DOI:
10.1039/C4RA13024C
(Paper)
RSC Adv., 2015,
5, 8657-8668
Theoretical and experimental investigations on the photoconductivity and nonlinear optical properties of donor–acceptor π conjugated copolymer, poly(2,5-(3,4-ethylenedioxythiophene)-alt-2,7-(9,9-dioctylfluorene))†
Received
18th November 2014
, Accepted 23rd December 2014
First published on 23rd December 2014
Abstract
The donor–acceptor (D–A) π conjugated copolymer, poly(2,5-(3,4-ethylenedioxythiophene)-alt-2,7-(9,9-dioctylfluorene)) (P(EDOT–FL)), a photoconductive and nonlinear optical material has been synthesized via, a direct arylation method using palladium acetate as a catalyst and characterized. The theoretical studies along with photophysical and electrochemical studies confirmed that the copolymer exhibited a relatively lower band gap (2.29 eV) than that of polyfluorene. The photoconductivity properties of the P(EDOT–FL):PC61BM films under various illuminations were studied and high photosensitivity was observed at 488 nm. The field dependence of the photoconductivity in P(EDOT–FL):PC61BM blend films showed that the films could withstand up to 75 V μm−1 and the photosensitivity at higher electric fields was even greater than two lakh (at 75 V μm−1, the photosensitivity was 289
742). At higher electric fields, the photocurrent entered into micro ampere ranges. The intensity dependence on the photocurrent in these films was also investigated. Third-order nonlinear optical properties of P(EDOT–FL) under nanosecond laser excitation at 532 nm have been studied by a Z-scan technique. The polymer exhibited a high nonlinear absorption and refraction coefficient. The polymer also showed a good optical limiting response at 532 nm. These results provide an avenue for application of P(EDOT–FL) in photo conducting and nonlinear optical devices.
Introduction
Over the last three decades, conducting polymers have attracted a great deal of research interest owing to their electrical, electrochemical and optical properties. Nowadays, with the advent of better designs and fabrication technology, a wide variety of conductive polymers are available in optoelectronic device applications. They are promising candidates for commercial applications in photovoltaics,1 light-emitting diodes,2 field-effect transistors,3 sensors,4 and electrochromic devices.5 Synthesis of D–A copolymers appears to be a general strategy to achieve enhanced efficiency compared to that of the parent homopolymers due to a combination of efficiency and versatility by allowing multiple possibilities of combinations of D and A building blocks.6–8 Conjugated polymers based on 3,4-ethylenedioxythiophene (EDOT) have been widely investigated owing to their relatively low ionization potential, high conductivity when doped, and good stability.9,10 These properties enable them to be ideally fit in various applications such as hole transport layer in light emitting devices, design and fabrication of chemical and biological sensors, antistatic coatings, transparent electrodes, and electrochromic displays etc.11–16 Polyfluorenes (PFs) have been widely studied for polymer light-emitting diodes (PLED) because of their processability, high photoluminescence efficiency, high hole mobility and good photostability.17–21 However, its large band gap (in the range of 2.95 eV to 3.68 eV)22,23 combined with poor electron-transport properties results in a large electron-injection barrier and imbalance of charge carrier transport. Copolymerization of fluorene with EDOT moieties has been investigated to improve the shortcomings.
Generally, EDOT–fluorene copolymer with alternating D–A structures were synthesized via, Stille24,25 or Suzuki26–28 coupling reactions or electrochemical polymerization.29–31 In fact, they demand stringent reaction conditions and polymers with high purity results only on substantial product purification. Recently, Anil Kumar et al.32 have developed a new reductive polymerization method for the synthesis of polymers which is economically viable, inert to the presence of functional groups and uses less stringent polymerization conditions. This method was adopted for the preparation of P(EDOT–FL) for utilising the desirable properties of the two monomeric species, EDOT and fluorene. Currently, there are two reports on direct arylation polycondensation for the synthesis of P(EDOT–FL) and high OPV performance with a power conversion efficiency of 4%.33,34
M. Leclerc et al. have reported the synthesis of such copolymer via palladium-catalyzed Suzuki coupling and have attracted great attention due to their thermochromic and solvatochromic properties.27 Also, S. M. Cassemiro et al.26 have synthesized an alternating copolymer of EDOT and fluorene, poly(2,5-(3,4-ethylenedioxythiophene)-alt-2,7-(9,9-dihexylfluorene)) by Suzuki coupling reaction and their investigation revealed the light emitting mechanism in the polymer. EDOT–fluorene copolymers have wide research interest due to their unique electro-optic properties. As a result, these copolymers are potential candidates in electrochromic devices,25,31 organic polymer light emitting diodes,24,26,28 and organic lasers.30 Photoconductivity refers to the increase in electrical conductivity when a material is exposed to electromagnetic radiation of suitable energy. Charge-transfer (CT) complexes are well-known solid-state organic functional materials which are composed of electron-rich molecules/polymers as donor (D) and electron-deficient molecules as acceptor (A). Hence a blend of narrow-band gap donor (P(EDOT–FL)) and PC61BM ([6,6]-phenyl-C61-butyric acid methyl ester) have been investigated in this study, thus forming a novel photoconductive material.
Third-order nonlinear optical materials have attracted a great deal of research interest because of their potential application in optical devices.35–37 Among the wide range of nonlinear optical (NLO) materials, π conjugated organic materials is most promising because of their inherent large third-order susceptibilities, fast response time, processability and good mechanical properties. In organic NLO materials, nonlinearity originates due to strong donor–acceptor intermolecular interaction and delocalized π electrons.38 Therefore nonlinear optical properties can be tuned by adopting suitable synthetic strategies such as copolymerization. Since P(EDOT–FL) is D–A alternate copolymer, it can induce a strong intramolecular charge transfer transition. In this paper, the design, synthetic procedure and properties (electrochemical and photophysical) of the conjugated polymer, P(EDOT–FL) is reported together with photoconductivity studies of P(EDOT–FL):PC61BM blends. Wavelength, intensity and electric field dependence of photocurrent have been studied. The third-order nonlinear optical properties of the P(EDOT–FL) in solution and as film has also been discussed in detail. This work presents a correlation between the theoretical and experimental results aiming to add further information about photorefractive properties of this material.
Experimental section
Materials
3,4-Ethylenedioxythiophene (EDOT, Aldrich, 98%), 2,7-dibromofluorene (Aldrich, 97%), magnesium sulphate (anhydrous) (MgSO4, Spectrochem Pvt. Ltd), tetrabutyl ammonium bromide (TBAB, Avra Synthesis Pvt. Ltd, 98%), sodium acetate (anhydrous) (Spectrochem Pvt. Ltd), PC61BM (Solenue BV, >99%), potassium hydroxide (KOH, Spectrochem Pvt. Ltd), palladium(II) acetate (Pd(OAc)2, Aldrich, 99.98%), tetrabutyl ammonium hexafluorophosphate (Bu4NPF6, Aldrich, >99%), and 1-bromooctane (Avra Synthesis Pvt. Ltd, 98%) were used as received. Acetonitrile HPLC grade (Aldrich), dimethyl acetamide (anhydrous) (DMAc, Spectrochem Pvt. Ltd), chloroform (CHCl3, Spectrochem Pvt. Ltd), dimethyl sulphoxide (DMSO, Spectrochem Pvt. Ltd), dicholoromethane (DCM, Spectrochem Pvt. Ltd), tetrahydrofuran HPLC grade (THF, Spectrochem Pvt. Ltd), dichlorobenzene (Spectrochem Pvt. Ltd) and methanol (anhydrous) (MeOH, Spectrochem Pvt. Ltd) were dried and distilled when necessary according to standard procedures.
