DOI:
10.1039/C4RA14126A
(Paper)
RSC Adv., 2015,
5, 32572-32579
Temporal changes in horsebean bioavailability and accumulation after removing extractable oxytetracycline fractions in soils
Received
8th November 2014
, Accepted 23rd March 2015
First published on 23rd March 2015
Abstract
Extractable fractions of oxytetracycline in soil affect its bioavailability and accumulation in plants. The objective was to assess the bioavailability of different bound fractions of oxytetracycline available in soil to horsebean. After freshly spiking with oxytetracycline for 24 h, soils were treated with water and mild (0.1 mol L−1 CaCl2) and exhaustive (0.1 mol L−1 Na2EDTA-McIlvaine) extractants as treatments T1, T2, and T3, respectively, to obtain different extractable oxytetracycline fractions. The control was a nonextracted soil. Horsebean was exposed to the abovementioned soils. The results showed that oxytetracycline was accumulated in shoots and roots at 10 days; however, after 10 days, the amount of oxytetracycline in the roots was below its quantification limits (<0.001 mg kg−1). The remained OTC as resistant bound fraction in T3 always exhibited the highest shoot concentration factors (SCF) compared with removing fewer extractable fractions during 14–28 days exposure. Moreover, the effect of horsebean upon oxytetracycline extractability during exposure was also studied. Adding horsebean did not affect the change trend of three extractable fractions in the control, T2, or T3 during exposure, but changed the trend of CaCl2- and total-extractable fractions in T1. Horsebean activity can increase total-extractable concentration in the control, but decrease total-extractable concentration in T1, T2 and T3. The correlation analysis showed that there were close relationships between water-, CaCl2-, total-extractable fractions in soil and root accumulation for the horsebean at 10 days of exposure. The interesting phenomenon was that resistant bound OTC had a high availability, and its ecological risk in soil had been underestimated in previous investigations.
1. Introduction
Tetracycline antibiotics including oxytetracycline (OTC) have been one of the most widely-used veterinary antibiotics in the livestock industry. It has been reported1,2 that above 75% of OTC administered to animals is excreted in an antimicrobially active form in urine or faeces. The agricultural application of untreated or evenly treated antibiotics-containing animal wastes has caused serious contamination in soil.3 At present, they are frequently detected at high concentrations in soils.4–6
To estimate the availability of contaminants, single-chemical extraction techniques are commonly used to extract their different fractions in soil. Moreover the chemical extraction is a relatively simple, one-step procedure.7 Different extractable fractions have different affinities for binding with soil, which determine or govern the mobility and bioavailability of the contaminant.8 OTC exists predominantly as a zwitterion with a positive charge on the tertiary amine functionality and a negative charge on the deprotonated hydroxyl group; thus, chemical extraction would also be suitable to estimate the labile fractions of OTC. In soil, water-extractable fraction represents the most available portion, and it can be stated with a degree of certainty that this fraction is dissolved and mobile in soil solution. Other OTC fractions are sorbed on soil as bound residue fractions. In previous studies, CaCl2 extraction was commonly used as a “mild/soft” extraction method, which was considered to represent the labile fraction of metals that have potential to enter terrestrial organisms.8–12 It has been reported that 0.1 mol L−1 CaCl2 extraction concentrations are consistent for various types of soil, and its extraction concentration is between water and exhaustive extraction.13 0.1 mol L−1 Na2EDTA-McIlvaine (pH, 4.0 ± 0.05), as exhaustive extraction, was applied to extract/remove total extractable fractions containing the dissolved, exchangeable fraction and loosely bound fraction;14 therefore, only the resistant bound fraction was not extracted. Moreover, in our previous study,13 it was also evident that 0.1 mol L−1 CaCl2, as neutralised salt solution, is suitable to extract loosely bound OTC, and that 0.1 mol L−1 Na2EDTA-McIlvaine (pH 4.0 ± 0.05), as exhaustive extraction, can achieve stable and efficient recovery compared with other buffer solutions. However, it cannot extract all OTC present in the soil, and its extraction rate is in the range of 31.97–80.64%.13 Only 43.9% of total OTC was extracted in contaminated soils in our following study, which showed that resistant bound (or non-extractable) OTC still existed in the soil.
Over the past decade, single-chemical extraction methods have been developed to address this issue by attempting to measure bioavailable OTC concentrations in soil.15,16 However, there is very less information on the study of biological methods to assess OTC bioavailability in soil, and it is unclear whether the chemical method gives the measure that is closest to the biological method. At present, it is known that the antibiotics can accumulate in plants from soil (or aqueous) media.17–26 It has been reported that soil-bound antibiotic retained bioavailability toward microorganisms.27,28 However, few studies have been published on the determination of different bound fractions of OTC in soil. Furthermore, very few studies have addressed the potential bioavailability of adsorbed (containing non-extracted and labile-extracted) OTC onto soils to plants. The uncertainty that whether the bound residues with different binding potentials have different bioavailabilities should be studied, and whether the resistant bound fraction has low bioavailability, though it is strongly adsorbed on soil, as we expect, should be investigated as well. Therefore, the present study aims to investigate OTC accumulation using horsebean in soils after removing different extractable fractions during an exposure experiment and to characterise the effect of horsebean on the extractability of OTC by a single-chemical extraction in soils during exposure. The objective was to exactly reveal the bioavailability of different OTC bound fractions remaining in soil and assess its ecological risk.
