DOI:
10.1039/C4RA14151B
(Paper)
RSC Adv., 2015,
5, 15461-15468
Fluorinated amphiphilic block copolymers via RAFT polymerization and their application as surf-RAFT agent in miniemulsion polymerization†
Received
9th November 2014
, Accepted 23rd January 2015
First published on 23rd January 2015
Abstract
This investigation reports the preparation of amphiphilic block copolymer (Am-BCP) based on poly(ethylene glycol) methyl ether methacrylate (PEGMA) and 2,2,3,3,4,4,4-heptafluorobutyl acrylate (HFBA) via RAFT polymerization. In this case poly(PEGMA) (PPEGMA) was prepared using 2-cyano-2-propyl dithiobenzoate (CPBT) as a RAFT agent. This PPEGMA was later used as a macro-RAFT agent for the polymerization of HFBA to prepare well-defined block copolymers (BCPs), (PPEGMA-b-PHFBA) of varied lengths and compositions. The BCPs self-assembled in aqueous solution to produce spherical micelles consisting of hydrophobic block PHFBA as a core and a hydrophilic block of PPEGMA as a flexible corona. This Am-BCP formed micelles of different sizes, as characterized by dynamic light scattering (DLS) and TEM analyses. The Am-BCP was later used as a surf-RAFT agent i.e. as a surfactant as well as a macro-RAFT agent for the miniemulsion polymerization of styrene. TEM analysis showed that the synthesized polystyrene emulsion had a core–shell morphology. The particle size and particle size distributions of the polystyrene latex were determined by DLS analysis.
Introduction
Block copolymers (BCPs) are interesting materials, because of their unique properties.1–7 The properties of BCPs can be tuned by the choice of different types of blocks and by designing the length of different blocks.8 The BCPs show different nano-structured morphologies like spherical, cylindrical or vesicles which are formed in selective solvents by varying concentration and chemical composition of different blocks. BCPs are also used as thermoplastic elastomers, surfactants for colloidal systems, compatibilizers for immiscible polymer blends, etc.1,9 Amphiphilic block copolymers (Am-BCP) are a special type of materials which show the combination of both hydrophilic and hydrophobic properties.10–15 They spontaneously aggregate into micelles in suitable solvents, where the less soluble block forms a core surrounded by chains of the more soluble block, which extend into the solvent phase.16–18
Polymeric micelles have different important applications like, as emulsifier, solubilizers and are also used in drug and gene delivery system.19–21 The miceller block copolymer domains are also used as nanoreactors for the synthesis of metallic nanoparticles (like, Ag, Au, Zn, TiO2 nanoparticles).22–26 Among the different Am-BCPs, the BCPs containing polyethylene oxide (PEO) as hydrophilic part and fluoropolymer hydrophobic parts27–33 would be potentially useful, because fluoropolymers have interesting characteristics like improved thermal stability, resistance to water, chemicals etc. Fluoropolymers have lower surface energy and very good hydrophobicity.34 The fluorinated Am-BCPs have strong tendency to form aggregates. Because of hydrophobic nature of fluorinated block Am-BCPs can be used for the delivery of hydrophobic particles.35–37
It is difficult to prepare BCPs having fluoropolymer segments with controlled block lengths, because of the lipophobic as well as hydrophobic nature of the fluorinated segments. The advent of controlled radical polymerization (CRP)38–40 has provided a novel approach to prepare polymers with controlled molecular weights and with well-defined architecture under easy and mild reaction conditions compared to living ionic polymerizations. By CRP methods, it is possible to prepare block copolymer, star shaped polymer and branched polymers.41 Among the different CRP methods reversible-addition fragmentation and chain transfer (RAFT) process has been very compatible with various fluoromonomers in solution as well as in heterogeneous system.42–46
In general, the perfluorinated polymers like PTFE and other polymers with high fluorine content are insoluble in conventional organic solvents.47 However, the partially fluorinated polymers like, fluorinated polyacrylates are soluble in common organic solvent. In addition, polyfluoroacrylates are amorphous in nature having the properties of non-fluorinated polyacrylates and that of fluorinated polymers. For these reasons polyfluoroacrylates are widely used in different applications like surface active agents, surface coatings, as additives etc.48 Moreover, the hydrophobicity and solubility of the polyfluoroacrylates depend on the chain length of the fluoroalkyl pendant group.49–51 The polyfluoroalkyl acrylate with long fluoroalkyl pendant group (number of carbon atoms, C ≥ 6) showed higher hydrophobicity with poor solubility in common organic solvents.48,51
To enhance the properties of polyacrylates, block copolymer with polyfluoroacrylates were synthesized. Several authors have reported the synthesis of fluorinated copolymers like random, graft and block copolymers.45,48,51–58 These block copolymers have the ability to self-assemble into organized micro domain structures in suitable solvents.59–61 The phase-separated nanostructures and incompatibility of the two blocks lead to the self-organization of the fluorinated block copolymer.1
In this investigation we report the preparation of Am-BCP based on PPEGMA as hydrophilic segment and PHFBA as hydrophobic segment via RAFT polymerization. In this case we first prepared the homopolymer of PEGMA via RAFT polymerization and then it was used as macro-RAFT agent to polymerize HFBA. This BCP was used as surfactant as well as macro-RAFT agent for the miniemulsion polymerization of styrene. Polymeric surfactants, especially based on BCPs have several advantages as stabilizer over the low-molecular-weight surfactants in emulsion polymerization.62 The advantages of polymeric surfactant over low molecular weight surfactant are to improve film formation by their polymeric component and to prepare micro latex.63,64 In this approach the prepared Am-BCP was used as surf-RAFT agent for the miniemulsion polymerization of styrene. The Am-BCPs were characterized by gel permeation chromatography (GPC), 1H NMR, UV-Vis spectroscopy, transmission electron microscopy (TEM) and dynamic light scattering (DLS) analyses.
