally it was kept in a desiccator for 24 h.
Preparation of Ag nanoparticle deposited Zn-Al LDH
Monodisperse Ag nanoparticle supported LDH was prepared by the reduction of an aqueous silver salt solution by intercalated citrate ions. In a typical synthesis, 1 L of 0.170 g (1 mmol) of AgNO3 solution in DI water was heated to boiling at 90 °C. Then, 1.0 g of citrate exchanged LDH was added and stirred for 30 min. Finally, the heat was removed to complete the reaction. The resulting precipitate was collected by repeated centrifugation and washing with water and finally it was stored in a desiccator.
Catalytic study
The catalytic dehydrogenation of benzylic alcohols was carried out using a closed reaction vessel. First, 2.5 mmol of benzylic alcohol and 20 ml p-xylene were placed in the reaction vessel, and then pure N2 gas was purged into the mixture for 2 h to remove the air in the reaction system. Then typically, 0.50 g of the powdery catalyst was added into the reaction mixture and heated to 110 °C with stirring. After the complete disappearance of the starting benzylic alcohol, as indicated by thin layer chromatography (TLC), the catalyst was separated by centrifugation and the yield was determined by GC analysis. Finally, the solvent was removed from the solution under vacuum to produce colourless oil as the product.
Characterization
The morphology of the as prepared ZnAl-LDH and Ag nanoparticle deposited ZnAl-LDH was observed by a JEOL 2100 high resolution transmission electron microscope (HRTEM) operated at 200 kV. TEM samples were prepared by drop casting from a dilute dispersion in ethanol on carbon coated Cu-grid with 200 mesh sizes. The samples were dried overnight in air before characterization. Powder X-ray diffraction (XRD) was performed using a Bruker-D8 Advance X-ray diffractometer (40 kV/40 mA) with Ni-filtered Cu Kα radiation (0.1542 nm) passed through a 0.04 rad Soller slit, a 1.0 mm fixed mask with a 1.0° divergence slit, and a 0.2° anti-scatter slit. The pattern was collected in the 2θ range of 5°–70° at a scan rate of 1° min−1 with a step size of 0.02° and counting time of 1 s per step. The slit width and accelerating voltage employed during the scans were uniform for all the samples. Fourier transform infrared (FTIR) analysis was performed using a Perkin-Elmer L 120-000A spectrometer and the spectra were recorded over the wavenumber range 400–4000 cm−1 with the scan speed of 200 nm min−1 using the KBr disc method. Twenty scans were collected and averaged for each spectrum. UV-vis measurements were recorded using a SHIMADZU UV-1601 PC spectrophotometer.
Results and discussion
X-ray diffraction (XRD)
The XRD patterns of the as synthesized ZnAl-(CO32−) LDH, ZnAl-(Cl−) LDH, ZnAl-(citrate) LDH and Ag nanoparticle supported ZnAl-LDH are shown in Fig. 2. The reflection peaks of the ZnAl-(CO32−) LDH in Fig. 2(a) have been indexed according to the JCPDS X-ray diffraction file (no. 89-5424). The XRD peaks of ZnAl-(CO32−) LDH are indexed as the rhombohedral structure of LDH, where carbonate is the interlayer anion. The basal (003) reflection that appears at 2θ ∼ 12.03 degree corresponds to the d003 of 0.74 nm, which is in agreement with the intercalation of carbonate as the main compensating anion (Wu et al., 2005).34 The absence of other crystalline phases indicates the formation of a well-crystallized brucite-like LDH phase. On the decarbonative ion exchange of CO32− with Cl−, the LDH exhibits a slight increase in the layer spacing d003-value to 0.78 nm as shown in Fig. 2(b). The intense basal reflection at lower angles suggests that the decarbonation was successful owing to the Cl− intercalation and the ZnAl-(Cl−) LDH is highly crystalline with interlayer expansion, which is consistent with a previously reported work.35 The XRD profile of the citrate anion exchanged ZnAl-(Cit) LDH from the as obtained ZnAl-(Cl−) LDH is shown in Fig. 3(c). After the replacement of the Cl− ion by the citrate anion, the characteristic basal reflection shifts to a lower angle with an increase in interlayer spacing to 0.86 nm. Such an increase in gallery height is ascribed to the replacement of the small chloride anions by carboxylic containing large citrate anions in ZnAl-(Cit) LDH. It is also observed that other lower angle peaks are shifted and deformed as a result of the citrate ion intercalation process, which indicates the decrease in crystallinity. The poor intensities and broadening of the 003 and 006 reflections as we move from chloride to citrate are seen, which was observed after the anionic exchange and this has been attributed to a disturbance in the stacking sequence of the layers along the c-axis in Cit-LDH.36 Furthermore, the peak broadening observed may result from the hydration of the citrate anion exchanged LDH, where citrate anions offer enhanced swelling to partial delamination because of their high water affinity. This is an agreement in terms of the preference of the H-bonding of citrate ions with water molecules rather than the hydroxyl groups of the metal hydroxide layer.37 The XRD pattern of the Ag nanoparticle supported ZnAl-LDH in Fig. 2(d) shows the re-appearance of crystalline phases of LDH with distinct (003), (006), (009) and other higher angle reflections. The basal d003 spacing of the Ag nanoparticle supported LDH is 0.74 nm, which suggests shrinkage in the gallery height of the LDH stacks. The positions of all the reflection peaks, including the d003 peak, resembles the carbonate intercalated ZnAl-LDH. Such a decrease in layer spacing may occur due to the decomposition of citrate ions in the LDH and restoration with the interlayer carbonate anions.