2. Materials and methods
2.1 Oxytetracycline (OTC) and other chemicals
Oxytetracycline hydrochloride (95% purity, analytical grade) was purchased from the Sigma Co. St. Louis, USA. The standard reference material of tetracycline hydrochloride was obtained from the Institute of Veterinary Drug Control in Beijing, China. Both were stored at 4 °C. Some important physicochemical properties of OTC are molecular weight (MW) (496.89 g mol−1), molecular formula (C22H24N2O9·HCl), and aqueous solubility (500 mg mL−1). The structure of OTC is shown in Fig. 1; OTC has three acid dissociation constants (pKa = 3.27, 7.32, and 9.11).
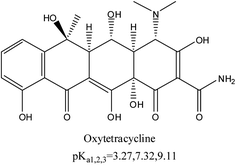 |
| Fig. 1 Molecular structure and molecular formula of oxytetracycline. | |
All other chemicals were of analytical grade. Deionized water was used for all the experiments.
2.2 Soil properties and treatments
Surface soil (0–20 cm) was manually collected from cinnamon soil of agricultural fields in Weifang, Shandong, China. The soil was air-dried and passed through a 1.00 mm sieve. Adding dairy manure in the ratio of 1
:
5 increased the organic matter content of soil to ensure plant growth with enough nutrients during exposure for 28 days. The analysis showed that the properties of mixed soil were as follows: organic matter, 8.64%; total nitrogen, 0.37%; total phosphorus, 0.34%; total potassium, 2.67%; alkali-hydrolyzable nitrogen, 110 mg kg−1; available phosphorus, 228 mg kg−1; available potassium, 2727 mg kg−1; and pH, 7.4. OTC was not detected in soil or dairy manure.
We wanted to compare the potential bioaccumulation and bioavailability of OTC in horsebeans from different OTC bound fractions remaining in soil. Briefly, the abovementioned mixed soil was freshly spiked with OTC. After contaminating for 24 h, the soils were then extracted with water (T1 treatment), 0.1 M CaCl2 (T2 treatment) and 0.1 mol L−1 Na2EDTA-McIlvaine (pH = 4.0 ± 0.05) (T3 treatment) for collecting the water dissolved fraction, a part of the loosely bound fractions, and all extractable fractions of OTC, respectively. The schematic of the OTC removal of three fractions is shown in Fig. 2. T1 was considered as the remaining OTC of all loosely bound and resistant bound fractions. T2 was considered as the remaining part of loosely bound and resistant bound fractions because Ca2+ can be exchanged with a part of adsorbed OTC on the soil. T3 was considered as the remaining resistant bound (non-extractable residues) fractions. In T3, the treated soils were washed with de-ionized water to avoid the effect of extracting agent on the growth of horsebean because crystalline substance was precipitated on the soil surface after EDTA extraction in our preliminary experiment. For non-soluble contaminants, water extraction is less effective than extraction with neutralizing solutions or chelating agents. OTC was not determined in all the eluates, which showed that washing did not remove OTC. Finally, adding clean soil in the ratio of 7
:
1 increased the volumes of these extracted soils.29 The control was not treated soil, that is to say, the soil was not extracted before exposure.
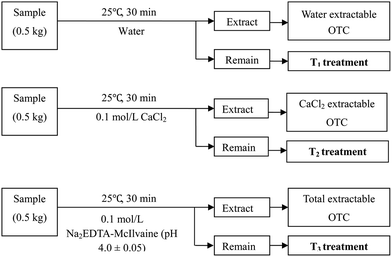 |
| Fig. 2 The schematic of the OTC removal of three fractions. | |
2.3 Exposure experiment
Horsebean (Pisum sativum Linn) seed was obtained from Tianjin Academy of Agriculture Science. Seeds were surface sterilized with 10% H2O2 for 10 min, rinsed with distilled water and soaked in deionised water for 2–3 h before planting. Three seeds of uniform size per each replicate were used to reduce the variability between horsebeans. Horsebeans were placed in a PVC pot (10 cm in diameter, 15 cm in height) containing 500 g of soil. Every treatment involved twenty-four PVC pots that contained twelve pots with horsebean and twelve pots without horsebean. The soils were initially adjusted to approximately 60% water holding capacity, and then deionised water was added when required to replace losses during the exposure experiment and to ensure initial field conditions. Pots were placed in a greenhouse to grow at 25 ± 1.0 °C for 28 days. Pots with horsebeans were investigated at 10, 14, 21 and 28 days after exposure, with triplicate analyses being carried out to determine OTC accumulation by root and shoot. We investigated the horsebean sample from the beginning of the 10 days of exposure because horsebean was still a seed without seedling prior to 10 days. Moreover, soil samples with and without plants were analysed by single-chemical extraction at 10, 14, 21 and 28 days of exposure.