Experimental
Materials
2,2,3,3,4,4,4-Heptafluorobutyl acrylate (HFBA) and poly(ethylene glycol) methyl ether methacrylate (PEGMA) (Mn = 300 gmol−1) were purchased from Sigma-Aldrich, USA, and were purified by passing through basic alumina column to remove the inhibitor. 2,2′-Azobisisobutyronitrile (AIBN) (Sigma-Aldrich, USA) was used as a thermal initiator and was purified by re-crystallization from methanol prior to use. Potassium persulphate (KPS) and 2-cyano-2-propyl dithiobenzoate (CPBT) were purchased from Sigma-Aldrich, USA. The solvent, 1,4-dioxane (Merck, India) was dried over calcium hydride (CaH2) and was purified by passing through basic alumina column prior to use. Tetrahydrofuran (THF) and n-hexane were purchased from Merck, and were used as received.
Characterization
Gel Permeation Chromatography (GPC) analysis was performed to determine the molecular weight and dispersity (Đ) of the polymers. It was carried out at ambient temperature on a Viscotek GPC instrument equipped with a VE 1122 solvent delivery system; RI detector (model VE 3580) and two VIscoGEL mixed bed columns (17392-GMHHRM), which were preceded by a guard column. Data analysis was done by using OmniSEC 4.2 software. The system was calibrated with linear and narrow disperse poly(methyl methacrylate) (PMMA) and THF was used as eluent at a flow rate of 1 mL min−1. The polymer solution in THF was thoroughly filtered using Millipore filter paper of pore size 0.5 μm before injecting into the GPC instrument. The retention time of the polymer solution within the instrument was set for 25 min.
1H NMR spectra of the polymers were performed by dissolving the polymers in CDCl3 and were recorded on a Brucker 400 MHz nuclear magnetic resonance (NMR) instrument with tetramethyl silane (TMS) as the internal standard.
Fourier-transform infrared (FTIR) spectra were recorded on a Perkin-Elmer, Inc. version 5.0.1 spectrophotometer. In this case, the polymer solution in THF was film cast over KBr cells and then FTIR spectra were recorded in the range of 400 to 4000 cm−1.
The particle size of the block copolymer micelles and latex was determined by using DLS analysis. The DLS analysis of the diluted latex was carried out on a Malvern Nano ZS instrument using a 4 mW He–Ne laser (λ = 632.8 nm).
The critical micelle concentration (CMC) of fluorinated Am-BCP was determined from the fluorescence spectra of the aqueous solution of the polymer with varying concentrations from 0.005 to 0.1 mg mL−1. The fluorescence spectra of the solutions containing pyrene probe (∼2 × 10−7 M) were measured on a Hitachi F-7000 spectrophotometer optical system equipped with a 150 W Xe lamp. The samples were excited at 335 nm and the emission spectrum was recorded between 350 and 550 nm with excitation and emission slit widths both set at 2.5 nm respectively.
The morphology of the block copolymer and latex was studied by a TEM instrument (Analytical TEM: FEI™, USA, Type 5022/22, Technai G2 20 S-Twin) operated at an accelerated voltage of 120 kV. A drop of dilute aqueous solution was drop-cast on 300 mesh carbon coated copper grid. The grids were dried in a desiccator before analysis.
DSC analysis was carried out using TA Instrument (DSC Q100 V8.1 Build 251) under nitrogen atmosphere at a heating rate of 10 °C min−1 within a temperature range of −100 °C to +100 °C. TGA was carried out on a TA Instrument (Q50) at a heating rate of 20 °C min−1 within the temperature range of 30 °C to 600 °C under nitrogen atmosphere.
The UV-Vis spectra of the samples were recorded on a UV-Vis spectrophotometer (Perkin-Elmer, Waltham, MA). UV-Vis spectra (absorbance vs. wave length) of the Am-BCPs were recorded in 1,4-dioxane at room temperature (25 °C). The samples were scanned in the wave length region from 200 to 500 nm.
Homopolymerization of PEGMA using 2-cyano-2-propyl dithiobenzoate (CPBT) as RAFT agent
The RAFT agent, CPBT (11.5 mg, 0.053 mmol) and 1,4-dioxane (3 mL) were taken in a Schlenk tube which was purged with nitrogen. PEGMA, monomer (1.5 g, 5.3 mmol) was added to the mixture. Finally, AIBN (1.7 mg, 0.01 mmol) dissolved in a small amount of solvent was added into the reaction tube under nitrogen atmosphere. The reaction tube was heated at 85 °C under constant stirring for 8 h. The polymer obtained was dissolved in THF and was purified by precipitation into hexane. The homopolymer of PEGMA (PPEGMA) was used as macro-RAFT agent for the synthesis of block copolymer with HFBA.
