DOI:
10.1039/C4RA13914C
(Paper)
RSC Adv., 2015,
5, 15494-15499
Analysis of pathogenic bacteria using exogenous volatile organic compound metabolites and optical sensor detection†
Received
5th November 2014
, Accepted 23rd January 2015
First published on 23rd January 2015
Abstract
A novel, low-cost and simple method for the detection of pathogenic bacteria is proposed. The approach is based on the generation of an exogenous volatile organic compound (VOC) produced by the addition of an enzyme substrate to the bacterial sample. The generated VOC is then trapped in agarose gel allowing colour development to take place; visual detection is then possible by both the naked eye and by colorimetric analysis. Agarose gel has been evaluated as both a suitable VOC trapping matrix and host for the colour-generating reagents. This proof of concept method allowed for the discrimination between β-glucosidase and β-alanyl aminopeptidase producing bacteria. Enterococcus faecium and Klebsiella pneumoniae are both β-glucosidase producers and generated a yellow colour within agarose gels upon enzymatic hydrolysis of 2-nitrophenyl-β-D-glucoside. Pseudomonas aeruginosa is a known β-alanyl aminopeptidase producer and was shown to hydrolyse the trifluoroacetic acid (TFA) salt of 3-amino-N-phenylpropanamide resulting in the development of an orange colour within agarose gels spiked with the sodium salt of 1,2-naphthoquinone-4-sulfonic acid. 3-Amino-N-phenylpropanamide (as its TFA salt) and 2-nitrophenyl-β-D-glucoside concentrations of 20 μg mL−1 (or 72 μmol L−1) and 100 μg mL−1 (or 332 μmol L−1), respectively were the minimum quantities required for colour production following 18 h of incubation. The use of 3-amino-N-phenylpropanamide, TFA salt indicated that synthesised enzyme substrates can be tailor-made to liberate exogenous VOCs for colour generation.
1. Introduction
The detection of pathogenic bacteria in clinical and food samples can often be a time-consuming and laborious process. The rapid identification of bacteria is essential for effective patient treatment in clinical settings and for determining the source of contamination in food samples. Bacteria have been shown to liberate a wide range of volatile organic compounds (VOCs).1,2 Several analytical methods which have focussed on the detection of VOCs liberated by bacteria have been developed. Detection of bacterial VOCs using headspace solid phase microextraction (SPME) coupled with gas chromatography-mass spectrometry (GC-MS) has shown potential as a diagnostic tool for bacterial detection.3 Other analytical methods used for bacterial VOC analysis include multi-capillary column-ion mobility spectrometry4 and secondary electrospray ionization-mass spectrometry.5 However, clinical and food laboratories are unlikely to possess such instruments for VOC analysis; in addition, they are expensive to purchase, require trained laboratory staff to operate them and regular maintenance. Alternative VOC detection methods that do not require instrumentation would be highly desirable. Visual VOC detection methods have recently been developed. For example, a disposable colorimetric sensing array to identify 10 strains of bacteria has been developed.6 Dyes incorporated into the sensor changed colour upon exposure to VOCs generated by bacteria. The use of enzyme substrates as a potential tool for bacterial detection, via exogenous VOC evolution, has previously been described.7E. coli was detected via the generation of the exogenous 2-nitrophenol using the enzyme substrate 2-nitrophenyl-β-D-galactoside; ion mobility spectrometry was used for VOC detection. Subsequently xerogels have been used to trap and colorimetrically detect VOCs, specifically 4-nitrophenol released by enzymatic hydrolysis of 4-nitrophenyl-β-D-glucuronide8 and p-dimethylaminocinnamaldehyde for the detection of E. coli (a known indole producer).9 In the case of the latter example, p-dimethylaminocinnamaldehyde reacted with indole to generate a green colour within the xerogel.