2.4 Horsebean-extraction and determination of OTC
The concentrations of OTC in plant samples were determined by adding 25 mL of 0.1 mol L−1 Na2EDTA-McIlvaine and following the method described by Li et al. (2011).14 The extracts were filtered through 0.22 μm polytetrafluoroethylene membrane and then concentrated and cleaned-up by solid phase extraction (SPE) cartridges (Oasis HLB, 6 cm3/500 mg). The concentration of OTC in the extraction solution was measured by a reverse-phase HPLC (Waters Corp.) with a 46 × 255 mm2 Waters ODS-C18 (5 μm) column, followed by UV detection at 360 nm. The mobile phase was a mixture of 0.01 M oxalic acid–acetonitrile (80
:
20, v/v) in an equilibrium system, supplied at a flow rate of 1.0 mL min−1. The retention time was 5.24 min.
2.5 Single-chemical extraction in soil after exposure experiment
As discussed in Section 2.2, three solutions (water, 0.1 mol L−1 CaCl2 and 0.1 mol L−1 Na2EDTA-McIlvaine (pH 4.0 ± 0.05)) were used in geochemical extraction schemes to determine the water dissolved fraction and two bound fractions with different binding potentials to estimate the effect of horsebean on potential bioavailability of OTC in soil. Briefly, soil samples were first freeze dried. 20 mL of extractant was added to 1.000 g dried soil and this mixture was shaken for 30 min at room temperature (25 °C). The extract was separated from the solid residue by centrifugation (3000 rpm) and then filtered through 0.22 μm polytetrafluoroethylene membrane. OTC concentration was determined by the procedure discussed in Section 2.4.
3. Results and discussion
3.1 The extractability of OTC in soils after removing extractable fractions
According to contamination and extraction treatment in soils before the exposure experiment, initial total OTC concentration was 200 ± 0.00, 196 ± 0.23, 153 ± 1.35, and 86.1 ± 2.12 mg kg−1 in the control, T1, T2, and T3, respectively. It is well known that all the contaminant fractions remaining in the soil are not completely available to organisms because a part is progressively sequestrated by the organic and inorganic constituents of the soil.30,31 Results showed that the water-extractable fraction was 4.24 ± 0.23 mg kg−1 in the control, and was not detected in the three treated soils (T1, T2, and T3). Moreover, CaCl2-extractable fractions were 43.3 ± 1.15 and 47.5 ± 1.35 mg kg−1 in T1 and control, respectively, but CaCl2-extractable fractions were not detected in T2 and T3. Total-extractable fractions were 66.4 ± 0.77, 110 ± 1.97 and 114 ± 2.12 mg kg−1 in T2, T1 and control, respectively, and not detected in T3. The abovementioned extraction results showed that OTC can exist as a resistant bound fraction with a concentration as high as 86.1 ± 2.12 mg kg−1 in soils because the fraction was not extracted even by the exhaustive extractant. According to pretreatment, initial various extractable and total concentrations were present in the following order: the control > T1 > T2 > T3.
3.2 Horsebean growth during exposure experiment
Horsebean growth was observed by measuring the dry weights of roots and shoots. As shown in Fig. 3a, the dry weight of root decreased gradually over time in all the soils. At 10 days of exposure, the root weight included root and seed because they cannot be seperated as a whole, while at 14 days of exposure, the seed disappeared and only root existed, which might have decreased the dry weight of root during 10–14 days. It was worth considering that the abovementioned decrease trend was not accurate. During the 14 to 21 days of exposure, the dry weight of root did not change significantly. From 21 to 28 days of exposure, the dry weight of root decreased. Root grew in the soil; thus, total-, CaCl2-, and water-extractable OTC (as shown in Fig. 5) in soil will directly affect root growth during 10–28 days of exposure. According to the results, there may be no relationship between influence on the root growth and extractable OTC concentration. Moreover, the negative influence is related with exposure time because the dry weight of root