Polymerization of HFBA using PPEGMA as a macro-RAFT agent
In a typical polymerization reaction, PPEGMA, the macro-RAFT agent (147 mg, 4.9 × 10−3 mmol) (Mn,GPC = 30
100 g mol−1) was dissolved in 1,4-dioxane (2 mL) in a Schlenk tube. The monomer, HFBA (500 mg, 1.96 mmol) and the initiator, AIBN (0.08 mg, 5 × 10−4 mmol) were added subsequently to the Schlenk tube under nitrogen atmosphere. The Schlenk tube was purged with nitrogen for 15 min. After that the reaction tube was placed in an oil bath preheated at 75 °C. The reaction was carried out for 10 h. Percent conversion was recorded gravimetrically as 72% and Mn,GPC = 64
000 g mol−1 and Đ = 1.23. Same procedure was followed for the synthesis of block copolymers of varying the PHFBA block length by using different PPEGMA as macro-RAFT agent.
Miniemulsion polymerization of styrene by using PPEGMA-b-PHFBA as surf-RAFT
The block copolymer, PPEGMA-b-PHFBA was used as surfactant as well as macro-RAFT agent for the polymerization of styrene. In this process, PPEGMA37-b-PHFBA35 (Mn = 18
200 g mol−1) (0.22 g, 0.012 mmol) was dissolved in deionised water (2 g) in a Schlenk tube under nitrogen atmosphere. Styrene (0.5 g, 4.8 mmol) was added into the reaction mixture and nitrogen was purged for 15 min. An aqueous solution of KPS (0.84 mg, 0.003 mmol) was then added to the pre-emulsion which was then ultrasonicated in ice water for 15 min. Finally, the Schlenk tube containing the miniemulsion was immersed into an oil bath preheated at 75 °C. The polymerization reaction was carried out for 5 h. The monomer conversion was determined gravimetrically. Conversion = 40%. Mn,GPC = 42
000 g mol−1 and Đ = 1.38.
Results and discussion
PPEGMA was prepared via RAFT polymerization in 1,4-dioxane at 85 °C using CPBT as RAFT agent and AIBN as initiator (Scheme 1). The molar ratio of RAFT agent CPBT and AIBN was 5
:
1. The chemical structure of the PPEGMA was identified by 1H NMR spectroscopy. Fig. 1a shows the 1H NMR spectra of PPEGMA. The resonances at δ = ∼0.8–1.1 and ∼1.7–2.0 ppm were due to the methyl (–CH3) and methylene (–CH2) protons respectively, as designated in Fig. 1a. The intense resonances at δ = 4.1 ppm is due to –OCH2– protons in the pendant group of PPEGMA. There were resonances at 7.2–8.1 ppm for the aromatic protons of the RAFT end group along with the residue protons in CDCl3. The molecular weight of the PPEGMA was calculated by comparing the integral areas at δ = 7.2–7.8 ppm due to RAFT end group and at δ = 4.1 ppm due to –OCH2– protons in PPEGMA. The molecular weights of the PPEGMA were also measured by GPC analysis and they were comparable with the values of Mn,NMR and Mn,theo (Table 1).
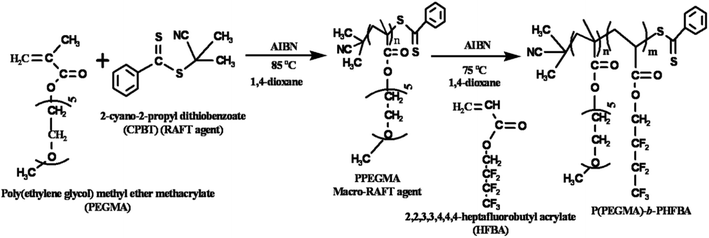 |
| Scheme 1 Synthesis of PPEGMA, macro-RAFT agent and amphiphilic block copolymer, PPEGMA-b-PHFBA. | |
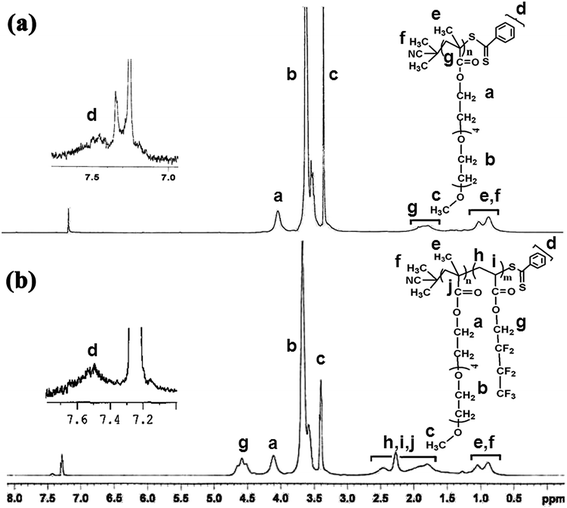 |
| Fig. 1 (a) 1H NMR spectra of PPEGMA (sl. no. 1 of Table 1a) and (b) PPEGMA100-b-PHFBA133 (sl. no. 4 of Table 1b). | |
Table 1 (a) and (b) Summary of PPEGMA and PPEGMA-b-PHFBA prepared via RAFT polymerizationa
(a) |
Sl. no. |
Polymer |
Conversion (%) |
Molar composition HFBA : PPEGMA |
Mn,Theo (g mol−1) |
Mn,GPC (g mol−1) |
Mn,NMR (g mol−1) |
Đ |
*[PPEGMA] : [I] = 10 : 1, where PPEGMA was used as macro-RAFT agent. Reaction time = 10 h. |
1 |
#PPEGMA |
72 |
— |
10 800 |
10 500 |
8400 |
1.26 |
2 |
P(PEGMA)37 b-P(HFBA)35 |
76 |
41 : 59 |
15 200 |
18 200 |
16 700 |
1.18 |
3 |
P(PEGMA)37 b-P(HFBA)77 |
86 |
42 : 58 |
26 000 |
30 600 |
23 000 |
1.45 |
(b) |
Sl. no. |
Polymer |
Conversion (%) |
Molar composition HFBA : PPEGMA |
Mn,Theo (g mol−1) |
Mn,GPC (g mol−1) |
Mn,NMR (g mol−1) |
Đ |
1 |
#PPEGMA |
87 |
— |
26 100 |
30 100 |
26 800 |
1.19 |
2 |
P(PEGMA)100-b-P(HFBA)39 |