Agarose is a polysaccharide with repeating units of D-galactose and 3,6-anhydro-L-galactose and upon gelation it forms a porous semi-solid matrix. Agarose gels are commonly used for separating molecules during electrophoresis; however, here it is used as a potential matrix for VOC trapping. Agarose gel is inexpensive and its preparation is simple. In addition, it is possible to modify the agarose gel by the addition of specific and selective colorimetric reagents that will generate colour in the presence of target compounds. The aim of this paper is to develop a novel, simple optical method for bacterial identification via the detection of exogenous VOCs. Following bacterial enzyme activity, the exogenous VOCs are trapped in modified agarose gel and optically detected by either the naked eye or colorimetric analysis; the latter allows quantification of the exogenous VOC. The proof of concept is demonstrated using two enzyme substrates: the commercially available 2-nitrophenyl-β-D-glucoside which liberates 2-nitrophenol in the presence of bacteria with β-glucosidase activity and a synthesised substrate, 3-amino-N-phenylpropanamide, TFA salt which liberates aniline in the presence of bacteria with β-alanine aminopeptidase activity. The 2-nitrophenol liberated from 2-nitrophenyl-β-D-glucoside is yellow coloured in alkaline conditions whereas the aniline released from a substrate targeting β-alanine aminopeptidase activity can be optically detected by its reaction with 1,2-naphthoquinone-4-sulfonic acid, sodium salt (NQS) giving a red colour. NQS has been used for the colorimetric detection of amines in solution10 and in the vapour phase.11
β-Glucosidase substrates are widely used in chromogenic media; β-glucosidase activity has been demonstrated in several species, including the Gram-positive organism Enterococcus faecium and the Gram-negative species Klebsiella pneumoniae.12Pseudomonas aeruginosa is a known β-alanine aminopeptidase producer and enzyme substrates targeting β-alanine aminopeptidase activity have been synthesised and successfully used in chromogenic media for the detection of Ps. aeruginosa.12,13 Pathogenic bacteria known to produce β-glucosidase and β-alanine aminopeptidase activity were selected to demonstrate this proof of concept, illustrating the use of exogenous VOC detection, via modified agarose gels, as a novel method of bacterial identification based on optical sensor detection. This novel approach for identifying or detecting bacteria, via the generation of exogenous VOCs, allows for label-free, non-invasive and continuous monitoring of a sample.
2. Experimental
2.1 Chemicals/reagents
2-Nitrophenol (98%), aniline (≥99%), N-methyl-2-pyrrolidinone (99%), tboc-β-alanine-OH (≥99.0%), N-hydroxysuccinimide (98%) and trifluoroacetic acid (99%) were purchased from Sigma Aldrich (Poole, UK). 2-Nitrophenyl-β-D-glucoside was obtained from Apollo Scientific (Stockport, UK). Dicyclohexylcarbodiimide (99%) and 1,2-naphthoquinone-4-sulfonic acid sodium salt (NQS) (97%) were purchased from Alfa Aesar (Morecambe, UK). Sodium hydroxide (98.0%) was obtained from BDH Laboratory Supplies (Lutterworth, UK) and agarose (molecular biology grade) from Melford Laboratories Ltd. (Ipswich, UK). All solvents (dichloromethane, hexane and ethyl acetate) were of analytical reagent grade and purchased from Fisher Scientific (Loughborough, UK). Brain heart infusion (BHI) broth and agar were purchased from Oxoid (Basingstoke, UK).
2.2 Microbiology
Gram-negative and Gram-positive bacteria were obtained from the National Collection of Type Cultures (NCTC), Colindale, UK. Gram-negative bacteria: Escherichia coli NCTC 10418, Klebsiella pneumoniae NCTC 9528, Pseudomonas aeruginosa NCTC 10662 and Pseudomonas fluorescens NCTC 10688. Gram-positive bacteria: Enterococcus faecium NCTC 7171 and Streptococcus agalactiae NCTC 8181. Bacteria were stored and sub-cultured weekly on BHI agar.