decreased significantly during 21–28 days and not during 14–21 days. As shown in Fig. 3b, the dry weight of shoots increased from 10 to 21 days of exposure in all the soils with the growth of the seedlings, and decreased from 21 to 28 days of exposure. The negative influence may be due to the high concentrations of OTC in shoots at 28 days of exposure, as seen in the results obtained from Fig. 4. The low accumulation of OTC in shoot with a value lower than 1.07 ± 0.09 mg increased the dry weight of the shoot of the plants during 10–21 days. The highest accumulation level of OTC with a value higher than 1.21 ± 0.06 mg had the worst effects on shoot growth at 28 days of exposure, which is similar to the results obtained in a previous study,32 because the antibiotic inhibits photosynthetic activity.33
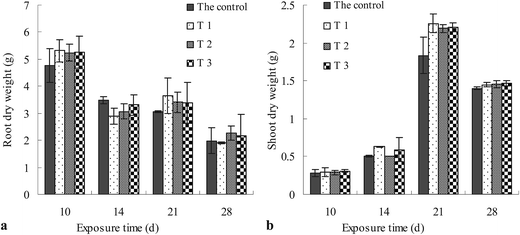 |
| Fig. 3 Root (a) and shoot (b) dry weights of horsebean from soils after removing different extractable fractions during 28 days exposure experiment. The bars represent ±SE of the mean. | |
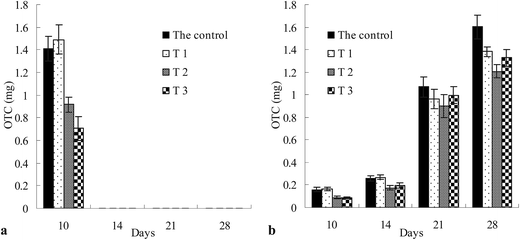 |
| Fig. 4 OTC accumulation by horsebean from soils after removing different extractable fractions during 28 days exposure experiment. Data are expressed as root accumulation concentration (a) and shoot accumulation concentration (b). The bars represent ±SE of the mean. | |
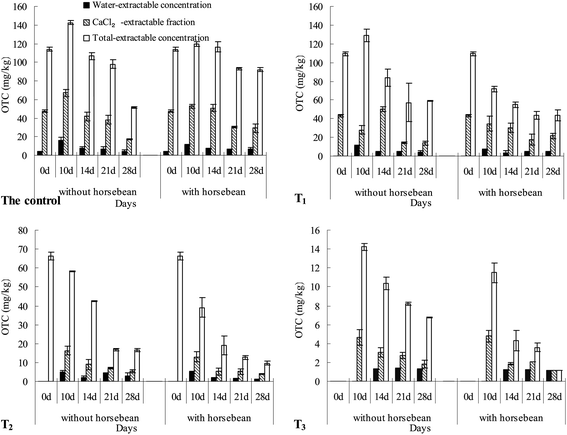 |
| Fig. 5 Effects of horsebean on OTC extractability from soils after removing different extractable fractions during 28 days exposure experiment. | |
There was an insignificant difference (p > 0.05) between root and shoot weight among the control, T1, T2, and T3 at same exposure times, which indicated that the soil treatment process had no effect on the growth of horsebean.
3.3 OTC accumulation by horsebeans during exposure experiment
The accumulation of OTC in root and shoot over time is shown in Fig. 4. In all the soils, OTC accumulation in root (Fig. 4a) was detected only at 10 days of exposure, and after that accumulation less than the quantification limits (<0.001 mg kg−1) were detected. At 10 days of exposure, the accumulation of OTC in root was in the order T1 > control > T2 > T3, which was a different order compared to initial various extractable fractions, and the order of the total concentration of OTC in soils was control > T1 > T2 > T3. There was an insignificant difference (p > 0.05) between the control and T1, and there was a significant difference (p < 0.01) between T1, T2, and T3.
In control, T1, T2 and T3, OTC accumulation in shoot (Fig. 4b) slightly increased during 10–14 days of exposure, which then rapidly increased during 14–28 days. At 10 and 14 days of exposure, OTC accumulation in shoot for control and T1 was significantly (p < 0.05) higher than that for T2 and T3. Moreover, there was an insignificant difference (p > 0.05) between control and T1 or between T2 and T3. At 21 days of exposure, there was an insignificant difference (p > 0.05) between the four soils. At 28 days of exposure, OTC shoot accumulation in the control was significantly (p < 0.05) higher than that in T1, T2 and T3. In a word, the highest OTC accumulation concentration was always found in the control or T1 at the same exposure time, which could be caused by higher initial various extractable fractions or total OTC concentration in control and T1. However, the order of OTC concentration in shoot in three differently treated soils at same exposure time was not consistent with that of initial various extractable and total concentrations in three differently treated soils.
At 10 days of exposure, OTC was detected in both root and shoot, and the accumulation in root, from 0.707 ± 0.104 to 1.491 ± 0.129 mg, was higher than in shoot, from 0.088 ± 0.012 to 0.164 ± 0.014 mg. After that, OTC concentration in root was lower than the quantification limit, and high OTC concentrations, from 0.176 ± 0.016 to 1.602 ± 0.107 mg, were detected in shoot, which showed that OTC translocation from root to shoot was stronger after 10 days than at 10 days.