67 |
35 : 65 |
34 000 |
40 000 |
34 200 |
1.42 |
3 |
P(PEGMA)100-b-P(HFBA)86 |
63 |
45 : 55 |
50 400 |
55 000 |
51 300 |
1.31 |
4 |
P(PEGMA)100-b-P(HFBA)133 |
72 |
— |
72 000 |
64 000 |
— |
1.23 |
These PPEGMAs were used as macro-RAFT agent for the preparation of block copolymer (BCP) with HFBA (Scheme 1). A series of BCPs were prepared by using PPEGMA as macro-RAFT agent. The molecular weights and dispersity of the different Am-BCPs obtained by GPC analysis are shown in Table 1. The BCPs were characterized by 1H NMR analysis. In the 1H NMR spectrum of the BCP, PPEGMA-b-PHFBA, [Fig. 1b] a new resonance emerged at δ = 4.6 ppm (w.r.t. 1HNMR spectrum of PPEGMA in Fig. 1a) which was attributed to the –OCH2– protons in PHFBA part. There were resonances at 7.2–7.8 ppm for the aromatic protons of the RAFT end group. The molar composition of the Am-BCP was calculated by comparing the resonances of the RAFT end group with the resonances of –OCH2– protons in PPEGMA (δ = 4.1 ppm) and PHFBA (δ = 4.6 ppm) respectively. The molecular weights of PPEGMA as well as PPEGMA-b-PHFBA were determined by GPC analysis using THF as the eluent. Fig. 2 shows the overlapping GPC traces of PPEGMA and the BCP, PPEGMA-b-PHFBA. Complete shift of the GPC traces towards higher molecular weight (lower elution volume) indicates successful preparation of BCP. Relatively narrow dispersity (Đ) confirms the controlled nature of the block copolymerization reaction. The molecular weights and the dispersity (Đ) of the different Am-BCPs obtained via GPC analysis are shown in Table 1. The molecular weights of the BCPs were also determined from 1H NMR spectrum using the integral area of the protons of RAFT end group in BCP (Table 1).
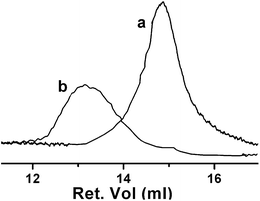 |
| Fig. 2 GPC traces of (a) homopolymer of PEGMA and (b) PPEGMA-b-PHFBA. | |
The presence of end functionality in the homo as well as block copolymers prepared via RAFT polymerization was studied by UV-Vis spectroscopy. Fig. 3 shows the UV-Vis spectra of PPEGMA prepared via RAFT polymerization as well as via conventional free radical polymerization (FRP). The UV-Vis spectroscopy showed the λmax at around 300 nm for the polymers prepared via RAFT. This PPEGMA prepared via FRP did not show any absorbance around 275 nm. This indicates the presence of RAFT end group in the PPEGMA as well as in Am-BCP prepared via RAFT polymerization.65
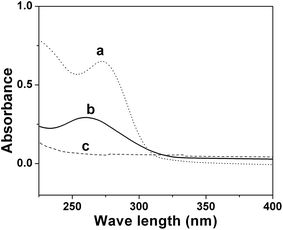 |
| Fig. 3 UV-Vis spectra of (a) PPEGMA and (b) Am-BCP prepared via raft polymerization and (c) PPEGMA prepared via FRP. | |
Fig. 4 depicts the FT-IR spectra of the PPEGMA and PPEGMA-b-PHFBA respectively. The FT-IR spectrum of PPEGMA showed an absorption band at 1725 cm−1 for >C
O stretching. However, the FT-IR spectrum of PPEGMA-b-PHFBA showed two distinct absorption bands at 1725 and 1756 cm−1 for the >C
O stretching frequency of PPEGMA and PHFBA block respectively.
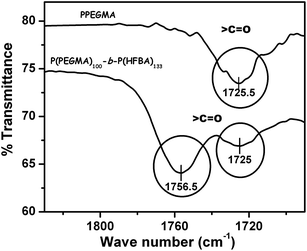 |
| Fig. 4 FT-IR spectra of PPEGMA (sl. no. 1 of Table 1a) and PPEGMA100-b-PHFBA133 (sl. no. 4 of Table 1b). | |
PHFBA is insoluble in water, but the Am-BCPs formed micelles in water, because of the hydrophilic poly(ethylene oxide) (PEO) moiety in the PEPGMA segment.66 The micelle formation of PPEGMA100-b-PHFBA39 block copolymer in aqueous solution was studied by DLS and TEM analyses. Fig. 5 shows the DLS profile of the micelle formed by the block copolymer (sl no. 2 in Table 1b) in aqueous solution (1 mg mL−1). It showed a z-average diameter of 236 nm. Am-BCPs with solubility difference between hydrophilic and hydrophobic segments assembled as polymeric micelles in aqueous solution.67–69 The shell is formed by the PPEGMA block and core is due to PHFBA block.