2.3 Gel preparation for the detection of 2-nitrophenol
Agarose gel (1%) was prepared by dissolving 0.5 g agarose in distilled water and microwaving until boiling (approximately 1 min). The solution was allowed to cool and then modified by addition of 0.2 mol L−1 NaOH; the final total volume was 50 mL. The final NaOH concentration was 2 mmol L−1. A sub-sample aliquot of the NaOH modified-agarose gel (150 μL) was added to a cuvette within a vial and allowed to set.
2.4 Gel preparation for the detection of aniline
A 0.5% solution of NQS was prepared by dissolving 0.025 g in 5 mL of distilled water. This reagent was prepared fresh daily. Agarose gel (1%) was prepared as described in Section 2.3. To 1% agarose gel, 0.25 mL of 0.5% NQS was added to give a total volume of 50 mL. A sub-sample aliquot of the NQS modified agarose gel (150 μL) was added to a cuvette within a separate vial and allowed to set.
For each gel type, a 1 mL total volume of sample in BHI media was added aseptically to a 1.5 mL Eppendorf tube within a sterilised vial. The BHI media was made up according to manufacturer's guidelines. Sterilisation of media was achieved by autoclaving at 121 °C for 11 minutes. Vials and cuvettes were sterilised by exposure to UV radiation for 90 min in a UV cabinet. The vial with sample was sealed and placed overnight in a 37 °C incubator. The absorbance of the gel in the cuvette was measured at the experimentally determined wavelengths of 415 nm for 2-nitrophenol and 470 nm for the coloured complex formed by aniline/NQS, compared against a reagent blank after 18 h incubation.
2.5 Optimisation of parameters
Aniline and 2-nitrophenol stock solutions were prepared in N-methyl-2-pyrrolidinone. The optimum percentage agarose in gels was determined using 50 μg mL−1 of 2-nitrophenol (or 359 μmol L−1) and 20 μg mL−1 (or 215 μmol L−1) of aniline in 1 mL of BHI media. The optimum volume of gel and NaOH concentration in gel were determined with 50 μg mL−1 2-nitrophenol in 1 mL BHI media following overnight incubation at 37 °C. [Note: as the yellow colour is generated by the ionic form i.e. 2-nitrophenolate (pKa of 2-nitrophenol is 7.23) it was necessary to increase the pH (with NaOH) to generate more of the anion; this resulted in a higher molar extinction coefficient in the presence of NaOH and a more intense colour.] Similarly, optimum NQS and NaOH concentrations in gel were determined with 20 μg mL−1 aniline in 1 mL BHI media. Absorption spectra of gels exposed to 2-nitrophenol and aniline from 300 nm to 700 nm were produced and maximum absorption wavelengths for both VOCs were determined. Calibration curves for both VOCs were produced using a VOC concentration range in BHI media and measuring absorbance after 18 h incubation at 37 °C. Absorbance readings below 0.03 were omitted. An absorption spectrum of NQS measured against agarose gel with no reagents added was also produced. A Pharmacia Biotech Ultraspec 2000 UV/visible spectrophotometer was used for measuring the absorbance of samples and blanks.