It has been found that initial total OTC concentrations were different in the three differently treated soils and control. For better presentation and for direct comparison of treated soils, root concentration factors (RCF) and shoot concentration factors (SCF) for OTC are displayed in Table 1. Accumulation factors were given in mg of OTC over mg of initially measured OTC in soil. RCF values were from 0.012 ± 0.002 to 0.016 ± 0.003 at 10 days of exposure, and there was an insignificant difference (p > 0.05) between the four soils. In all the four soils, SCF slightly increased from 10 to 14 days of exposure, which then rapidly increased during 14–28 days of exposure. At 10 days of exposure, there was an insignificant difference (p > 0.05) between the control, T1, T2 and T3. At 14–28 days of exposure, the highest SCF was observed in T3, and there were insignificant differences (p > 0.05) between the control, T1 and T2. In particular, in T3 at 28 days of exposure, SCF was as high as 0.031. Results showed that resistant bound OTC remaining in T3 was more readily accumulated in horsebean shoot.
Table 1 Root concentration factors (RCF) and shoot concentration factors (SCF) for OTC in soila
Soil treatments |
Root/shoot |
Days |
10 |
14 |
21 |
28 |
Accumulation factors are given in mg of OTC over mg of initial OTC measured in soil. |
The control |
Root |
0.014 ± 0.005 |
0 |
0 |
0 |
Shoot |
0.002 ± 0.001 |
0.003 ± 0.001 |
0.011 ± 0.004 |
0.016 ± 0.002 |
T1 |
Root |
0.015 ± 0.003 |
0 |
0 |
0 |
Shoot |
0.002 ± 0.0004 |
0.003 ± 0.001 |
0.010 ± 0.003 |
0.014 ± 0.001 |
T2 |
Root |
0.012 ± 0.002 |
0 |
0 |
0 |
Shoot |
0.001 ± 0.0007 |
0.002 ± 0.001 |
0.012 ± 0.003 |
0.016 ± 0.004 |
T3 |
Root |
0.016 ± 0.003 |
0 |
0 |
0 |
Shoot |
0.002 ± 0.001 |
0.005 ± 0.002 |
0.023 ± 0.006 |
0.031 ± 0.008 |
In the present study, the pretreatment caused only different OTC fractions to remain in the soils; however, other soil properties in the control and the three differently treated soils were not affected by the pretreatment. Consequently, we speculated that the variability in OTC accumulation by horsebean might be explained mainly by the differences in remaining OTC fractions in soils. Heise et al.34 suggested that investigations on the phenomenon of non-extractability under different laboratory and field conditions are necessary to complete the data pool for a respective environmental risk assessment because the nature of non-extractable antibiotic residues in soil is unknown to date. In this study, it is worth noting that non-extractable (resistant bound) OTC residue in soil has still higher bioaccumulation factors (SCF values) by horsebean, although it was not extracted by 0.1 mol L−1 Na2EDTA-McIlvaine, which was used as an exhaustive extractant compared to the other extractant and the control. It has been reported35 that easily extractable antibiotic fraction are accelerated near roots throughout the plant growth period because of the function of the root exudates and microbial communities in the rhizosphere. However, as shown in Fig. 5, for T3, water-, CaCl2-, total-extractable concentrations in soils with horsebean are not higher than that without horsebean. This phenomenon may occur because non-extractable OTC residues first get transformed easily into extractable OTC in the rhizosphere, and then get absorbed quickly by the plant root, which explains why the three extractable OTC concentrations were not high in soils with horsebean in our study. Thus, the high concentration of OTC accumulation in shoot was also found for T3 as compared to the other treated soils (Fig. 4b). Moreover, initial total OTC concentration in T3 soil was lower than other treated soils (as shown in 3.1), which was because SCF values as the ratio (OTC mg in shoot)/(OTC mg in soil) in T3 were always higher than other treated soils. Consequently, the abovementioned tests confirmed the low affinity of the non-extractable OTC residues towards the soil matrix for bioavailability in horsebean. It is worth noting that the resistant bound fraction of OTC in soil could still be related to its high ecological risk for the plant, which indicated that OTC was transferred through the food chain to crops or other foods of human beings, and it finally caused risk to human health.