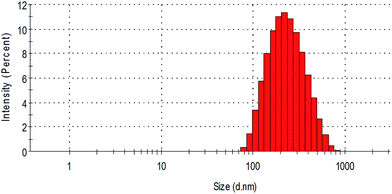 |
| Fig. 5 DLS profile of P(PEGMA)100-b-P(HFBA)39 in aqueous solution (1 mg mL−1). | |
Fig. 6 shows the TEM analysis of the aqueous solution of BCP at different concentrations. It showed the spherical micelles at the BCP concentration of 1 mg mL−1 (Fig. 6a). It shows an average particle size of about 140 nm. This is found to be smaller than the same obtained by DLS analysis which is presumably due to the dried micelle, as carried out by TEM analysis. However, when the concentration of solution increased from 1 mg mL−1 to 5 mg mL−1, the aggregation took place (Fig. 6d).
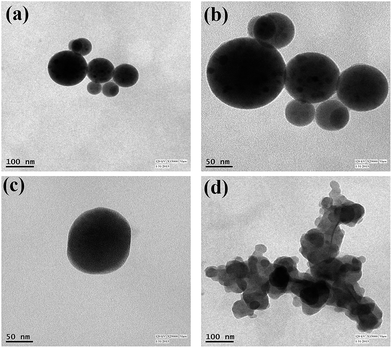 |
| Fig. 6 TEM images of P(PEGMA)100-b-P(HFBA)39 in aqueous solution. (a)–(c) for 1 mg mL−1 of block copolymer aqueous solution and (d) for 5 mg mL−1 of aqueous solution. | |
The DSC traces of the Am-BCP showed two glass transition temperatures (Tg); one at −55 °C for PPEGMA block and another at −23 °C for PHFBA block.70 (DSC traces are shown in the ESI section at Fig. S1†). This indicates the presence of two phases in the Am-BCP. Thermal stability of the Am-BCPs and their respective homopolymers were studied by TGA. Fig. 7(a) and (b) show the TGA and DTG plots of the different polymers respectively. PPEGMA shows two stages of degradation. The first stage degradation occurs within the range 100–300 °C which is probably due to the removal of absorbed water and subsequently, degradation of PEO side chains. At the second stage, main chain degradation of PPEGMA takes place at 308 °C. The maximum degradation temperature (Tmax) of PHFBA is 415 °C (Fig. 7a and b), but the Tmax of its block copolymers with PPEGMA is in between 308 and 415 °C. According to Fig. 7, the thermal stability of the BCP increases (351 to 364 °C), as the block length of PHFBA increases.
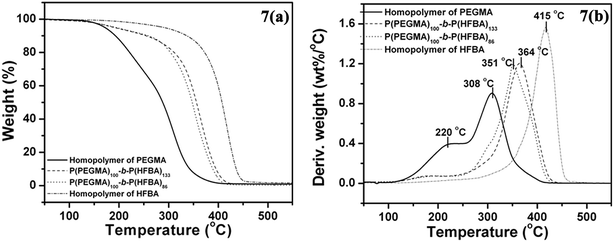 |
| Fig. 7 TGA thermogram (a) and DTG thermogram (b) of the homopolymers (PHFBA and PPEGMA) and PPEGMA-b-PHFBA. | |
Am-BCP as surf-RAFT agent in miniemulsion polymerization
Miniemulsion polymerization of styrene was carried out using PPEGMA37-b-PHFBA35 as surfactant and KPS as initiator (Scheme 2). In water this Am-BCP formed micelles in which PHFBA segment formed the core and PPEGMA formed the hydrophilic corona. The block copolymer showed CMC value of 0.049 mg mL−1, as measured by fluorescence spectroscopy using pyrene as probe. The plot of I1/I3 vs. concentration of Am-BCP, P(PEGMA)37-b-P(HFBA)35 is shown in ESI section in Fig. S2.† Being hydrophobic in nature styrene was inside the micelle and polymerization took place therein.29 Fig. 8 shows the evolution of conversion of styrene with polymerization time. There has been a linear increase in conversion with time.