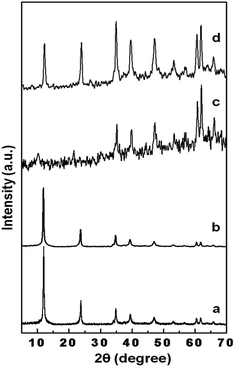 |
| Fig. 2 XRD patterns of (a) ZnAl-(CO32−) LDH, (b) ZnAl-(Cl−) LDH, (c) ZnAl-(citrate) LDH and (d) the Ag NPs deposited ZnAl-LDH. | |
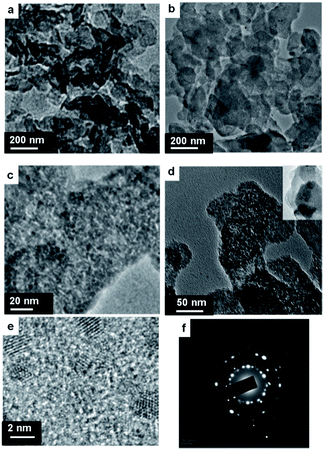 |
| Fig. 3 TEM image of (a) the as prepared ZnAl-(CO32−) LDH (b and c) citrate exchanged ZnAl-LDH nanosheet at low magnification and high magnification. TEM images of (d) Ag nanoparticle deposited ZnAl-LDH nanocomposites after citrate reduction. (e and f) HRTEM image of Ag nanoparticles and corresponding SAED pattern. Inset of (d) shows the stacking of LDHs. | |
Transmission electron microscopy (TEM)
TEM observations on the sample was employed to confirm the formation of Ag nanoparticles on the LDH sheets. TEM image of ZnAl-(CO32−) in Fig. 3(a) shows a thin platelet of morphologically irregular LDH hexagonal nanosheets stacked one upon another. The lateral size of the citrate exchanged LDH in Fig. 3(b) ranges between 100 and 200 nm. Upon citrate ion intercalation, the hexagonal LDH crystallites are more irregular with stacking faults observed. The high magnification image in Fig. 3(c) shows the presence of citrate moieties on the LDH sheets, which are bright in appearance because of the contrast differences between organic molecules and inorganic surfaces. TEM image in Fig. 3(d) shows monodisperse Ag nanoparticles deposited on the LDH surface treated with 1 mmol AgNO3 aqueous solution. The onset image exhibits the retension of the stacking layers of the LDHs on the deposition of Ag nanoparticles. The citrate ion intercalated LDHs produce a superficial interaction site with Ag+ ions in aqueous solution and reduce them to monodisperse Ag nanoparticles. A few monodisperse Ag NPs from the LDH surfaces are also removed on sonication for 5 min, which suggests the weak interaction between the LDH surfaces and deposited nanoparticles (ESI S1†). The HRTEM image in Fig. 3(e) represents the Ag nanoparticles of ∼2.5 nm in size with a 0.23 nm lattice distance corresponding to the (111) lattice spacing of cubic structured silver. The corresponding SAED image in Fig. 3(f) indicates the formation of polycrystalline Ag nanoparticles. Concurrently during the autoreduction of the Ag+ ions at elevated temperature directly by citrate ions, neutral Ag nanoparticle nucleation and growth occurs in the aqueous phase outside the intergallery region with different geometries to the colloidal nanoparticles of ∼25–30 nm and are preferably stabilized with dicarboxylate anions (ESI S2†). Despite the formation of large colloidal nanoparticles in the aqueous solution, a few large Ag nanoparticles also grow at the boundary of the LDH sheets (ESI S3†).