2.6 Synthesis of 3-amino-N-phenylpropanamide, TFA salt
3-Amino-N-phenylpropanamide, TFA salt was synthesised using tboc-β-alanine-OH as a starting material. To a stirred solution of tboc-β-alanine-OH (0.50 g, 2.6 mmol) and N-hydroxysuccinimide (0.30 g, 2.6 mmol) in dry dichloromethane (DCM) (10 mL) at room temperature with a CaCl2 trap was added dicyclohexylcarbodiimide (DCCI) (0.55 g, 2.6 mmol). The solution was stirred at room temperature for 1.5 h. To dry DCM (10 mL) was added aniline (0.24 g, 2.6 mmol) and to this was added the contents of the other flask slowly over 1–2 min. The mixture was allowed to stir overnight at room temperature after which the precipitate was filtered and solvent evaporated on a rotary evaporator. The intermediate, tboc-3-amino-N-phenylpropanamide (0.49 g, 71% yield), was purified by column chromatography over silica gel using hexane
:
ethyl acetate (2
:
1, v/v) as the eluent. A portion (0.35 g) of the product was deprotected with trifluoroacetic acid (TFA) (used as solvent and reagent) yielding the TFA salt of 3-amino-N-phenylpropanamide (0.31 g, 84% yield). For structure verification and comparison with literature, 3-amino-N-phenylpropanamide, TFA salt was dissolved in ice-cold water (2 mL), made alkaline with 2 mmol L−1 sodium hydroxide and extracted with chloroform (5 × 2 mL). The combined organic phases were dried with sodium sulphate and the solvent evaporated. Structure verification was achieved using 1H-NMR spectrum (400 MHz) and 13C-NMR spectrum (101 MHz) recorded on a Jeol ECS400 instrument. Low resolution mass spectrum (LRMS) was recorded via direct injection of a dilute methanolic solution into a Thermo Finnigan LCQ Advantage MS detector using electrospray ionisation (ESI). Melting point was determined using Stuart SMP1 melting point apparatus. Analytical data for 3-amino-N-phenylpropanamide, TFA salt and the scheme for synthesis are given in the ESI.†
2.7 Preparation of bacterial samples
All bacteria were sub-cultured overnight at 37 °C on BHI agar one day prior to the preparation of samples. After overnight incubation, bacteria were inoculated in sterile BHI broth and incubated at 37 °C. Samples were prepared by measuring the absorbance of the incubated bacterial suspension at OD600nm. At an absorbance reading of 0.132 (equivalent to 0.5 McFarland units/1.5 × 108 CFU mL−1) an aliquot of 10 μL of bacterial suspension (1.5 × 106 CFU) was added to a 1.5 mL Eppendorf containing 0.99 mL sterile BHI broth with enzyme substrate. Samples were placed in a sterile vial alongside a cuvette containing agarose gel with added reagents and incubated overnight (Fig. 1(a)). Media with enzyme substrate was prepared by dissolving 10 mg of enzyme substrate in N-methyl-2-pyrrolidinone then transferring aliquots aseptically to BHI broth.
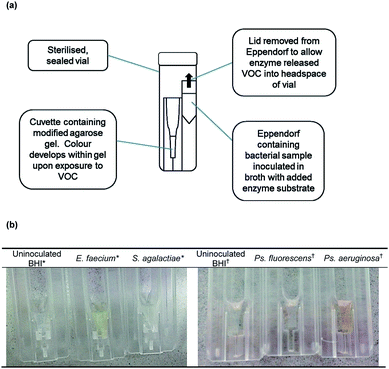 |
| Fig. 1 Exogenous volatile organic compound detection (a) schematic diagram of experimental set-up and (b) application to bacterial samples. *Enzyme substrate: 200 μg mL−1 (or 664 μmol L−1) 2-nitrophenyl-β-D-glucoside; †Enzyme substrate: 100 μg mL−1 (or 359 μmol L−1) 3-amino-N-phenylpropanamide, TFA salt. | |
2.8 Application of optimised methods to bacterial samples
E. faecium, S. agalactiae, E. coli and K. pneumoniae were tested with 2-nitrophenyl-β-D-glucoside. Ps. aeruginosa, Ps. fluorescens and E. coli were evaluated with 3-amino-N-phenylpropanamide, TFA salt. Parameters investigated with E. faecium and 2-nitrophenyl-β-D-glucoside and Ps. aeruginosa with 3-amino-N-phenylpropanamide, TFA salt were substrate concentration and sensitivity in terms of initial inoculum used. All samples were tested after overnight incubation at 37 °C, after which the absorbance of the gel in the cuvette was measured at 415 nm with 2-nitrophenyl-β-D-glucoside and at 470 nm with 3-amino-N-phenylpropanamide, TFA salt, both against reagent blanks. Uninoculated broth with added substrate and bacteria inoculated in broth without substrate were tested to ensure no colour developed due to spontaneous breakdown of the substrate or interfering VOCs. All bacterial samples, as well as uninoculated BHI samples with substrate and uninoculated BHI samples without substrate, were tested in triplicate.