3.4 Effects of horsebean on OTC extractability from soil during exposure experiment
To investigate the effect of horsebean on bioavailable OTC concentrations in soil after removing various extractable fractions over the exposure experiment, three fractions were extracted by water, 0.1 mol L−1 CaCl2, and 0.1 mol L−1 Na2EDTA-McIlvaine as water-extractable fraction, CaCl2-extractable fraction, and total-extractable fraction, respectively. It is well known that the water-extractable fraction has the highest bioavailability among all the extractable fractions. The second is CaCl2-extractable fraction because the total concentration of Ca2+ is readily exchangeable and water soluble fraction. Following is the total-extractable fraction, which is the sum of all the extractable fractions. As shown in Fig. 5, the three extractable concentrations increased during 0–10 days and then decreased after that in the control, irrespective of the presence of horsebean. Adding horsebean decreased three extractable concentrations during 0–10 days and 14–21 days, and increased their concentrations during 10–14 days and during 21–28 days of exposure. There were significant effects of horsebean on CaCl2- and total-extractable concentrations (p < 0.05), but not on water-extractable fraction. In T1, water-extractable concentration was 0 mg kg−1 at 0 day, and the concentration increased during 0–10 days and then decreased after that, irrespective of the presence of horsebean. For soils without horsebean, CaCl2-extractable concentration decreased during 0–10 days, and increased during 10–14 days, and again decreased after that. Total-extractable concentration increased first during 0–10 days, and then decreased. For soils with horsebean, CaCl2- and total-extractable concentrations decreased during the entire exposure. Adding horsebean decreased water- and total-extractable concentrations during the entire exposure experiment. The effect on the total-extractable fraction was at a significant level (p < 0.05) during exposure experiment, and the effect on the water-extractable fraction was significant (p < 0.05) at 10 and 21 days of exposure. Adding horsebean increased (p < 0.05) CaCl2-extractable concentrations at 10, 21, and 28 days of exposure, but decreased (p < 0.01) CaCl2-extractable concentrations at 14 days of exposure. In T2, initial water- and CaCl2-extractable concentration was 0 mg kg−1. Irrespective of the presence of horsebean, their concentrations increased rapidly during 0–10 days, and then decreased during 10–28 days. Total-extractable concentration decreased gradually during the entire exposure experiment, irrespective of the presence of horsebean. Horsebean activity decreased water-, CaCl2- and total-extractable concentrations during the entire exposure period with a significant difference (p < 0.05), except for water-extractable fraction at 10 and 14 days with insignificant difference (p > 0.05). For T3, initial water-, CaCl2- and total-extractable concentrations were 0 mg kg−1. Water-extractable concentrations were still 0 mg kg−1 at 10 days of exposure, which increased rapidly during 10–14 days, and then decreased during 14–28 days, irrespective of the presence of horsebean. CaCl2- and total-extractable concentrations increased rapidly during 0–10 days and then decreased during 10–28 days of exposure, irrespective of the presence of horsebean. Horsebean activity significantly decreased (p < 0.05) the CaCl2- (during 14–28 days) and total-extractable (during 10–28 days) concentrations, but did not affect water-extractable concentrations.
In the end of exposure experiment, adding horsebean significantly increased (p < 0.05) the total-extractable concentrations with a value of 40.6 mg kg−1 in the control, but significantly decreased it (p < 0.05) in T1, T2 and T3. The reason was as follows. In all the soils without plants, water- and CaCl2-extractable fractions were released from resistant bound fraction under the experimental condition. Then, horsebean activity further promoted the release of resistant bound OTC in soils. In the control, initial three extractable OTC concentrations were high, and then experiment condition and horsebean increased their concentrations by root exudate, and thus during exposure, the concentrations of the three extractable were increased, although horsebean absorbed a part of extractable fractions compared with the three differently treated soils. T1, T2 and T3 contained small concentrations of initial extractable OTC due to the pretreatment. Although the experimental condition and horsebean increased the concentration of extractable OTC according to the results obtained for the control, horsebean itself absorbed and utilize the released extractable OTC with high accumulation ratio, as shown in Section 3.3, which resulted in a decrease in the concentration of total or various extractables in soils treated with horsebean at the end of the exposure experiment compared to those without horsebean.
Regardless of the presence of horsebean, the concentrations of the three extractables at the same exposure time were always in the following order: control or T1 > T2 > T3, and this order was approximately consistent with the initial three extractable and total concentrations. During the entire exposure experiment, total-extractable concentration ranges were 51.6 (±0.91)–143 (±2.23), 43.4 (±5.85)–129 (±6.65), 9.66 (±0.98)–58.5 (±0.19), and 1.15 (±0.00)–14.2 (±0.38) mg kg−1 in control, T1, T2, and T3, respectively. CaCl2-extractable concentration ranges were 17.6 (±0.37)–67.3 (±3.79), 13.9 (±2.32)–50.3 (±2.74), 3.99 (±0.13)–16.3 (±2.30), and 1.15 (±0.00)–4.77 (±0.66) mg kg−1 in control, T1, T2, and T3, respectively. Water-extractable concentration ranges were 4.33 (±1.69)–15.7 (±4.03), 3.67 (±2.12)–11.1 (±0.64), 1.13 (±0.01)–5.07 (±0.93), and 1.15 (±0.00)–1.29 (±0.01) mg kg−1 in control, T1, T2, and T3, respectively.
In this study, adding horsebean did not affect the change trend of the three extractable fractions in control, T2, and T3 during exposure. Regardless of the presence of horsebean, water-extractable concentration first increased and then decreased in control, T2, and T3. CaCl2- and total-extractable concentrations first increased and then decreased in control. CaCl2- and total-extractable concentrations were gradually decreased during exposure in T2 and T3. However, adding horsebean affected the change trend of CaCl2- and total-extractable fractions in T1, whereas did not affect the change trend of water-extractable fraction. In T1, CaCl2-extractable concentration decreased during 0–10 days, increased during 10–14 days, and then decreased during 14–28 days without horsebean. CaCl2-extractable concentration decreased gradually during the entire exposure experiment with horsebean.