This further leads to the narrow layer separation after the deposition of the Ag nanoparticles.
Fourier transform infrared spectroscopy (FTIR)
To prove the ion exchange of the carbonate by the chloride and citrate ions successively between the layers, FT-IR spectra were recorded. FT-IR spectra of the ZnAl-(CO32−) LDH, chloride exchanged ZnAl-(CO32−) LDH and citrate exchanged ZnAl-(Cl−) LDH samples are given in Fig. 4. All of the samples show a broad intense band at 3450 cm−1 corresponding to the O–H stretching frequency. ZnAl-(CO32−) LDH shows a strong absorption band at 1372 cm−1, which is attributed to the stretching mode of the carbonate molecules within the LDH lamellae. The anion exchange of carbonate with chloride in ZnAl-(Cl−) LDH exhibits the O–H bending vibration of the interlayer water at 1630 cm−1. The IR spectrum does not exhibit any characteristic absorption in the range of 1000–1500 cm−1 as expected for the IR inactive Cl− intercalated LDH. There is no absorption at 1372 cm−1 which confirms indirectly the replacement of the CO32− ions with Cl− ions in the LDH. Further ion exchange of Cl− with citrate in ZnAl-(citrate) LDH shows the characteristic absorptions of the carboxylate group of the citrate ligands. The asymmetric νas(COO) and symmetric νs(COO) vibrations appear at 1595 cm−1 and 1393 cm−1, respectively. However, the spectrum of AgNPs–LDH shows an intense peak at 1367 cm−1, which is attributed to the stretching frequency of the carbonate anions. The bands recorded below 800 cm−1 are due to the vibration of the metal–oxygen bond (MII–O and MIII–OH) in the brucite-like lattice.38,39
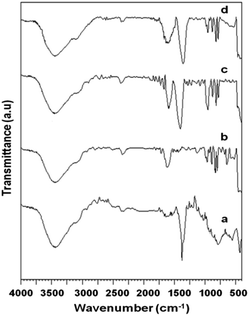 |
| Fig. 4 FTIR spectra of (a) ZnAl-(CO32−) LDH, (b) ZnAl-(Cl−) LDH, and (c) ZnAl-(citrate) LDH and (d) Ag NPs–LDH. | |
UV-vis study
The UV-vis absorbance results of the Ag nanoparticle deposited ZnAl-LDH is shown in Fig. 5. The absorption spectrum of the Ag nanoparticles on LDH in Fig. 5(a) shows a characteristic band at ∼390 nm, which is typical of surface plasmon resonance bands due to the collective oscillation of the electrons in the conduction band of the Ag atoms in the ∼2.5 nm Ag nanoparticles. The weak absorption in the blue region arises as the particle size is reduced to the quantum regime. A slight broadening in the surface plasmon resonance band can be ascribed to the strong electron scattering at the different dielectric interfaces of the Ag NPs and LDHs arising from their ultrasmall sizes. The UV-vis absorbance also recorded for the colloidal Ag nanoparticle suspension in aqueous solution, which was separated from the LDH layers by a careful sonication–centrifugation method. The spectrum exhibits the characteristic absorbance band around 414 nm arising from the surface plasmon resonance of the ∼25 nm diameter Ag NPs. These large Ag nanoparticles are grown at the boundary of the LDH sheets and are separated during high energy centrifugation. The colloidal Ag nanoparticle aqueous suspension is well dispersed and stable for more than one month when stored at room temperature, as shown in the inset of Fig. 5(b).