3. Results and discussion
Modified agarose gel has been identified as a suitable matrix for the trapping of exogenous VOCs. It was found that colour development was homogenous throughout the gel, allowing accurate absorbance measurements to be recorded. The reagents required to generate colour were easily added to agarose gels and their optimisation for increased colour generation was straightforward (see ESI†).
3.1 Application of optimised methods to bacterial samples
The structures of both enzyme substrates are shown in Fig. 2. 3-Amino-N-phenylpropanamide has previously been synthesised.14,15 A comparison of the NMR data is given in the ESI.† Bacterial samples were prepared with a final 2-nitrophenyl-β-D-glucoside concentration of 200 μg mL−1 (664 μmol L−1). There was an increase in absorbance and the development of a yellow colour after 18 h incubation with both E. faecium and K. pneumoniae (known producers of β-glucosidase). The generation of a yellow colour indicated that 2-nitrophenol had been trapped in the modified agarose gel. Both S. agalactiae and E. coli showed no activity with 2-nitrophenyl-β-D-glucoside and no yellow colour was generated (Table 1). It was noted that all species were able to grow in the presence of enzyme substrate. 2-Nitrophenol generating bacteria could be visually differentiated from those species negative for 2-nitrophenol production (Fig. 1(b)).
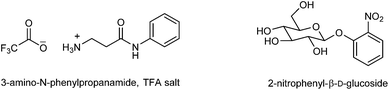 |
| Fig. 2 Structures of 3-amino-N-phenylpropanamide, TFA salt and 2-nitrophenyl-β-D-glucoside. Note: 3-amino-N-phenylpropanamide, TFA salt: empirical formula C11H13F3N2O3; molecular weight 278.23. 2-Nitrophenyl-β-D-glucoside: empirical formula C12H15NO8; molecular weight 301.25 | |
Table 1 2-Nitrophenyl-β-D-glucoside with bacterial samples
Species |
Growtha |
Mean 2-nitrophenol concentration ± 1 SDbd (μg mL−1) |
+, Growth observed.
SD, 1 standard deviation (n = 3).
ND, not detected.
Absorbance measured at 415 nm.
Gram-positive organism.
Gram-negative organism.
|
E. faecium
e
|
+ |
33.50 ± 2.32 |
S. agalactiae
e
|
+ |
NDc |
K. pneumoniae
f
|
+ |
17.31 ± 1.95 |
E. coli
f
|
+ |
ND |
Bacterial samples were tested with a final 3-amino-N-phenylpropanamide, TFA salt concentration of 100 μg mL−1 (or 359 μmol L−1). Ps. aeruginosa hydrolysed 3-amino-N-phenylpropanamide, TFA salt and this was detected by an increase in absorbance of the gel and the generation of an orange colour within the gel. No hydrolysis occurred with Ps. fluorescens and E. coli and no orange colour developed with these species (Table 2). All species grew in the presence of 3-amino-N-phenylpropanamide, TFA salt. Aniline generating bacteria, and therefore β-alanine aminopeptidase producing species, could be differentiated visually from species that did not liberate aniline (Fig. 1(b)). No substrate hydrolysis occurred in uninoculated BHI controls with either enzyme substrate. In addition, there was no colour development in bacterial samples in BHI without the addition of enzyme substrates.
Table 2 3-Amino-N-phenylpropanamide, TFA salt with bacterial samples
Species |
Growtha |
Mean aniline concentration ± 1 SDb,d (μg mL−1) |
+, Growth observed.