Synthesis methods
Synthesis of 2,7-dibromo-9,9-dioctylfluorene (II). In a three necked round bottom flask, KOH (8.0 g) was dissolved in 100 ml DMSO and degassed under N2. After degassing, 2,7-dibromofluorene (1.03 g, 6.77 mmol) and TBAB (1.0 g, 3.08 mmol) were added to the stirred solution and kept for 30 min. 1-Bromooctane was added in small portions under stirring and, the reaction was allowed to proceed for 24 h at 80 °C. The solution was cooled and poured into distilled water. The organic layer was extracted with DCM and solvent was removed under vacuum by rotary evaporation. Finally it was purified twice using chloroform and activated charcoal and washed with cold MeOH until pale yellow powder was obtained with 80% yield. Mp 62 °C (from chloroform), 1H NMR (400 MHz, CDCl3, δ): 0.40–0.60 (m, 4H), 0.70–0.92 (t, 6H), 1.02–1.25 (m, 20H), 1.85–1.91 (m, 4H), 7.41–7.48 (3d, 6H).
Synthesis of poly(2,5-(3,4-ethylenedioxythiophene)-alt-2,7-(9,9-dioctylfluorene)). To a stirred solution of EDOT (0.024 g, 0.17 mmol) in 10 ml DMAc was added TBAB (0.05 g, 0.17 mmol) and sodium acetate (0.05 g, 0.68 mmol). The reaction mixture was stirred at room temperature for 30 min followed by addition of 2,7-dibromo-9,9-dioctylfluorene (0.1 g, 0.17 mmol) and 10% palladium acetate (0.003 g). The reaction mixture was stirred at 90 °C for 48 h. The mixture was cooled to room temperature and poured in to methanol (20 ml). The precipitate was filtered and washed with methanol. The polymer was purified by soxhlet extraction using hexane and methanol for 24 h. The residue was dissolved in minimum amount of CHCl3 and precipitated using methanol and dried under vacuum. Yield: 61%, FT-IR νmax/cm−1 2918s and 2852s (CH), 1086s and 1050w (CO), 1667br (conjugation), 1H NMR (400 MHz, THF, δ): 0.7–0.8 (m, 10H), 1.1–1.3 (m, 20H), 2.06 (br, 4H), 4.2–4.5 (m, 4H), 6.9–7.8 (m, 6H).
Instrumentation
1H Nuclear magnetic resonance (1H NMR) spectrum was recorded on JEOL, JNM-EX400, FT NMR SYSTEM operating at 400 MHz and chemical shifts were quoted down field of TMS. Fourier transform infra-red (FT-IR) analysis was obtained from Perkin-Elmer Spectrum 100, FT-IR Spectrometer. The copolymer was characterized by X-ray Photoelectron Spectroscopy (XPS) measurements (Kratos Analytical XPS), which was carried out using a monochromatic Al anode at energy of 40 eV. Gel permeation chromatography (GPC) was carried out with Waters GPC with THF as the eluent. All the electrochemical experiments were performed in dry acetonitrile with Bu4NPF6 (the supporting electrolyte) using BAS Epsilon Electrochemical analyser with quiet time of 2 s and scan rate of 100 mV s−1. Absorption spectra were recorded with Thermo Scientific, Evolution 201, ultraviolet-visible (UV-visible) spectrophotometer. Fluorescence spectra were recorded with DT 1000CE, fluorescence spectrophotometer and were corrected for the spectral response of the machine. Thermogravimetric measurements (TG and DTG) were performed on a TA Instrument Q 50 Thermogravimetric analyzer with a continuous nitrogen flow and a heating rate of 10 °C min−1. Differential scanning calorimetry (DSC) was carried out on TA Instrument Q 100 with a continuous nitrogen flow and a heating rate of 2 °C min−1. The steady state photocurrent measurements were done using Keithly-236 Source Measure Unit. He–Ne laser (632.8 nm, Melles Griot), diode pumped solid state laser (532 nm, Coherent), and optically pumped semiconductor laser (488 nm, Coherent) were used as different laser sources.
Computation methods
To determine the lowest energy structure of the P(EDOT–FL) copolymer, a sequence of steps were followed involving quantum mechanical calculations, based on density functional theory (DFT) methods.39 Initially, the ground state geometries of oligomers were optimized by means of the DFT at the B3LYP (Becke, three parameter, Lee–Yang–Parr)40–42 level of theory using 6-31G basis set. In order to correlate the experimental data with theory, DFT calculations at three different energy levels were carried out using B3LYP, HSE06 (Heyd–Scuseria–Ernzerhof)43,44 and LSDA (Local Spin Density Approximation)45 on 6-31G basis set as implemented in Gaussian 09 package.46 The periodic boundary condition (PBC) was used to study the electronic properties of P(EDOT–FL) copolymer. The starting unit cell geometries for the PBC calculation was taken from the central portion of the optimized oligomer and optimized inside a given lattice length on the constraints of PBC by assuming that the unit cell is repeated identically an infinite number of times along the translation vector. In the main chain of the polymer, long alkyl groups have been replaced by methyl group to reduce the time of calculation and to avoid the frequently encountered convergence problems. It has been assumed that the presence of alkyl groups does not significantly affect the equilibrium geometry, and hence, the opto-electronic properties. Band structure in the positive region of the first Brillouin zone (between k = 0 and k = π/a) was plotted. i.e., the lowest 4 unoccupied and highest 4 occupied bands in the positive region of the first Brillouin zone were plotted.
Preparation of sandwich cells
For photoconductivity measurements, a device configuration consisting of glass/ITO/P(EDOT–FL):PC61BM/Ag was used. The samples were prepared by spin casting of 5 wt% polymer solution (at 1
:
1 ratio) in dried spectroscopic grade chloroform onto ITO coated glass plates. The ITO layer had a thickness of 200 nm. The solution was filtered using a 0.45 μm PTFE filter prior to spin casting. After drying at room temperature for 12 h, the samples were transferred to a vacuum desiccator and kept for complete solvent removal. Thickness of the films was determined using a Dektak 6M Stylus profiler. The prepared films had a thickness of 800 nm. Finally, silver electrodes of area 2 × 2 mm2 were vacuum deposited on to the films to complete the sandwich structure. The silver electrode had a thickness of 50 nm.
NLO measurements
NLO measurements were performed by the single beam Z-scan technique with nanosecond laser performed with a Q-switched Nd:YAG laser system (Spectra Physics LAB-1760) continuum with pulse width of 7 ns at 10 Hz repetition rate and 532 nm wavelength. The sample was moved in the direction of light propagation near the focal spot of the lens with focal length of 200 mm. The radius of the beam waist was calculated to be 42.56 μm. The Rayleigh length, Z0 = πωo2/λ, was calculated to be 10.69 mm, which was greater than the thickness of the sample cuvette (1 mm), an essential requirement for Z-scan experiments. The Z-scan system was calibrated using CS2 as the standard. The transmitted beam energy, reference beam energy and their ratios were measured simultaneously by an energy ratiometer (REj7620, Laser Probe Corp.) having two identical pyroelectric detector heads (Rjp 735). The effect of fluctuations of laser beam was eliminated by dividing the transmitted power by the power obtained at the reference detector; both being measured using identical photo detectors. The data were analysed according to the procedure described by Bahae et al.,47 and the nonlinear coefficients were obtained by fitting the experimental Z-scan plot with the theoretical plots.