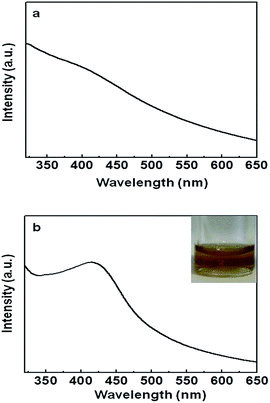 |
| Fig. 5 UV absorption spectra of (a) Ag nanoparticle deposited ZnAl-LDH and (b) colloidal large Ag nanoparticles separated from LDH during sonication. Inset in the figure show the corresponding image of the suspension. | |
Mechanism of Ag nanoparticle deposition
The intercalation of the citrate anions in the interlayer space of the LDH was evaluated by XRD, where the interlayer spacing increases as the large carboxylic groups of the citrate moiety displaces Cl− in the host layer. The Cit-LDH is believed to swell with increased layer spacing upon hydration in aqueous solution. The extensive swelling of LDH is mainly due to the superior hydration of carboxylate groups in citrate through H-bonding with the water molecules present in the LDH.37,40 In the course of the citrate ion mediated reaction it is believed that the Ag+ ions are reduced to Ag nanoparticles while the citrate ions are oxidised to carbon dioxide and dicarboxylate anions (ESI S4†).41 Carbon dioxide thus evolved during decomposition process can lead to the formation of interlayer carbonate species due to the basic hydroxide layers in the Ag NPs supported ZnAl-LDH.38 The representative (003), (006), (009) reflections in the XRD patterns show a noteworthy variation in relative intensity and their position remain almost identical with respect to ZnAl-(CO32−) LDH. The structural changes may occur due to the deformation and stacking faults of the layers. The deposition of monodisperse Ag nanoparticles on the LDH surface can be described by a silver oxide (Ag2O) mediated reduction by citrate ions as indicated in eqn (1)–(3). A small amount of insoluble Ag2O could be formed at room temperature after Ag+ ions form a complex with the significant number of OH− groups present on the LDH surface. Ag2O particles during heating may form soluble Ag(OH)x such as Ag(OH) and Ag(OH)2− on hydrolysis. The Ag NPS deposited on the LDH surface through the reduction of Ag(OH)x with citrate anions was followed by nucleation to yield monodisperse Ag NPs.42,43 |
Ag+ + OH⋯LDH → AgOH⋯LDH → Ag2O⋯LDH
| (1) |
|
(AgOH)x + LDH-citrate → Ag NPs–LDH
| (3) |
Catalytic activity on hydrogenation
The catalytic activity of the Ag nanoparticle deposited LDH prepared by the reduction–deposition method was investigated using the dehydrogenation of benzyl alcohol to benzaldehyde at 110 °C in p-xylene. The catalyst is stable to the catalytic reaction because the nanoparticles are well dispersed and embedded on the LDH layers, which prevents sintering or aggregation under the abovementioned reaction conditions. The improvement of their stability is achieved through the surface confinement effect of the metal NPs on the LDH surfaces. The catalytic performances of the Ag nanoparticle supported LDH in the oxidant free dehydrogenation of benzyl alcohol is shown in Fig. 6. Benzyl alcohol was oxidised to benzaldehyde with over 90% yields within only 6 h of reaction time in the presence of the catalyst. The effective dehydrogenation of benzylic alcohols with different substituents (entries 1–4) in the presence of the Ag nanoparticle supported LDH catalyst is given Table 1. In all the cases, the catalyst gives a high yield of carbonyl compounds. The dehydrogenation process of benzyl alcohol on the Ag NPs supported LDH catalyst surface is further confirmed by the reaction with the bare LDH. The treatment of the benzyl alcohol in the bare LDH without Ag nanoparticles did not give any products. From the table it is also clear that the electronic nature of the group present on the benzene ring has no significant effect on this oxidation procedure. The presence of electron donating (entry 2 and 3) as well as electron-withdrawing (entry 4) groups on the benzene ring produced very similar results. The Ag NP deposited LDH materials offer specific interactions at the interface which facilitate the catalytic reactivity of the AgNPs towards alcohol oxidation.44
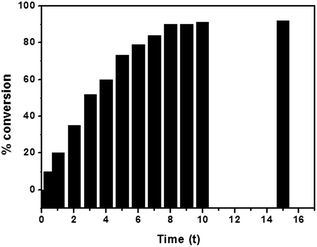 |
| Fig. 6 UV benzyl alcohol conversion time course for the Ag nanoparticle supported LDH catalysts prepared by the reduction deposition method. | |
Table 1 Catalytic behaviours of the Ag nanoparticle supported LDH catalysts for the dehydrogenation of various alcoholsa
Entry |
Substrate |
Product |
Time (h) |
Yield [%] |
Reaction conditions, 0.50 g; T = 110 °C; substrate, 2.5 mmol; p-xylene, 20 ml. |
1 |
 |
 |
8 h |
90 |
2 |
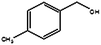 |
 |
12 h |
92 |
3 |
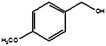 |
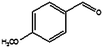 |
10 h |
90 |
4 |
 |
 |
10 h |
85 |
Conclusions
In summary, silver nanoparticle deposited layered double hydroxides (Ag NPs–LDH) was prepared by a facile reduction–deposition method. Citrate intercalated LDH was produced by the successive ion exchange of carbonate with chloride and citrate anion. The citrate ion exchanged LDHs reduces Ag+ ions to Ag NPs. The Ag nanoparticles of approximately 2.5 nm deposited on the LDH sheets are highly dispersed throughout the LDH surface. The Ag nanoparticle deposited LDH prepared in this process shows high catalytic performance in the dehydrogenation of benzylic alcohol to aldehydes.