3.5 Relationship between extractability concentration in soil and accumulation by horsebean
Correlation analysis showed that there was an insignificant correlation (p > 0.05) between OTC concentration in horsebean shoot (or whole plant) and various extractable OTC in soil during exposure in control, T1, T2 and T3. It is well known that root provides the passage for OTC from soil to plant; thus, the close relationship between the accumulation in horsebean root and different extractable OTC at 10 days of exposure in soils suggests the bioavailability of different extractable OTC fractions in soils. As shown in Fig. 6, significant positive correlation was observed between the concentration of OTC in root and water-extractable fraction (r = 0.914*; p < 0.05), CaCl2-extractable fraction (r = 0.963**; p < 0.01) and total-extractable fraction (r = 0.937*; p < 0.05), which showed that there were close relationships between water-, CaCl2-, total-extractable fractions in soil and root accumulation in horsebean.
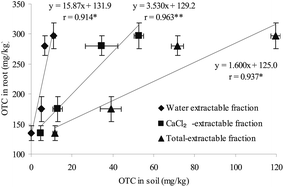 |
| Fig. 6 The correlation analysis between different extractable OTC concentrations and OTC accumulations by root at 10 days of exposure. *p < 0.05; **p < 0.01. | |
4. Conclusion
This study provides, for the first time, evidence for OTC accumulation by horsebean from soils after removing various labile extractable fractions by pretreatment. Findings of this study clearly demonstrate that the resistant bound fraction of OTC in soil after removing all extractable fractions with exhaustive extraction using 0.1 mol L−1 Na2EDTA-McIlvaine always exhibits the highest bioaccumulation factors in horsebean compared with when fewer extractable fractions are removed during 14–28 days of exposure. Most of the previous studies have been focused on assessing total OTC contamination concentration in soil using 0.1 mol L−1 Na2EDTA-McIlvaine extraction, and little attention was paid to the biological availability of OTC remaining in the soil as resistant bound fraction. Findings of this study indicated that it was not accurate that resistant bound OTC had such a low availability as we expected, and the ecological risk of resistant bound OTC in soil has been underestimated in previous investigations. OTC was accumulated in shoot and root during exposure; however, after exposure for 10 days, the amount of OTC in the root was less than its quantification limit. Adding horsebean did not affect the change trend of various extractable fractions in the control, T2, and T3 during the exposure. However, adding horsebean affected the change trend of CaCl2- and total-extractable fractions in T1, but did not affect the change trend of water-extractable fraction. Compared to the case when horsebean was not added, horsebean activity increased the total-extractable concentration in the control, but decreased the total-extractable concentration in T1, T2 and T3. The correlation analysis showed that there were close relationships between water-, CaCl2-, total-extractable fractions in soil and root accumulation in the horsebean. An important future research direction might be to derive the release and bioavailability of resistant bound OTC in soil. Such study would greatly facilitate risk assessment and ecological benchmark calculations for soil OTC contamination.
Acknowledgements
This work was financially supported by the National Natural Science Foundation of China as a key project (grant no. 21037002) and by the Tianjin Municipal Science and Technology Commission as a youth project (grant no. 13JCQNJC09000) and a key project (grant no. 13JCZDJC35900).
References
- P. Kulshrestha, R. F. Giese and D. S. Aga, Environ. Sci. Technol., 2004, 38, 4097–4105 CrossRef CAS.
- B. Halling-Sørensen, Chemosphere, 2000, 40, 731–739 CrossRef.
- Y. Y. Bao, Q. X. Zhou, L. Z. Guan and Y. Y. Wang, Waste Manage., 2009, 29(4), 1416–1423 CrossRef CAS PubMed.
- X. Hu, Q. Zhou and Y. Luo, Environ. Pollut., 2010, 158, 2992–2998 CrossRef CAS PubMed.
- K. L. D. Henderson and J. R. Coats, Veterinary Pharmaceuticals in the Environment, ACS Symposium Series, ch. 2, 2010, pp. 3–7 Search PubMed.
- M. Kahle and C. Stamm, Environ. Sci. Technol., 2007, 41(1), 132–138 CrossRef CAS.
- S. Saha, M. K. Mandal, L. C. Chen, S. Ninomiya, Y. Shida and K. Hiraoka, J. Mass Spectrom., 2013, 2, S0008 Search PubMed.
- B. A. Smith, B. Greenberg and G. L. Stephenson, Chemosphere, 2010, 81, 755–766 CrossRef CAS PubMed.
- P. H. F. Hobbelen, J. E. Koolhaas and C. A. M. Van Gestel, Environ. Pollut., 2006, 144, 639–646 CrossRef CAS PubMed.
- D. R. Ownby, K. A. Galvan and M. J. Lydy, Environ. Pollut., 2005, 136, 315–321 CrossRef CAS PubMed.