Results and discussion
Polymer synthesis
P(EDOT–FL) have been synthesized via direct arylation of EDOT with 2,7-dibromo-9,9-dioctylfluorene in dry DMAc and 0.01 mol% palladium acetate served as catalyst. Polymer was obtained in moderate yield (61%). To increase the solubility of targeted copolymer, two bulky octyl chains were introduced to the fluorene building unit. Hence P(EDOT–FL) exhibited amenable solubility in organic solvents such as CH2Cl2, CHCl3, THF and dichlorobenzene. This provides the processability required for electronic device applications. The molecular weight of the P(EDOT–FL) was evaluated by gel permeation chromatography in THF referring to polystyrene standards. Copolymer exhibited number average molecular weight (Mn) of 6994 and weight average molecular weight (Mw) of 7106 with polydispersity index (PDI) of 1.01. Scheme 1 illustrates the synthesis route used for the preparation of P(EDOT–FL).
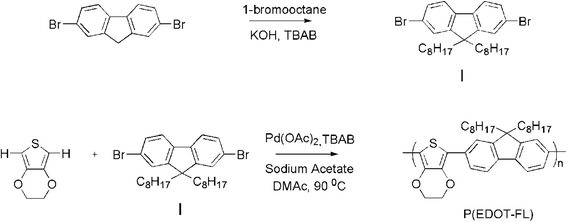 |
| Scheme 1 Synthesis of P(EDOT–FL) by direct arylation. | |
Structural characterization
P(EDOT–FL) copolymer was characterized by FT-IR (ESI: Fig. S1†), 1H NMR (ESI: Fig. S2†) and XPS. FT-IR spectrum of the P(EDOT–FL) showed intense peaks at 2918 and 1364 cm−1 characteristic of aliphatic C–H stretching and bending vibration of fluorene (FL) unit respectively. The C–O–C stretching vibration arising from bridged C–O in the ethylenedioxy group of EDOT was revealed at 1086 and 1050 cm−1 and ethylenedioxy ring deformation mode at 920 cm−1.48,49 The broad band observed at 1667 cm−1 proves polyconjugation in the copolymer.50 Peaks at 814, 845 and 943 cm−1 are assigned to the C–H out-of-plane vibration of the phenyl ring of FL unit and the peak at 1060 cm−1 is due to C–H in-plane vibration of the phenyl ring of FL unit.51 The intense peaks at 2918 and 1086 cm−1 were attributed to the incorporation of EDOT–FL units into the polymer structure during copolymerization. From the results of FT-IR analysis, the successful achievement of copolymerization was revealed. The structure of the polymer was further confirmed with 1H NMR spectra. P(EDOT–FL) showed multiplets at δ 0.8–2 ppm region due to alkyl protons. Resonance peaks corresponding to –O–CH2– protons of EDOT units were observed at δ 4.2–4.5 ppm as multiplet. The broad peak corresponding to aromatic protons observed at δ 6.9–7.8 ppm were confirmed to be the part of FL units. The characteristic signals at δ 0.8–2 and 6.9–7.8 ppm were attributed to FL units and δ 4.2–4.5 ppm are related to EDOT units. They are in good agreement with the FT-IR results. In order to get more insight into the polymer structure XPS investigation was also carried out. The XPS survey scan is given in Fig. 1.
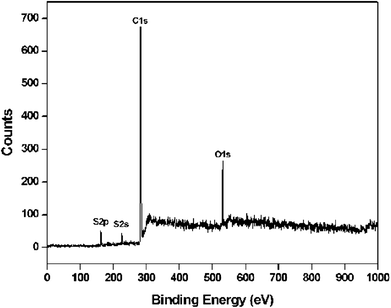 |
| Fig. 1 XPS survey scans of P(EDOT–FL). | |
XPS survey scans of the copolymer revealed the presence of C, O, S as the characteristic elements of the co-monomers. As seen from the Fig. 2, the C–C and C–H functional groups are centered at 282.51 eV and C–S and C–O bonds of EDOT moiety were revealed at 284.09 and 285.0 eV, respectively.52 The results obtained from XPS of P(EDOT–FL) are in good agreement with those corresponding to FT-IR and 1H NMR.
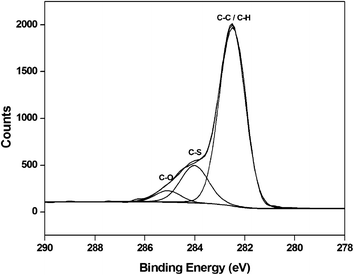 |
| Fig. 2 XPS C1s high-resolution spectra of P(EDOT–FL). | |
Theoretical calculation
Quantum mechanical calculation at the DFT level has been carried out to examine the interaction between EDOT and FL units when they are directly linked in copolymer chain. In the present work, we have used B3LYP, HSE06 and LSDA combined with 6-31G basis set to calculate the electronic properties of P(EDOT–FL) copolymer. Here, periodic boundary condition (PBC) calculation was used to study the electronic properties of the P(EDOT–FL), because, it is more computationally cost effective than oligomer approach. In PBC calculation, polymer molecule of infinite chain length is optimized using translational symmetry. The energy levels of the HOMO (highest occupied molecular orbital) and LUMO (lowest unoccupied molecular orbital) were determined from the maximum point of the highest occupied crystal orbital and the minimum point of the lowest unoccupied crystal orbitals, respectively. The band gap corresponded to the lowest energy difference between the HOMO and LUMO energy levels at a constant k.
Recently, S. M. Cassemiro et al.26 have demonstrated the use of oligomer approach in poly[2,5-(3,4-ethylenedioxythiophene)-alt-(9,9′-dihexyl-2,7-fluorene)] to generate the band gap of 2.62 eV and 2.46 eV, showing good agreement with that obtained experimentally (2.50 eV). Error of about 4.6% and 1.0%, respectively resulted. Recently, electronic properties of vinylene-linked heterocyclic conducting polymers were calculated using DFT method in periodic boundary condition approach.53 Newly introduced Heyd–Scuseria–Ernzerhof (HSE) functional incorporates a screened Hartree–Fock interaction, more computationally efficient than traditional hybrid functionals like B3LYP. The obtained HOMO and LUMO values are summarized in Table 1. For the sake of comparison PBC calculation of poly(dioctylfluorene) (P(FL)) was also carried out. It can be seen from Fig. 3 that lowest band gap occur at Γ point (k = 0), suggesting that the copolymer was direct band gap polymer.