Acknowledgements
The authors are grateful to the Department of Science and Technology (DST) for the financial support.
References
- H. Acharya, J. Sung, B.-H. Sohn, D.-H. Kim, K. Tamada and C. Park, Chem. Mater., 2009, 21, 4248 CrossRef CAS.
- M. B. Cortie and A. M. McDonagh, Chem. Rev., 2011, 111, 3713 CrossRef CAS PubMed.
- M.-C. Daniel and D. Astruc, Chem. Rev., 2004, 104, 293 CrossRef CAS PubMed.
- P. V. Kamat, J. Phys. Chem. B, 2002, 106, 7729 CrossRef CAS.
- C. Noguez and I. L. Garzón, Chem. Soc. Rev., 2009, 38, 757–771 RSC.
- K. Tschulik, C. B. McAuley, H.-S. Toh, E. J. E. Stuart and R. G. Compton, Phys. Chem. Chem. Phys., 2014, 16, 616 RSC.
- L. M. Liz-Marzan, Langmuir, 2006, 22, 32 CrossRef CAS PubMed.
- M. Rycenga, P. H. C. Camargo, W. Li, C. H. Moran and Y. Xia, J. Phys. Chem. Lett., 2010, 1, 696 CrossRef CAS PubMed.
- A. Sivanesan, H. K. Ly, J. Kozuch, M. Sezer, U. Kuhlmann, A. Fischer and I. M. Weidinger, Chem. Commun., 2011, 47, 3553 RSC.
- M.-H. Lee, H. Cai and I.-M. Hsing, Analyst, 2005, 130, 364 RSC.
- A. Mignani, B. Ballarin, M. Giorgetti, E. Scavetta, D. Tonelli, E. Boanini, V. Prevot, C. Mousty and A. Iadecola, J. Phys. Chem. C, 2013, 117, 16221 CAS.
- H. Acharya, J. Sung, T. H. Kim, D.-H. Kim and C. Park, Chem.–Eur. J., 2012, 18, 14695 CrossRef CAS PubMed.
- W. Schärtl, Nanoscale, 2010, 2, 829 RSC.
- H. Zhang, L. Cao, W. Liu, G. Su, R. Gao and Y. Zhao, Dalton Trans., 2014, 43, 4822 RSC.
- H. Ai, X. Huang, Z. Zhu, J. Liu, Q. Chi, Y. Li, Z. Li and X. Ji, Biosens. Bioelectron., 2008, 24, 1048 CrossRef CAS PubMed.
- S. Gago, M. Pillinger, R. A. S. Ferreira, L. D. Carlos, T. M. Santos and I. S. Gonçalves, Chem. Mater., 2005, 17, 5803 CrossRef CAS.
- E. Scavetta, S. Stipa and D. Tonelli, Electrochem. Commun., 2007, 9, 2838 CrossRef CAS PubMed.
- Y. Yuan and W. Shi, Appl. Clay Sci., 2012, 67–68, 83–90 CrossRef CAS PubMed.
- X. Zhao, F. Zhang, S. Xu, D. G. Evans and X. Duan, Chem. Mater., 2010, 22, 3933 CrossRef CAS.
- G. R. Williams and D. O'Hare, J. Mater. Chem., 2006, 16, 3065 RSC.