SD, 1 standard deviation (n = 3).
ND, not detected.
Absorbance measured at 470 nm.
|
Ps. aeruginosa
|
+ |
6.12 ± 0.75 |
Ps. fluorescens
|
+ |
NDc |
E. coli
|
+ |
ND |
A 2-nitrophenyl-β-D-glucoside concentration range of 50–200 μg mL−1 was tested with E. faecium (Table 3); the optimum substrate concentration was determined to be 200 μg mL−1 (or 664 μmol L−1). An increase in absorbance readings and a yellow colour were detected from a 2-nitrophenyl-β-D-glucoside concentration of 100 μg mL−1 (or 332 μmol L−1); 2-nitrophenol generation continued to increase as the substrate concentration increased up to 200 μg mL−1. A 3-amino-N-phenylpropanamide, TFA salt concentration range of 10–200 μg mL−1 was tested with Ps. aeruginosa (Table 3). Aniline was detected from a 3-amino-N-phenylpropanamide, TFA salt concentration of 20 μg mL−1 (or 72 μmol L−1); the optimum 3-amino-N-phenylpropanamide, TFA salt concentration was 100 μg mL−1 (or 359 μmol L−1). At a 3-amino-N-phenylpropanamide, TFA salt concentration of 200 μg mL−1 (or 719 μmol L−1), aniline generation decreased from that detected at a substrate concentration of 100 μg mL−1. An initial inoculum of 1–1.5 × 104 CFU mL−1 was required for detectable levels of colour to develop with both enzyme substrates after 18 h incubation (Table 4).
Table 3 Exogenous VOC detection over an enzyme substrate concentration range
Substrate concentration (μg mL−1) |
2-Nitrophenol concentrationb (μg mL−1) |
Aniline concentrationc (μg mL−1) |
ND, not detected.
E. faecium with 2-nitrophenyl-β-D-glucoside, absorbance measured at 415 nm.
Ps. aeruginosa with 3-amino-N-phenylpropanamide, TFA salt, absorbance measured at 470 nm.
Not tested at this concentration.
|
200 |
33.48 |
5.32 |
150 |
23.92 |
—d |
100 |
10.46 |
9.63 |
75 |
NDa |
— |
50 |
ND |
8.37 |
20 |
— |
5.84 |
10 |
— |
ND |
Table 4 Sensitivity of modified agarose gels method in terms of initial inoculum used
Initial inoculum (CFU mL−1 BHI) |
2-Nitrophenol concentrationb (μg mL−1) |
Aniline concentrationc (μg mL−1) |
ND, not detected.
E. faecium with 200 μg mL−1 2-nitrophenyl-β-D-glucoside, absorbance measured at 415 nm.
Ps. aeruginosa with 3-amino-N-phenylpropanamide, TFA salt, absorbance measured at 470 nm.
|
1–1.5 × 106 |
31.5 |
8.25 |
1–1.5 × 105 |
27.8 |
6.48 |
1–1.5 × 104 |
16.4 |
5.03 |
1–1.5 × 103 |
NDa |
ND |
1–1.5 × 102 |
ND |
ND |
1–1.5 × 101 |
ND |
ND |
The developed method allowed for the discrimination of β-glucosidase producing bacteria, as well as β-alanine aminopeptidase producing species. Bacterial VOC analysis has previously suffered from a lack of specificity; in addition, experimental variables such as choice of growth medium have been shown to alter the VOCs generated.3 Use of enzyme substrates increases the specificity of bacterial VOC profiles as VOCs released from substrates would act as markers for a particular species.