Table 1 Band structure data of P(EDOT–FL)
Sample |
HOMO (eV) |
LUMO (eV) |
Band gap (eV) |
Estimated from DFT/B3LYP/6-31G calculation. Estimated from DFT/HSE06/6-31G calculation. Estimated from DFT/LSDA/6-31G calculation. |
P(EDOT–FL) |
−4.95a |
−1.44a |
3.51a |
−4.82b |
−1.70b |
3.12b |
−4.92c |
−2.64c |
2.28c |
P(FL) |
−5.24a |
−1.30a |
3.94a |
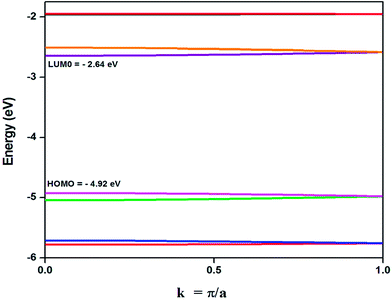 |
| Fig. 3 Band structure of P(EDOT–FL) obtained from DFT/LSDA/6-31G calculation. | |
From the theoretical results it was found that band gap from LSDA was more comparable to experimental values than B3LYP and HSE06. The HOMO and LUMO values obtained for P(EDOT–FL) were −4.92 eV and −2.64 eV, respectively. Meanwhile, HOMO and LUMO values for P(FL) were −5.24 eV and −1.30 eV respectively. As seen from band structure it can be seen that the introduction of EDOT unit increased the energy of HOMO level of P(FL) by a factor of 0.3 eV, while LUMO level is decreased by a factor of 1.34 eV and we get P(EDOT–FL) with a reduced band gap of 2.28 eV.
Thermal properties of P(EDOT–FL)
Thermal properties of the copolymer P(EDOT–FL) was investigated by both TG and DSC analysis. Fig. 4 shows the TG, DTG and DSC (insert figure) traces of (PEDOT–FL). In TGA of the copolymer there is an exotherm coincident with mass loss due to decomposition under nitrogen atmosphere. Decomposition onset was defined as a mass loss of 2%. The peak decomposition temperature was defined as the first inflection point in the thermogravimetric curve, corresponding to peak in the derivative of TG data. On the basis of TGA exotherm, the onset of degradation and degradation temperature were 354 and 430 °C, respectively.
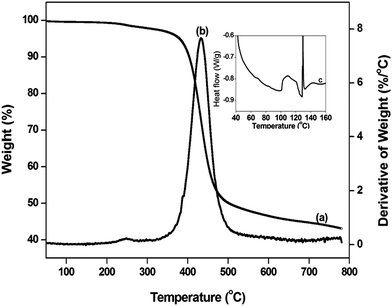 |
| Fig. 4 (a) TG, (b) DTG, and (c) DSC (insert figure) traces of P(EDOT–FL). | |
As revealed by TGA, the polymer exhibited excellent thermal stability, which is higher than that of polyfluorene degradation temperature (400 °C) implying that the polyfluorene backbone has become more rigid upon the incorporation of EDOT units in the copolymer.22 This is possibly because of the EDOT groups encourage stronger inter chain van der Waals bonding. The glass transition temperature (Tg) estimated from DSC traces was 123 °C, which is higher than that of polyfluorene with 67 °C.22 These results allow for the use of high temperature sealing procedures in device fabrication.
Optical and electrochemical properties and band gap evaluation
Absorption spectra of the copolymer P(EDOT–FL) in THF and in spin coated films are shown in Fig. 5. In the absorption spectrum, the band with absorption maximum below 350 nm is due to π–π* transition of the backbone and the peak at longest wavelength is attributed to the intermolecular charge transfer (ICT) band. As seen from the Fig. 5, the optical absorption edges of the copolymer P(EDOT–FL) in solution was 540 nm from which the optical band gap (Eoptg) was calculated to be 2.29 eV (from the onset of low energy transition). Meanwhile, the absorption edges of the polymer in thin film form was 586 nm for P(EDOT–FL), from which Eoptg was calculated to be 2.12 eV. Thus ICT band edge is red shifted by 46 nm compared to P(EDOT–FL) in solution. Significant redshift of the ICT band in the film state than in the solution indicates the presence of strong dipole–dipole interactions of these polymers in solid state. This is attributable to small permanent dipole moment in the film, which does a major role in solid-state packing in the polymer. As expected, P(EDOT–FL) exhibited low band gap compared to polyfluorene.22,23 This difference reflects the strong donor property of the EDOT moieties, which raises the HOMO level and decreases the LUMO level, leading to low band gap. This finding is also consistent with previous reports.28 This Eoptg values agree well with theoretical values obtained by DFT/LSDA/6-31G than those obtained by DFT/B3LYP/6-31G and DFT/HSE06/6-31G calculation. To improve the efficiency of P(EDOT–FL) devices, the concept of bulk heterojunction was introduced, in which the interfacial area between the donor and acceptor was increased. Here an n-type material having high electron affinity, PC61BM was used as strong acceptor. Optical properties of P(EDOT–FL):PC61BM (1
:
1) blend was studied both in solution and in the solid state. P(EDOT–FL) exhibits similar absorption spectral features both in pristine films and in blend films, but a red shift towards longer wavelength (at 605 nm) was revealed due to polaron formation or inter charge transition between P(EDOT–FL) and PC61BM. This reveals that the molecular organization of P(EDOT–FL) was kept unchanged in the blend films. The λmax of P(EDOT–FL) in blend with PC61BM was also improved for 1
:
1 ratio i.e., an increase from 444 nm to 455 nm. The presence of EDOT units led to more structured material through S–O interaction, which permits to obtain more planar chains and thus more structured materials even in the presence of PC61BM.
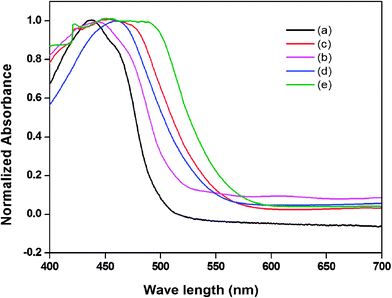 |
| Fig. 5 Absorption spectra of (a) P(EDOT–FL) in solution, (b) P(EDOT–FL) as film, (c) P(EDOT–FL):PC61BM (1 : 1) as film, (d) P(EDOT–FL):PC61BM (1 : 2) in solution, and (e) P(EDOT–FL):PC61BM (1 : 2) as film. | |
Electrochemical methods provide an excellent means of establishing HOMO and LUMO energies. Electrochemical properties of the P(EDOT–FL) was investigated by cyclic voltammetry (CV) and differential pulse voltammetry (DPV) (Fig. 6). CV and DPV were performed in a solution of Bu4NPF6 (0.1 M) in dry acetonitrile at 100 mV s−1 under nitrogen atmosphere. The setup was a three-electrode configuration with a Ag/Ag+ reference electrode, a platinum button electrode (0.08 cm2) coated with the thin copolymer film as working electrode, and a platinum wire as counter electrode.
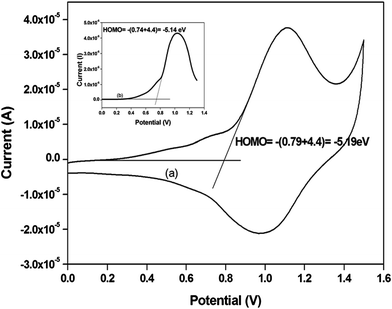 |
| Fig. 6 CV (a) and DPV (insert picture) (b) curves of P(EDOT–FL) film on Pt electrode in 0.1 M Bu4NPF6 solution in acetonitrile at a scan rate of 100 mV s−1. | |
HOMO level of the P(EDOT–FL) was calculated using the formula, HOMO = −(4.4 + Eonsetox) eV and LUMO level was estimated using the optical band gap. The values are −5.19 eV and −2.9 eV, respectively. Moreover, from DPV, HOMO and LUMO was calculated to be −5.14 eV and 2.86 eV, respectively. The DPV estimated HOMO–LUMO levels were found to be smaller than those determined by CV. This may be due to reduced background current in DPV and sharper redox onset compared to the CV. In conclusion, incorporation of EDOT unit to the P(EDOT–FL) chain effectively increases the HOMO level and reduces the LUMO level of the polymer, because of more planar structure and conjugation.