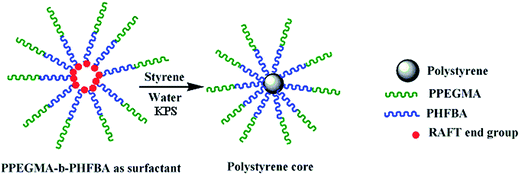 |
| Scheme 2 Schematic representation of miniemulsion polymerization of styrene using Am-BCP as surf-RAFT. | |
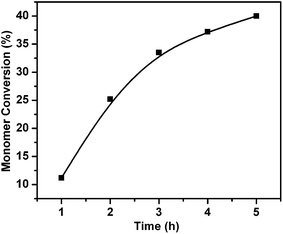 |
| Fig. 8 Conversion versus time plot for the polymerization of styrene using Am-BCP as surf-RAFT agent. | |
The Am-BCPs were readily soluble in water and formed a miceller structure,71 as evident from TEM analysis (Fig. 6). These micelles were utilized as polymerization site for styrene monomer. GPC analysis of the prepared polystyrene showed controlled molecular weight (Mn,GPC = 42
000 g mol−1, Mn,theo = 40
000 g mol−1) and low dispersity (Đ = 1.38). This indicates that the polymerization of styrene using Am-BCP (PPEGMA-b-PHFBA) as macro-RAFT agent was controlled. The resultant polystyrene (PS) latex was stable and has an average particle diameter of around 400 nm, as evidenced by DLS analysis (Fig. 9). DLS analysis showed a considerable increase in particle diameter in PS latex of ∼400 nm (Fig. 9) compared to the micelles of ∼236 nm formed by Am-BCP (Fig. 5). TEM analysis of the synthesized PS latex showed core–shell morphology (Fig. 10). In this case PS formed the core72 and the shell was formed by the Am-BCP. The schematic diagram of the PS latex stabilized by Am-BCP has been shown in Fig. 10c. EDX analysis showed the presence of fluorine atom in the shell of PS latex indicating the presence of Am-BCP in the shell. EDX profile of the sample is shown in ESI Section in Fig. S3.† Thus, the above studies indicate that the Am-BCP acted as surf-RAFT agent i.e. as a macro-RAFT agent in controlling the molecular weight of polystyrene as well as a surfactant for the miniemulsion polymerization of styrene.
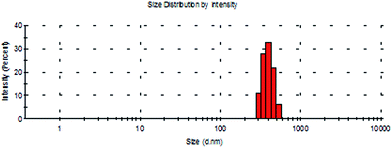 |
| Fig. 9 DLS profiles of the size distribution of polystyrene latex prepared using Am-BCP as surf-RAFT. | |
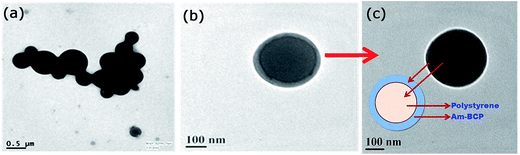 |
| Fig. 10 TEM image of polystyrene latex prepared using Am-BCP as surf-RAFT and schematic representation of core–shell morphology of the polystyrene latex. | |
Conclusions
Amphiphilic block copolymers having PEO moiety as hydrophilic and PHFBA as hydrophobic segment were successfully prepared via RAFT polymerization of HFBA using PPEGMA as macro-RAFT agent. DLS and TEM studies showed that PPEGMA-b-PHFBA aggregates into spherical micelles in aqueous solution. In the micelles, the PHFBA block formed the core and the PPEGMA remained at the shell. The BCPs form well-defined distribution of spherical micelles in aqueous solution. The Am-BCPs were successfully used as surf-RAFT agent for the miniemulsion polymerization of styrene. The Am-BCP provided good stability to the polystyrene latex having core–shell morphology, in which polystyrene was at the core and was surrounded by the Am-BCP in the shell, as observed in TEM analysis. Compared to conventional surfactant and conventional RAFT agent this kind of surf-RAFT agent may lead to the development of new materials for specialty applications.
Acknowledgements
The authors are thankful to IIT Kharagpur and CSIR (New Delhi) for financial supports.
References
- Complex macromolecular architecture: synthesis, characterization and self-assembly, ed. N. Hadjichristidis, A. Hirao, Y. Tezuka and F. D. Prez, John Wiley, New York, 2011 Search PubMed.
- T. P. Lodge, Macromol. Chem. Phys., 2003, 204, 265–273 CrossRef CAS.
- N. K. Singha, A. Kavitha, D. J. Haloi, P. Mandal, A. Janke, D. Jehnichen, H. Komber and B. Voit, Macromol. Chem. Phys., 2012, 213, 2034–2043 CrossRef CAS.
- A. A. Kavitha and N. K. Singha, Macromolecules, 2010, 43, 3193–3205 CrossRef CAS.
- D. J. Haloi, S. Ata, N. K. Singha, D. Jehnichen and B. Voit, ACS Appl. Mater. Interfaces, 2012, 4, 4200–4207 CAS.
- H. Datta and N. K. Singha, J. Polym. Sci., Part A: Polym. Chem., 2008, 46, 3499–3511 CrossRef CAS.
- M. E. Arnold, K. Nagai, R. J. Spontak, B. D. Freeman, D. Leroux, D. E. Betts, J. M. DeSimone, F. A. DiGiano, C. K. S. Tebbins and R. W. Linton, Macromolecules, 2002, 35, 3697–3707 CrossRef CAS.
- H. Datta, A. K. Bhowmick and N. K. Singha, Polymer, 2012, 50, 3259–3268 CrossRef PubMed.
- W. Jakubowski, J.-F. Lutz, S. Slomkowski and K. Matyjaszewski, J. Polym. Sci., Part A: Polym. Chem., 2005, 43, 1498–1510 CrossRef CAS.
- Y. Tu, X. Wan, D. Zhang, Q. Zhou and C. Wu, J. Am. Chem. Soc., 2000, 122, 10201–10205 CrossRef CAS.
- S. A. Jenekhe and X. L. Chen, Science, 1998, 279, 1903–1907 CrossRef CAS.