3-Amino-N-phenylpropanamide, TFA salt demonstrated potential as a suitable substrate for the detection of Ps. aeruginosa. The development of an orange colour indicated aniline liberation and its subsequent coupling with NQS, and thereby signifying β-alanine aminopeptidase activity. A 3-amino-N-phenylpropanamide, TFA salt concentration of between 20–100 μg mL−1 was sufficient for colour generation; this was comparable to β-alanine aminopeptidase enzyme substrate concentration used in chromogenic media.13 However, the absorbance of gels decreased at 3-amino-N-phenylpropanamide, TFA salt concentrations above 100 μg mL−1, possibly due to the increased amount of solvent at higher substrate concentrations as 3-amino-N-phenylpropanamide, TFA salt was less soluble in N-methyl-2-pyrrolidinone than 2-nitrophenyl-β-D-glucoside.
Limitations of the current method include use of a small sample size, testing with a limited range of species and only one strain of each species used. Applying the method to more species is required. As a result of the size of vials used (30 mL), the headspace volume in the vial was large. Use of a smaller vial would decrease the headspace volume, could lead to an improvement in the linear range for quantification purposes, as well as improving method sensitivity and hence increasing the amount of colour developed. An initial inoculum of 1–1.5 × 104 CFU mL−1 was required for colour generation after 18 h incubation with both enzyme substrates. However, using a xerogel, p-nitrophenol was detected after 16 h incubation with an initial E. coli inoculum of 105 CFU mL−1.8 The initial inoculum required to produce a detectable amount of colour could be reduced by decreasing the headspace volume. Following the implementation of a new experimental set-up with a smaller sample headspace volume, a study investigating the minimum time required for sufficient exogenous VOC liberation and detection would be useful. Pure bacterial cultures inoculated in a liquid culture medium were used to demonstrate the proof of concept; however, this method could potentially be applied to the identification of pathogenic bacteria in “real” clinical and food samples.
Producers of β-glucosidase and/or β-alanyl aminopeptidase can be readily detected by inclusion of chromogenic or fluorogenic enzyme substrates in the medium that contains the bacterial cells without the need to detect released VOCs in the headspace, and such methods are well established. However, our approach has several possible advantages. For example, inoculation of foodstuffs or other test samples into enrichment broths may lead to opacity or discolouration that could confound the interpretation of whether a chromogenic substrate has been hydrolysed (or lead to the quenching of released fluorogens, if fluorogenic substrates are used). Furthermore, development of this technology could lead to the design of a sensor that is divided into compartments, each of which contains specific reagents for trapping and visualizing distinct VOCs. This could potentially allow generation of a biochemical profile showing the presence (or absence) of multiple bacterial enzymes within an enrichment broth allowing the detection of bacterial pathogens with high specificity.
In addition, it is also possible to compare the theoretical trapping efficiency of 2-nitrophenol in agarose. This would allow this approach to be directly compared with other trapping media (e.g. xerogels).8 Unfortunately some uncertainty exists in the scientific literature on the values for the molar extinction coefficient and Henry's constant for 2-nitrophenol. For example, the molar extinction coefficient (molar absorptivity) for 2-nitrophenol has been reported to have values that range from 2150–21
300 mol−1 L cm−1.16 In addition, the Henry's constant for 2-nitrophenol also have some variability in their numerical values (e.g. 4.13 × 10−5 and 1.28 × 10−5 atm m3 mol−1).8,17 Similar variability is also evident for aniline. On that basis it is not possible to determine an accurate trapping efficiency for either VOC.
4. Conclusions
This work highlights the potential of designing enzyme substrates to liberate exogenous VOCs for bacterial identification, as well as the suitability of agarose gel as a matrix for VOC trapping. In addition, the ability to tailor reagents present in the gel for the colorimetric detection of specific exogenous VOCs is demonstrated and reagents added to gel can be optimised for maximum colour generation. This innovative method displays potential for the development as a novel, simple and low-cost optical VOC sensor for the detection of bacteria in clinical and food samples.
Acknowledgements
The financial support of Northumbria University is gratefully acknowledged.