Photoluminescence properties
The photoluminescence spectrum (PL) of the P(EDOT–FL) in dilute solution and as solid film (PC61BM blend) is shown in Fig. 7. The wavelength corresponding to the absorption maximum of each polymer was used as excitation wavelength. The emission maximum of the P(EDOT–FL) occur at 514 nm. Before testing the photoconductivity of P(EDOT–FL) in blend with PC61BM in devices, fluorescence measurements were performed to examine the charge transfer (CT) efficiency from the copolymer (donor) to PC61BM (acceptor). There is a drastic decrease in PL emission of P(EDOT–FL) after the incorporation of PC61BM. Poor PL efficiency could be attributed to the increase in intermolecular charge transfer between the polymer matrix and PC61BM units. The significant fluorescence quenching is a strong evidence to the efficient charge transfer from the photoexcited P(EDOT–FL) conjugated backbone to PC61BM and thus this system can be used in photoconductive applications.
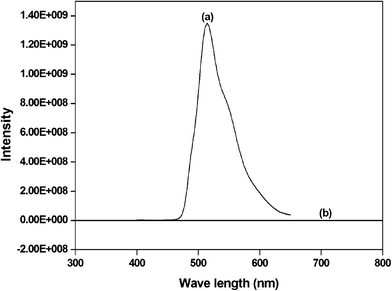 |
| Fig. 7 PL spectra of the polymer (a) P(EDOT–FL) in solution (1 : 0) and (b) P(EDOT–FL):PC61BM (1 : 1) as blend film. | |
Photoconductivity
Heterojunction photoconductive device was fabricated using the P(EDOT–FL) as the electron donor and PC61BM as the electron acceptor. The energy diagram presented in Fig. 8 shows the potential of P(EDOT–FL) to serve as appropriate host for PC61BM and hence efficient charge transfer and better photoconductivity. In this device, the donor and acceptor materials are mixed together and excitons can easily access the donor–acceptor interface and dissociate to free charge carriers.54,55 From the theoretical as well as experimental data, it was found that the polymer P(EDOT–FL) has narrow band gap which is very much suitable for photoconductive application. Hence devices were fabricated with a typical sandwich structure of glass/ITO/(PEDOT–FL):PC61BM/Ag and the steady state photocurrent measurements were performed using Keithly-236 Source Measure Unit.
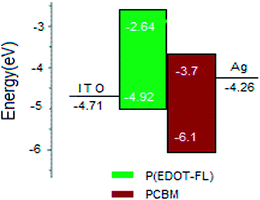 |
| Fig. 8 Energy level diagram of P(EDOT–FL) and PC61BM blend in comparison with work functions of common electrodes used in the device. | |
Photocurrent measurement in the steady state involves measuring the steady state DC current through the sandwich cell with and without light. For this, DC voltage was applied to the sandwich cell which is kept in the dark and the resulting current was measured with respect to time. After the dark current through the cell was stabilized, light was allowed to fall on the ITO surface. Due to the photoinduced change in conductivity, the current increases suddenly and reaches a steady state. If ID and IL are the steady state current values, prior to illumination and after illumination respectively, the photosensitivity of the polymer device can be written as,
Under dark conditions, the P(EDOT–FL):PC61BM device produced a very low current (10−11 A), meaning an “off ” state with a very low concentration of charge carriers. But after the exposure to a pulsed light, the charge transfer process from the donor to acceptor led to high photoconductivity in P(EDOT–FL):PC61BM without any thermal treatment and photosensitivity obtained was found to be 415 at an applied field of 3.75 V μm−1 and laser power density of 10 mW cm−2. Indeed, the alternative D–A arrangement ensures a stable CT and avoids phase separation and resulting in good photoconductivity. Further increasing the PC61BM content (1
:
2) in the polymer blends led to significant decrease of film quality and thereby efficiency.
Dependence of photocurrent on intensity of illumination was studied at three different photon energies in blend film and is shown in Fig. 9. The intensity of the laser beam was varied using a polarizer. The sample exhibited a good photo response at 488 nm. Electric field dependence of photoconductivity at 488 nm was also investigated (Fig. 10).
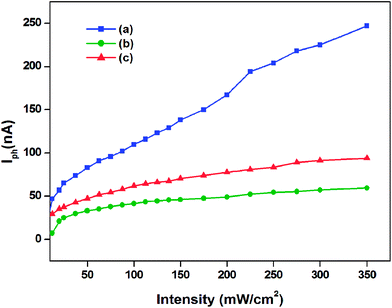 |
| Fig. 9 Intensity dependence of photocurrent of P(EDOT–FL) blend at 3 photon energy values (a) 488 nm, (b) 532 nm, and (c) 632 nm (at an applied field of 3.75 V μm−1). | |
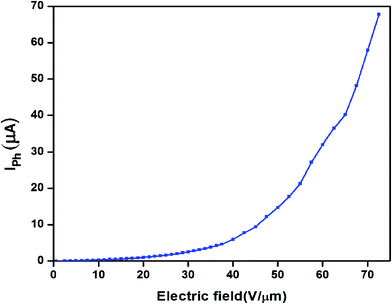 |
| Fig. 10 Electric field dependence of photocurrent of blend film at 5 mW cm−2. | |
Generally, in organic semiconductors, photoconductivity is not through a direct generation of carriers, but through the formation and subsequent dissociation of an exciton. The exciton can dissociate at impurity sites, interfaces with asymmetric ionization potentials or it can be dissociated by a strong electric field.56,57 The photogenerated carrier move within the transporting medium in the general direction of the applied electric field. Field dependences of photogeneration quantum yields of charge carriers in films of amorphous molecular semiconductors at high external electric field strengths cannot be always explained in terms of the Onsager or the Poole–Frenkel model. It is expected that an external electric field shifts the electron density in molecules from its equilibrium distribution, thus altering the probability of electronic transitions resulting in changes not only in intermolecular electronic transitions but also in probabilities of intramolecular transitions.58 As seen in Fig. 10, it is observed that the films can withstand upto an electric field of 75 V μm−1 and the photosensitivity at higher electric fields was even greater than two lakh (at 75 V μm−1, the photosensitivity is 289
742). At higher electric fields, the photocurrent was of the order of micro ampere range and showed a steep increase in the current with respect to field. The photogeneration efficiency for carrier generation is strongly dependant on applied field, since; polymers have a low dielectric constant.59 The sensitivity of the polymer to light, expressed as photoconductive sensitivity. i.e., the photoconductivity per unit light intensity, can be calculated as,
where
σ is the photoconductivity,
I is the light intensity,
P is the laser power,
d is the thickness and
V is the applied voltage. At 10 V μm
−1, for 488 nm, the photoconductive sensitivity was calculated to be 7.6 × 10
−10 S cm W
−1, which is significantly higher than other polymer systems such as polybenzoxazine–C
60 (
ref. 60) and PVK:TNF:DANS.