- D. H. Han and C. Y. Pan, Eur. Polym. J., 2006, 42, 507–515 CrossRef CAS PubMed.
- K. Matsumoto, H. Mazaki and H. Matsuoka, Macromolecules, 2004, 37, 2256–2267 CrossRef CAS.
- H. Hong, Y. Mai, Y. Zhou, D. Yan and J. Cui, Macromol. Rapid Commun., 2007, 28, 591–596 CrossRef CAS.
- S. A. Jenekhe and X. L. Chen, Science, 1999, 283, 372–375 CrossRef CAS.
- P.-H. Tung, S.-W. Kuo, S.-C. Chen, C.-L. Lin and F.-C. Chang, Polymer, 2007, 48, 3192–3200 CrossRef CAS PubMed.
- R. Erhardt, M. Zhang, A. Boker, H. Zettl, C. Abetz, P. Frederik, G. Krausch, V. Abetz and A. H. E. Muller, J. Am. Chem. Soc., 2003, 125, 3260–3267 CrossRef CAS PubMed.
- R. Erhardt, A. Boker, H. Zettl, H. Kaya, W. Pyckhout-Hintzen, G. Krausch, V. Abetz and A. H. E. Muller, Macromolecules, 2001, 34, 1069–1075 CrossRef CAS.
- Y. Zhao, J. Mater. Chem., 2009, 19, 4887–4895 RSC.
- A. W. York, S. E. Kirkland and C. L. McCormick, Adv. Drug Delivery Rev., 2008, 60, 1018–1036 CrossRef CAS PubMed.
- J. Wang, K. Yao, C. Wang, C. Tang and X. Jiang, J. Mater. Chem. B, 2013, 1, 2324–2332 RSC.
- I. W. Hamley, Nanotechnology, 2003, 14, R39–R54 CrossRef CAS.
- S. Bhaviripudi, A. Reina, J. Qi, J. Kong and A. M. Belcher, Nanotechnology, 2006, 17, 5080–5086 CrossRef CAS.
- L. Zhang, H. Niu, Y. Chen, H. Liu and M. Gao, J. Colloid Interface Sci., 2006, 298, 177–182 CrossRef CAS PubMed.
- D. Ray and V. K. Aswal, J. Nanopart. Res., 2012, 14, 778–786 CrossRef.
- D. J. Pochan, J. Zhu, K. Zhang, K. L. Wooley, C. Miesch and T. Emrick, Soft Matter, 2011, 7, 2500–2506 RSC.
- L. He, J. P. Hinestrosa, J. M. Pickel, S. Zhang, D. G. Bucknall, S. M. Kilbey II, J. W. Mays and K. Hong, J. Polym. Sci., Part A: Polym. Chem., 2011, 49, 414–422 CrossRef CAS.
- S. Gupta, R. Tyagi, V. S. Parmar, S. K. Sharma and R. Haaga, Polymer, 2012, 53, 3053–3078 CrossRef CAS PubMed.
- E. Velasquez, J. Rieger, F. Stoffelbach, B. Charleux, F. D'Agosto, M. Lansalot, P.-E. Dufils and J. Vinas, Polymer, 2013, 54, 6547–6554 CrossRef CAS PubMed.
- S. Sosnowski, M. Gadzinowski and S. Slomkowski, Macromolecules, 1996, 29, 4556–4564 CrossRef CAS.
- S. Sosnowski, S. Slomkowski, A. Lorenc and H. R. Kricheldorf, Colloid Polym. Sci., 2002, 280, 107–115 CAS.
- J. W. Bartels, S. I. Cauet, P. L. Billings, L. Y. Lin, J. Zhu, C. Fidge, D. J. Pochan and K. L. Wooley, Macromolecules, 2010, 43, 7128–7138 CrossRef CAS PubMed.
- V. Kumar, B. Gupta, G. Kumar, M. K. Pandey, E. Aiazian, V. S. Parmar, J. Kumar and A. C. Watterson, J. Macromol. Sci., Part A: Pure Appl. Chem., 2010, 47, 1154–1160 CrossRef CAS.
- Fluoropolymers I and II, ed. G. Hougham, P. E. Cassidy, K. Johns and T. Davidson, Kluwer Academic Publishers, USA, 2002 Search PubMed.
- J. He, P. Ni and C. Liu, J. Polym. Sci., Part A: Polym. Chem., 2008, 46, 3029–3041 CrossRef CAS.
- K. Matsuoka and Y. Moroi, Curr. Opin. Colloid Interface Sci., 2003, 8, 227–235 CrossRef CAS.
- M. Miyamoto, K. Aoi and T. Saegusa, Macromolecules, 1989, 22, 3540–3543 CrossRef CAS.
- Controlled and living polymerizations, ed. A. H. E. Muller and K. Matyjaszewski, Wiley-VCH Verlag GmbH & Co. KGaA, Weinheim, Germany, 2009 Search PubMed.
- G. Moad, J. Chiefari, Y. K. Chong, J. Krstina, R. T. A. Mayadunne, A. Postma, E. Rizzardo and S. H. Thang, Polym. Int., 2000, 49, 993–1001 CrossRef CAS.
- J. Chiefari, Y. K. Chong, F. Ercole, J. Krstina, J. Jeffery, T. P. T. Le, R. T. A. Mayadunne, G. F. Meijs, C. L. Moad, G. Moad, E. Rizzardo and S. H. Thang, Macromolecules, 1998, 31, 5559–5562 CrossRef CAS.