- K. Lock and C. R. Janssen, Environ. Toxicol. Chem., 2003, 22, 1162–1166 CrossRef CAS.
- O. Schramel, B. Michalke and A. Kettrup, Sci. Total Environ., 2000, 263, 11–22 CrossRef CAS.
- Q. Yu, Y. Y. Bao, Y. M. Li, Q. X. Zhou and Y. X. Liu, China Environ. Sci., 2011, 31(6), 951–957 CAS.
- Y. W. Li, X. L. Wu, C. H. Mo, Y. P. Tai, X. P. Huang and L. Xiang, J. Agric. Food Chem., 2011, 59, 7268–7276 CrossRef CAS PubMed.
- W. D. Kong, C. G. Li, J. M. Dolhi, S. Y. Li, J. Z. He and M. Qiao, Chemosphere, 2012, 87(5), 542–548 CrossRef CAS PubMed.
- N. S. Simon, Environ. Sci. Technol., 2005, 39, 3480–3487 CrossRef CAS.
- A. B. A. Boxall, P. Johnson, E. J. Smith, C. J. Sinclair, E. Stutt and L. S. Levy, J. Agric. Food Chem., 2006, 54(6), 2288–2297 CrossRef CAS PubMed.
- D. W. Hawker, R. Cropp and M. Boonsaner, J. Hazard. Mater., 2013, 263, 458–466 CrossRef CAS PubMed.
- D. H. Kang, S. Gupta, C. Rosen, V. Fritz, A. Singh, Y. Chander, H. Murray and C. Rohwer, J. Agric. Food Chem., 2013, 61, 9992–10001 CrossRef CAS PubMed.
- L. J. Carter, C. D. Garman, J. Ryan, A. Dowle, E. Bergström, J. Thomas-Oates and A. B. A. Boxall, Environ. Sci. Technol., 2014, 48(10), 5955–5963 CrossRef CAS PubMed.
- M. Boonsanera and D. W. Hawker, Sci. Total Environ., 2010, 408, 1731–1737 CrossRef PubMed.
- M. Boonsanera and D. W. Hawker, Environ. Toxicol. Chem., 2012, 78, 142–147 Search PubMed.
- M. Boonsanera and D. W. Hawker, Chemosphere, 2013, 93, 1009–1014 CrossRef PubMed.
- N. P. Gujarathi, B. J. Haney, H. J. Park, S. R. Wickramasinghe and J. C. Linden, Biotechnol. Prog., 2005, 21, 775–780 CrossRef CAS PubMed.
- R. J. Fussell, M. G. Lopez, D. N. Mortimer, S. Wright, M. Sehnalova, C. J. Sinclair, A. Fernandes and M. Sharman, J. Agric. Food Chem., 2014, 62, 3651–3659 CrossRef CAS PubMed.
- W. D. Kong, Y. G. Zhua, Y. C. Liang, J. Zhang, F. A. Smith and M. Yang, Environ. Pollut., 2007, 147, 187–193 CrossRef CAS PubMed.
- F. J. Peng, L. J. Zhou, G. G. Ying, Y. S. Liu and J. L. Zhao, Environ. Toxicol. Chem., 2014, 33(4), 776–783 CrossRef CAS PubMed.
- F. J. Peng, G. G. Ying, Y. S. Liu, H. C. Su and L. Y. He, Sci. Total Environ., 2015, 506–507, 58–65 CrossRef CAS PubMed.
- B. Gevao, C. Mordaunt, K. T. Semple, T. G. Piearce and K. C. Jones, Environ. Sci. Technol., 2001, 35(3), 501–507 CrossRef CAS.
- Y. Wang, L. Wang, F. Li, J. Liang, Y. Li, J. Dai, T. Loh and Y. Ho, J. Agric. Food Chem., 2009, 57(13), 5878–5883 CrossRef CAS PubMed.
- A. J. Carrasquillo, G. L. Bruland, A. A. MacKay and D. Vasudevan, Environ. Sci. Technol., 2008, 42(20), 7634–7642 CrossRef CAS.
- M. B. M. Ahmed, A. U. Rajapaksha, J. E. Lim, N. T. Vu, S. Kim, H. M. Kang, S. S. Lee and Y. S. Ok, J. Agric. Food Chem., 2015, 63, 398–405 CrossRef CAS PubMed.
- G. D. Marc, A. Gismondi, L. Canuti, M. Scimeca, A. Volpe and A. Canini, Plant Biol., 2014, 16(4), 792–800 CrossRef PubMed.
- J. Heise, S. Höltge, S. Schrader and R. Kreuzig, Chemosphere, 2006, 65, 2352–2357 CrossRef CAS PubMed.
- I. Rosendahl, J. Siemens, J. Groeneweg, E. Linzbach, V. Laabs, C. Herrmann, H. Vereecken and W. Amelung, Environ. Sci. Technol., 2011, 45(12), 5216–5222 CrossRef CAS PubMed.
|
This journal is © The Royal Society of Chemistry 2015 |