61 This photoconductive sensitivity is sufficiently high for photorefractive effect.
Nonlinear optical properties
The third-order NLO properties of P(EDOT–FL) was investigated using Z-scan technique, which was distinguished for its simplicity and sensitivity. The open-aperture (OA) Z-scan trace of P(EDOT–FL) in dilute CHCl3 (a) and as thin film (b) is shown in Fig. 11.
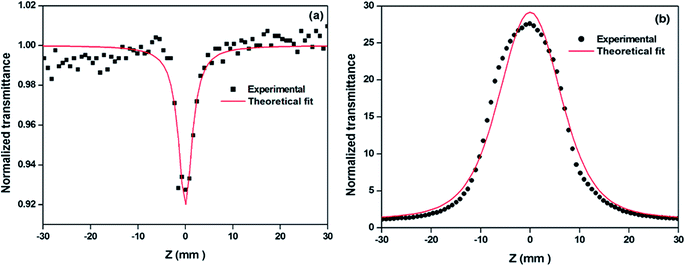 |
| Fig. 11 Open aperture Z-scan traces of P(EDOT–FL) (a) in solution and (b) as film at 112 μJ. | |
In solution phase, the P(EDOT–FL) shows a normalized valley, indicating that polymer is behaving as reverse saturation absorber (RSA) with a positive NLO absorption coefficient. However, in film phase the copolymer is behaving as a saturation absorber (SA). This is due to ground state band bleaching in film phase62 i.e., the excited state absorption and free carrier absorption leading to ground state band bleaching in the polymer. The nonlinear absorption of the copolymer was fitting well by two photon absorption (TPA). The nonlinear absorption coefficient, β is obtained by fitting the experimental scan plot of the OA measurement to eqn (3).
|
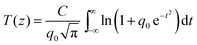 | (3) |
where
q0(z,r,t) =
βI0(
t)
Leff and
Leff = (1 − e
−αl)/
α is the effective thickness with linear absorption coefficient
α, and
I0 is the irradiance at focus. The solid curves in
Fig. 11 are the theoretical fit to the experimental data. The imaginary part of the third-order susceptibility is given by
eqn (4).
|
Im X(3) = n02c2β/(240π2ω)
| (4) |
where
n0 = 1.45 is the linear refractive index of the polymer solution,
c is the velocity of light in vacuum,
ω is the angular frequency of radiation used.
In order to determine the sign and magnitude of nonlinear refraction (NLR) property of P(EDOT–FL), closed-aperture (CA) Z-scan technique was performed by placing an aperture in front of the detector. The pure NLR Z-scan curves after excluding nonlinear absorption effects was assessed from the ratio of CA normalized Z-scan data to the corresponding normalized OA data. The normalized transmittance T(z) for pure NLR is given by eqn (5).
|
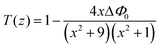 | (5) |
where
T(
z) is the normalized transmittance for the pure refractive nonlinearity at different
z,
Φ0 is on-axis nonlinear phase shift and
x is given by
z/
z0. The CA trace of P(EDOT–FL) is shown in
Fig. 12. The polymer exhibits peak–valley characteristics, indicating the negative NLR index due to self-defocusing. The nonlinear refractive index (
n2), the real parts of
χ(3) (Re
χ(3)) and third-order nonlinear susceptibility (
χ(3)) are calculated by the following
eqn (6)–(8).
|
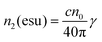 | (6) |
|
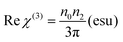 | (7) |
|
χ(3) = Re χ(3) + iIm χ(3)
| (8) |
where
γ is the molecular cubic hyperpolarizability of polymer which can be estimated through the following equations

and

where
k is wave vector.
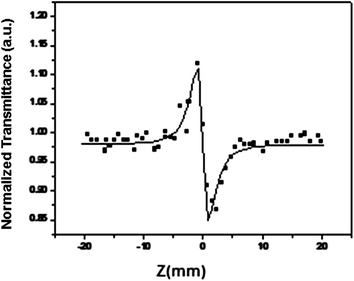 |
| Fig. 12 Closed aperture Z-scan trace of P(EDOT–FL) at 112 μJ. | |
The calculated values of nonlinear absorption coefficient (β, m W−1), the nonlinear refraction coefficient (n2, esu) and the third-order nonlinear susceptibility (χ(3), esu) are given in Table 2. The β value of P(EDOT–FL) is obtained to be 3.19 × 10−10 m W−1. Furthermore, the χ(3) value is found to be 1.25 × 10−11 esu. As expected, P(EDOT–FL) show large optical nonlinearity, due to donor–acceptor scheme. Here, nonlinearity is mainly due to the charge transfer from donor to acceptor unit i.e., due to strong delocalization of π electrons.
Table 2 Calculated values of nonlinear absorption, nonlinear refraction, and nonlinear susceptibility of P(EDOT–FL)
Copolymer |
Nonlinear absorption coefficient (β, m W−1) |
Nonlinear refractive index (n2, esu) |
Imaginary part of nonlinear susceptibility (Im χ(3), esu) |
Real part of nonlinear susceptibility (Re χ(3), esu) |
Nonlinear susceptibility (χ(3), esu) |
P(EDOT–FL) |
3.19 × 10−10 |
−0.68 × 10−10 |
0.07 × 10−10 |
−1.04 × 10−11 |
1.25 × 10−11 |
Optical power limiting
Optical limiters are materials in which they are transparent at low input fluences, but become opaque at high inputs,63 due to enhanced absorption from the excited state or multiphoton absorption or both.64 Such materials can be used for the protection of eyes and sensitive optical devices from laser induced damage. The criteria required for a good optical limiter are low limiting threshold, high linear transmittance throughout the sensor band width, stability etc. Optical limiting experiment was performed at 532 nm using OA Z-scan technique. The optical limiting process has different origins, such as TPA, free carrier absorption, RSA, self-focusing, self-defocusing and induced scattering.65 Fig. 13 shows the transmitted energy of P(EDOT–FL) as a function of input fluence. From the figure it could be seen that at low input fluence, the output varies linearly with input power. The deviation from linearity is noted as optical limiting threshold. Optical limiting threshold of P(EDOT–FL) is determined to be 0.47 GW cm−2. The P(EDOT–FL) exhibits low limiting threshold due to the presence of donor–acceptor units in the polymer i.e., due to the large variation in excited state dipole moment.
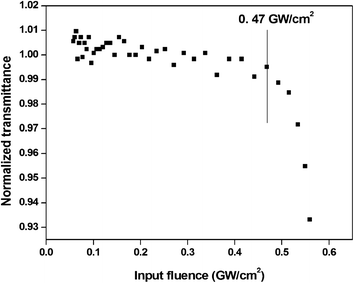 |
| Fig. 13 Optical limiting curves of P(EDOT–FL). | |
Conclusions
In summary, this paper reports the synthesis and characterization of a copolymer containing fluorene and EDOT monomer units. The copolymer has been synthesized via direct arylation method. The optical, electrochemical, photoluminescence, photoconductive and third-order nonlinear optical properties were evaluated. The copolymer revealed good thermal stability and amenable solubility. DFT computations have been performed for analysing the electronic properties and the band gap (Eg) of the P(EDOT–FL) was found to be 2.28 eV, using LSDA/6-31G level. The band gap of the copolymer was obtained to be 2.29 eV from the electronic absorption spectrum of copolymer which is in good agreement with theoretical band gap. Theoretical and experimental studies revealed that the copolymer film exhibited better properties than that of P(FL). Photocurrent measurements were done in the sandwich cell configuration as blend with PC61BM (1
:
1). Photoluminescence quenching and moderate photoconductive sensitivity (7.6 × 10−10 S cm W−1) confirmed the suitability of the copolymer for fabricating photoconductive devices. The third-order nonlinear optical properties of P(EDOT–FL) was investigated by Z-scan technique. The polymer show negative nonlinear refraction and switch over from RSA to SA behaviour was observed as the sample changes from the solution to the thin film. The nonlinear absorption coefficient (β), the nonlinear refraction coefficient (n2), the third-order nonlinear susceptibility (χ(3)) and the optical limiting threshold of polymer were determined to be 3.19 × 10−10 m W−1, −0.68 × 10−10 esu, 1.25 × 10−11 esu and 0.47 GW cm−2, respectively.