- H. Acharya, S. K. Srivastava and A. K. Bhowmick, Compos. Sci. Technol., 2007, 67, 2807 CrossRef CAS PubMed.
- W. Fang, Q. Zhang, J. Chen, W. Deng and Y. Wang, Chem. Commun., 2010, 46, 1547 RSC.
- Z. Gu, B. E. Rolfe, Z. P. Xu, A. C. Thomas, J. H. Campbell and G. Q. M. Lu, Biomaterials, 2010, 31, 5455 CrossRef CAS PubMed.
- X. X. Guo, F. Z. Zhang, D. G. Evans and X. Duan, Chem. Commun., 2010, 46, 5197 RSC.
- A. I. Khan and D. O'Hare, J. Mater. Chem., 2002, 12, 3191 RSC.
- X. Li, J. P. Liu, X. X. Ji, J. A. Jiang, R. M. Ding, Y. Y. Hu, A. Z. Hu and X. T. Huang, Sens. Actuators, B, 2010, 147, 241 CrossRef CAS PubMed.
- V. Rives, M. del Arco and C. Martín, Appl. Clay Sci., 2014, 88–89, 239–269 CrossRef CAS PubMed.
- B. Ballarin, A. Mignani, E. Scavetta, M. Giorgetti, D. Tonelli, E. Boanini, C. Mousty and V. Prevot, Langmuir, 2012, 28, 15065 CrossRef CAS PubMed.
- A. Matei, R. Birjega, A. Vlad, C. Luculescu, G. Epurescu, F. Stokker-Cheregi, M. Dinescu, R. Zavoianu and O. D. Pavel, Appl. Phys. A: Mater. Sci. Process., 2013, 110, 841 CrossRef CAS.
- Y. Wang, D. Zhang, M. Tang, S. Xu and M. Li, Electrochim. Acta, 2010, 55, 4045 CrossRef CAS PubMed.
- G. Wu, L. Wang, D. G. Evans and X. Duan, Eur. J. Inorg. Chem., 2006, 3185 CrossRef CAS.
- B. Yang, Z. Yang, R. Wang and Z. Feng, J. Mater. Chem. A, 2014, 2, 785 CAS.
- Z. Liu, R. Ma, M. Osada, N. Iyi, Y. Ebina, K. Takada and T. Sasaki, J. Am. Chem. Soc., 2006, 128, 4872 CrossRef CAS PubMed.
- Q. Wu, A. Olafsen, Ø. B. Vistad, J. Roots and P. Norby, J. Mater. Chem., 2005, 15, 4695 RSC.
- N. Iyi, T. Matsumoto, Y. Kaneko and K. Kitamura, Chem. Mater., 2004, 16, 2926 CrossRef CAS.
- C. Gérardin, D. Kostadinova, B. Coq and D. Tichit, Chem. Mater., 2008, 20, 2086 CrossRef.
- Q. Li and R. Kirkpatrick, Am. Mineral., 2007, 92, 397 CrossRef CAS.
- J. C. Villegas, O. H. Giraldo, K. Laubernds and S. L. Suib, Inorg. Chem., 2003, 42, 5621 CrossRef CAS PubMed.
- E. M. Seftel, E. Popovici, M. Mertens, P. Cool and E. F. Vansant, J. Optoelectron. Adv. Mater., 2008, 10, 3477 CAS.
- T. Hibino and W. Kobayashi, J. Mater. Chem., 2005, 15, 653 RSC.
- S. Patra, A. K. Pandey, D. Sen, S. V. Ramagiri, J. R. Bellare, S. Mazumder and A. Goswami, Langmuir, 2014, 30, 2460 CrossRef CAS PubMed.
- S. Nishimura, D. Mott, A. Takagaki, S. Maenosono and K. Ebitani, Phys. Chem. Chem. Phys., 2011, 13, 9335 RSC.
- C. Chen, P. Gunawan, X. W. Lou and R. Xu, Adv. Funct. Mater., 2012, 22, 780 CrossRef CAS.
- T. Mitsudome, Y. Mikami, H. Funai, T. Mizugaki, K. Jitsukawa and K. Kaneda, Angew. Chem., 2008, 120, 144 CrossRef.
Footnote |
† Electronic supplementary information (ESI) available. See DOI: 10.1039/c4ra14286a |
|
This journal is © The Royal Society of Chemistry 2015 |