- W. A. Braunecker and K. Matyjaszewski, Prog. Polym. Sci., 2007, 32, 93–146 CrossRef CAS PubMed.
- A. Hiraoa, K. Sugiyamaa and H. Yokoyama, Prog. Polym. Sci., 2007, 32, 1393–1438 CrossRef PubMed.
- Handbook of RAFT Polymerization, ed. C. Barner-Kowollik, Wiley-VCH Verlag GmbH & Co. KgaA, Weinheim, Germany, 2008 Search PubMed.
- Z. Qinghua, Z. Xiaoli, C. Fengqiu, S. Ying and W. Qiongyan, J. Polym. Sci., Part A: Polym. Chem., 2007, 45, 1585–1594 CrossRef.
- T.-Y. Guo, D. Tang, M. Song and B. Zhang, J. Polym. Sci., Part A: Polym. Chem., 2007, 45, 5067–5075 CrossRef CAS.
- H. Natanya, J. Katja and H. Soren, Eur. Polym. J., 2007, 43, 255–293 CrossRef PubMed.
- H. Teng, Appl. Sci., 2012, 2, 496–512 CrossRef PubMed.
- I. J. Park, S.-B. Lee and C. K. Choi, Macromolecules, 1998, 31, 7555–7558 CrossRef CAS.
- W. R. Dreher, A. Singh and M. W. Urban, Macromolecules, 2005, 38, 4666–4672 CrossRef CAS.
- M. J. Krupers and M. Möller, J. Fluorine Chem., 1997, 82, 119–124 CrossRef CAS.
- Z. Qinghua, Z. Xiaoli, C. Fengqiu, S. Ying and W. Qiongyan, J. Polym. Sci., Part A: Polym. Chem., 2007, 45, 1585–1594 CrossRef.
- Q. Zhang, Q. Wang, Z. Luo, X. Zhan and F. Chen, Polym. Eng. Sci., 2009, 49, 1818–1824 CAS.
- N. M. L. Hansen, K. Jankova and S. Hvilsted, Eur. Polym. J., 2007, 43, 255–293 CrossRef CAS PubMed.
- Z. Ma and P. Lacroix-Desmazes, J. Polym. Sci., Part A: Polym. Chem., 2004, 42, 2405–2415 CrossRef CAS.
- M. Eberhardt and P. Theato, Macromol. Rapid Commun., 2005, 26, 1488–1493 CrossRef CAS.
- X. Zhou, P. Ni, Z. Yu and F. Zhang, J. Polym. Sci., Part A: Polym. Chem., 2007, 45, 471–484 CrossRef CAS.
- J. Zhou and L. Zhang, J. Chem. Eng., 2013, 223, 8–17 CrossRef CAS PubMed.
- A. Chakrabarty and N. K. Singha, J. Colloid Interface Sci., 2013, 408, 66–74 CrossRef CAS PubMed.
- P. Alexandridis and R. J. Spontak, Curr. Opin. Colloid Interface Sci., 1999, 4, 130–139 CrossRef CAS.
- K. Y. Mya, E. M. J. Lin, C. S. Gudipati, H. B. A. S. Gose and C. He, J. Phys. Chem. B, 2010, 114, 9128–9134 CrossRef CAS PubMed.
- B. Tan, C. Gudipati, H. Hussain, C. He, Y. Liu and T. Davis, Macromol. Rapid Commun., 2009, 30, 1002–1008 CrossRef CAS PubMed.
- N. Yeole and D. Hundiwale, Colloids Surf., A, 2011, 392, 329–334 CrossRef CAS PubMed.
- Y. Luo, J. Tsavalas and F. J. Schork, Macromolecules, 2001, 34, 5501 CrossRef CAS.
- G. Riess, Colloids Surf., A, 1999, 153, 99–110 CrossRef CAS.
- K. Skrabania, A. Miasnikova, A. M. Bivigou-Koumba, D. Zehm and A. Laschewsky, Polym. Chem., 2011, 2, 2074–2083 RSC.
- K. Yu and A. Eisenberg, Macromolecules, 1996, 29, 6359–6361 CrossRef CAS.
- K. Kataoka, A. Harada and Y. Nagasaki, Adv. Drug Delivery Rev., 2001, 47(2001), 113–131 CrossRef CAS.
- Encyclopedia of Surface and Colloid Science, ed. A. T. Hubbard, Marcel Dekker, Inc., New York, 2002 Search PubMed.
- H. Hussain, K. Busse and J. Kressler, Macromol. Chem. Phys., 2003, 204, 936–946 CrossRef CAS.
- B. P. Koiry, M. Moukwa and N. K. Singha, J. Fluorine Chem., 2013, 153, 137–142 CrossRef CAS PubMed.
- L. Zhang and A. Eisenberg, J. Am. Chem. Soc., 1996, 118, 3168–3181 CrossRef CAS.
- N. R. Yeole, D. G. Hundiwale and T. Jana, J. Colloid Interface Sci., 2011, 354, 506–510 CrossRef CAS PubMed.
Footnote |
† Electronic supplementary information (ESI) available. See DOI: 10.1039/c4ra14151b |
|
This journal is © The Royal Society of Chemistry 2015 |
Click here to see how this site uses Cookies. View our privacy policy here.