Abbreviation
(D–A) | Donor–acceptor |
(P(EDOT–FL)) | Poly(2,5-(3,4-ethylenedioxythiophene)-alt-2,7-(9,9-dioctylfluorene)) |
(PC61BM) | [6,6]-Phenyl-C61-butyric acid methyl ester |
(EDOT) | 3,4-Ethylenedioxythiophene |
(PEDOT) | Poly(3,4-ethylenedioxythiophene) |
(PFs) | Polyfluorenes |
(D) | Donor |
(A) | Acceptor |
(TBAB) | Tetrabutyl ammonium bromide |
(DMAc) | Dimethyl acetamide |
(DMSO) | Dimethyl sulphoxide |
(DCM) | Dicholoromethane |
(THF) | Tetrahydrofuran |
(MeOH) | Methanol (anhydrous) |
(1H NMR) | 1H Nuclear magnetic resonance |
(FT-IR) | Fourier transform infra-red |
(XPS) | X-ray photoelectron spectroscopy |
(GPC) | Gel permeation chromatography |
(UV-visible) | Ultraviolet-visible |
(DSC) | Differential scanning calorimetry |
(DFT) | Density functional theory |
(B3LYP) | Becke, three parameter, Lee–Yang–Parr |
(HSE06) | Heyd–Scuseria–Ernzerhof |
(LSDA) | Local spin density approximation |
(PBC) | Periodic boundary condition |
(ITO) | Indium tin oxide |
(PTFE) | Polytetrafluoroethylene |
(Mn) | Number average molecular weight |
(Mw) | Weight average molecular weight |
(FL) | Fluorene |
(P(FL)) | Poly(dioctyl fluorene) |
(Eoptg) | Optical band gap |
(HOMO) | Highest occupied molecular orbital |
(LUMO) | Lowest unoccupied molecular orbital |
(CV) | Cyclic voltammetry |
(DPV) | Differential pulse voltammetry |
(PL) | Photoluminescence spectrum |
(Eg) | Band gap |
(DC) | Direct current |
(CT) | Charge transfer |
(ID) | The steady state current values, prior to illumination |
(IL) | The steady state current, after illumination |
(Iph) | Photosensitivity |
(σ) | Photoconductivity |
(I) | Light intensity |
(P) | Laser power |
(d) | Thickness |
(V) | Applied voltage |
(PVK) | Poly(N-vinylcarbazole) |
(TNF) | 2,4,7-Trinitro-9-fluorenone and |
(DANS) | 4-N,N-Dimethyl-4′-nitrostilbene |
Acknowledgements
The authors gratefully acknowledge financial support from University Grants Commission Research Fellowships in Sciences for Meritorious Students (UGC-RFSMS), Department of Science and Technology (DST) and Council of Scientific and Industrial research (CSIR). Also, we thank Dr Aby C. P. for characterization of the polymer sample.
References
-
(a) P. Dutta, H. Park, W. H. Lee, I. N. Kang and S. H. Lee, Polym. Chem., 2014, 5, 132–143 RSC;
(b) C.-W. Ge, C.-Y. Mei, J. Ling, J.-T. Wang, F.-G. Zhao, L. Liang, H.-J. Li, Y.-S. Xie and W.-S. Li, J. Polym. Sci., Part A: Polym. Chem., 2014, 52, 2356–2366 CrossRef CAS;
(c) W. Zhuang, M. Bolognesi, M. Seri, P. Henriksson, D. Gedefaw, R. Kroon, M. Jarvid, A. Lundin, E. Wang, M. Muccini and M. R. Andersson, Macromolecules, 2013, 46, 8488–8499 CrossRef CAS;
(d) J. Y. Ma, H.-J. Yun, S.-O. Kim, G. B. Lee, H. Cha, C. E. Park, S.-K. Kwon and Y.-H. Kim, J. Polym. Sci., Part A: Polym. Chem., 2014, 52, 1306–1314 CrossRef CAS.
-
(a) A. Kraft, A. C. Grimsdale and A. B. Holmes, Angew. Chem., Int. Ed., 1998, 37, 402–428 CrossRef;
(b) N. R. Evans, L. S. Devi, C. S. K. Mak, S. E. Watkins, S. I. Pascu, A. Kohler, R. H. Friend, C. K. Williams and A. B. Holmes, J. Am. Chem. Soc., 2006, 128, 6647–6656 CrossRef CAS PubMed;
(c) Q. Huang, G. A. Evmenenko, P. Dutta, P. Lee, N. R. Armstrong and T. J. Marks, J. Am. Chem. Soc., 2005, 127, 10227–10242 CrossRef CAS PubMed.
-
(a) H. H. Fong, V. A. Pozdin, A. Amassian, G. G. Malliaras, D. M. Smilgies, M. He, S. Gasper, F. Zhang and M. Sorensen, J. Am. Chem. Soc., 2008, 130, 13202–13203 CrossRef CAS PubMed;
(b) M. H. Hoang, M. J. Cho, D. C. Kim, K. H. Kim, J. W. Shin, M. Y. Cho, J. Joo and D. H. Choi, Org. Electron., 2009, 10, 607–617 CrossRef CAS PubMed;
(c) P. M. Beaujuge, W. Pisula, H. N. Tsao, S. Ellinger, K. Mullen and J. R. Reynolds, J. Am. Chem. Soc., 2009, 131, 7514–7515 CrossRef CAS PubMed;
(d) S. Pang, H. N. Tsao, X. Feng and K. Mullen, Adv. Mater., 2009, 21, 3488–3491 CrossRef CAS;
(e) H. H. Chang, C. E. Tsai, Y. Y. Lai, W. W. Liang, S. L. Hsu, C. S. Hsu and Y. J. Cheng, Macromolecules, 2013, 46, 7715–7726 CrossRef CAS.
- D. T. McQuade, A. E. Pullen and T. M. Swager, Chem. Rev., 2000, 100, 2537–2574 CrossRef CAS PubMed.
-
(a) P. M. Beaujuge, C. M. Amb and J. R. Reynolds, Acc. Chem. Res., 2010, 43, 1396–1407 CrossRef CAS PubMed;
(b) P. M. Beaujuge and J. R. Reynolds, Chem. Rev., 2010, 110, 268–320 CrossRef CAS PubMed;
(c) P. Shi, C. M. Amb, A. L. Dyer and J. R. Reynolds, Appl. Mater. Interfaces, 2012, 4, 6512–6521 CrossRef CAS PubMed;
(d) E. Kaya, A. Balan, D. Baran and A. Cirpan, Org. Electron., 2011, 12, 202–209 CrossRef CAS PubMed.