References
- S. Schulz and J. S. Dickschat, Nat. Prod. Rep., 2007, 24, 814–842 RSC.
- E. Tait, J. Perry, S. Stanforth and J. R. Dean, TrAC, Trends Anal. Chem., 2014, 53, 117–125 CrossRef CAS PubMed.
- E. Tait, J. Perry, S. Stanforth and J. R. Dean, J. Chromatogr. Sci., 2014, 52, 363–373 CAS.
- M. Junger, W. Vautz, M. Kuhns, L. Hofmann, S. Ulbricht, J. I. Baumbach, M. Quintel and T. Perl, Appl. Microbiol. Biotechnol., 2012, 93, 2603–2614 CrossRef PubMed.
- J. Zhu and J. E. Hill, Food Microbiol., 2013, 34, 412–417 CrossRef CAS PubMed.
- Y. W. Chu, B. Y. Wang, D. A. Engebretson and J. R. Carey, Analyst, 2013, 138, 5879–5885 RSC.
- A. P. Snyder and D. B. Schoff, Anal. Chem., 1991, 63, 526–529 CrossRef CAS.
- L. H. Guillemot, M. Vrignaud, P. R. Marcoux, C. Rivronb and T. H. Tran-Thi, Phys. Chem. Chem. Phys., 2013, 15, 15840–15844 RSC.
- S. Crunaire, P. R. Marcoux, K.-Q. Ngo, J. P. Moy, F. Mallard and T. H. Tran-Thi, Chemosensors, 2014, 2, 171–181 CrossRef PubMed.
- Q. M. Li and Z. J. Yang, Spectrochim. Acta, Part A, 2007, 66, 656–661 CrossRef PubMed.
- F. Feigl, Anal. Chem., 1961, 33, 1118–1121 CrossRef CAS.
- S. Orenga, A. L. James, M. Manafi, J. D. Perry and D. H. Pincus, J. Microbiol. Methods, 2009, 79, 139–155 CrossRef CAS PubMed.
- A. Zaytsev, R. J. Anderson, A. Bedernjak, P. W. Groundwater, Y. Huang, J. D. Perry, S. Orenga, C. Roger-Dalbert and A. James, Org. Biomol. Chem., 2008, 6, 682–692 CAS.
- H. Tsu, X. Chen, C. T. Chen, S. J. Lee, C. N. Chang, K. H. Kao, M. S. Coumar, Y. T. Yeh, C. H. Chien, H. S. Wang, K. T. Lin, Y. Y. Chang, S. H. Wu, Y. S. Chen, I. L. Lu, S. Y. Wu, T. Y. Tsai, W. C. Chen, H. P. Hseih, Y. S. Chao and W. T. Jiaang, J. Med. Chem., 2006, 49, 373–380 CrossRef CAS PubMed.
- A. Göblyös, L. Lázár and F. Fülöp, Tetrahedron, 2002, 58, 1011–1016 CrossRef.
- M. Soundararajan, C. P. Bailey and J. Markwell, Biochem. Mol. Biol. Educ., 2008, 36, 61–64 CrossRef CAS PubMed.
- ChemIDplus. A Toxnet Database. US National Library of Medicine, Bethesda, MD, USA, http://chem.sis.nlm.nih.gov/chemidpus/name/2-nitrophenol, accessed 15 January 2015.
Footnote |
† Electronic supplementary information (ESI) available: The ESI contains details of the optimisation processes for determination of percentage agarose gel, 2-nitrophenol detection, aniline detection, analytical calibration data for 2-nitrophenol and aniline. In addition, experimental details for the synthesis of 3-amino-N-phenylpropanamide, TFA and its analytical data are included as well as analytical data for 3-amino-N-phenylpropanamide. In total eight Figures and three Tables are provided. See DOI: 10.1039/c4ra13914c |
|
This journal is © The Royal Society of Chemistry 2015 |
Click here to see how this site uses Cookies. View our privacy policy here.