- P. Leriche, P. Frère, A. Cravino, O. Aleveque and J. Roncali, J. Org. Chem., 2007, 72, 8332–8336 CrossRef CAS PubMed.
- M. Melucci, P. Frère, M. Allain, E. Levillain, G. Barbarella and J. Roncali, Tetrahedron, 2007, 63, 9774–9783 CrossRef CAS PubMed.
- J. Y. Balandier, F. Quist, C. Amato, S. Bouzakraoui, J. Cornil, S. Sergeyev and Y. Geerts, Tetrahedron, 2010, 66, 9560–9572 CrossRef CAS PubMed.
- L. B. Groenendaal, F. Jonas, D. Freitag, H. Pielartzik and J. R. Reynolds, Adv. Mater., 2000, 12, 481–494 CrossRef CAS.
- L. B. Groenedaal, G. Zotti, P. H. Aubert, S. M. Waybright and J. R. Reynolds, Adv. Mater., 2003, 15, 855–879 CrossRef.
- J. Roncali, P. Blanchard and P. Frere, J. Mater. Chem., 2005, 15, 1589–1610 RSC.
- C. B. Nielsen, A. Angerhofer, K. A. Abboud and J. R. Reynolds, J. Am. Chem. Soc., 2008, 130, 9734–9746 CrossRef CAS PubMed.
- I. Schwendeman, C. L. Gaupp, J. M. Hancock, L. Groenendaal and J. R. Reynolds, Adv. Funct. Mater., 2003, 13, 541–547 CrossRef CAS.
- K. Krishnamoorthy, R. S. Gokhale, A. Q. Contractor and A. Kumar, Chem. Commun., 2004, 820–821 RSC.
- R. B. Bazaco, R. Gomez, C. Seoane, P. Baeuerle and J. L. Segura, Tetrahedron Lett., 2009, 50, 4154–4157 CrossRef CAS PubMed.
- B. Winther-Jensen, O. Winther-Jensen, M. Forsyth and D. Macfarlane, Science, 2008, 321, 671–674 CrossRef CAS PubMed.
- F. I. Wu, P. I. Shih, C. F. Shu, Y. L. Tung and Y. Chi, Macromolecules, 2005, 38, 9028–9036 CrossRef CAS.
- Y. Yang and Q. Pei, J. Appl. Phys., 1997, 81, 3294–3298 CrossRef CAS PubMed.
- B. Liu, W. L. Yu, Y. H. Lai and W. Huang, Chem. Mater., 2001, 13, 1984–1991 CrossRef CAS.
- O. Stephan, F. Tran-Van and C. Chevrot, Synth. Met., 2002, 131, 31–40 CrossRef CAS.
- L. Akcelrud, Prog. Polym. Sci., 2003, 28, 875–962 CrossRef CAS.
- W. C. Wu, C. L. Liu and W. C. Chen, Polymer, 2006, 47, 527–538 CrossRef CAS PubMed.
- E. Bundgaard and F. C. Krebs, Sol. Energy Mater. Sol. Cells, 2007, 91, 954–985 CrossRef CAS PubMed.
- A. Cuendias, M. Urien, S. Lecommandoux, G. Wantz, E. Cloutet and H. Cramail, Org. Electron., 2006, 7, 576–585 CrossRef PubMed.
- C. B. Bezgin, A. Kivrak and M. A. Onal, Electrochim. Acta, 2011, 58, 223–230 CrossRef PubMed.
- S. M. Cassemiro, C. Zanlorenzi, T. D. Z. Atvars, G. Santos, F. J. Fonseca and L. Akcelrud, J. Lumin., 2013, 134, 670–677 CrossRef CAS PubMed.
- P. Blondin, J. Bouchard, S. Beaupr, M. Bellete, G. Durocher and M. Leclerc, Macromolecules, 2000, 33, 5874–5879 CrossRef CAS.
- P. H. Aubert, M. Knipper, L. Groenendaal, L. Lutsen, J. Manca and D. Vanderzande, Macromolecules, 2004, 37, 4087–4098 CrossRef CAS.
- B. Bezgin, A. Yagan and A. M. Onal, J. Electroanal. Chem., 2009, 632, 143–148 CrossRef CAS PubMed.
- B. Bezgin and A. M. Onal, Electrochim. Acta, 2010, 55, 779–784 CrossRef CAS PubMed.
- G. Nie, H. Yang, J. Chen and Z. Bai, Org. Electron., 2012, 13, 2167–2176 CrossRef CAS PubMed.
- A. Kumar and A. Kumar, Polym. Chem., 2010, 1, 286–288 RSC.
- K. Yamazaki, J. Kuwabara and T. Kanbara, Macromol. Rapid Commun., 2013, 34, 69–73 CrossRef CAS PubMed.
- J. Kuwabara, T. Yasuda, S. J. Choi, W. Lu, K. Yamazaki, S. Kagaya, L. Han and T. Kanbara, Adv. Funct. Mater., 2014, 24, 3226–3233 CrossRef CAS.
- Z. B. Liu, Y. F. Xu, X. Y. Zhang, X. L. Zhang, Y. S. Chen and J. G. Tian, J. Phys. Chem. B., 2009, 113, 9681–9686 CrossRef CAS PubMed.
- K. Yoneda, M. Nakano, R. Kishi, H. Takahashi, A. Shimizu, T. Kubo, K. Kamada, K. Ohta, B. Champagne and E. Botek, Chem. Phys. Lett., 2009, 480, 278–283 CrossRef CAS PubMed.
- E. Hendry, P. Hale, J. Moger, A. Savchenko and S. A. Mikhailov, Phys. Rev. Lett., 2010, 105, 97401–97404 CrossRef CAS.
- L. Kamath, K. B. Manjunatha, S. Shettigar, G. Umesh, B. Narayana, S. Samshuddin and B. K. Sarojini, Opt. Laser Technol., 2014, 56, 425–429 CrossRef CAS PubMed.
- R. G. Parr and W. Yang, Density-Functional Theory of Atoms and Molecules, Oxford University Press, New York, 1989 Search PubMed.
- A. D. Becke, J. Chem. Phys., 1993, 98, 5648–5652 CrossRef CAS PubMed.
- C. Lee, W. Yang and R. G. Parr, Phys. Rev. B: Condens. Matter Mater. Phys., 1994, 37, 785–789 CrossRef.
- K. Burke, J. P. Perdew, Y. Wang, J. F. Dobson, G. Vignale and M. P. Das, Electronic Density Functional Theory: Recent Progress and New Directions, Plenum Press, New York, 1998 Search PubMed.
- J. Heyd, G. E. Scuseria and M. Ernzerhof, J. Chem. Phys., 2003, 118, 8207–8215 CrossRef CAS PubMed.
- A. V. Krukau, O. A. Vydrov, A. F. Izmaylov and G. E. Scuseria, J. Chem. Phys., 2006, 125, 224106–224110 CrossRef PubMed.
-
(a) S. H. Vosko, L. Wilk and M. Nusair, Can. J. Phys., 1980, 58, 1200–1211 CrossRef CAS PubMed;
(b) U. Salzner, J. B. Lagowski, P. G. Pickup and R. A. Poirier, J. Comput. Chem., 1997, 18, 1943–1953 CrossRef CAS.
- M. J. Frisch, G. W. Trucks, H. B. Schlegel, G. E. Scuseria, M. A. Robb, J. R. Cheeseman, G. Scalmani, V. Barone, B. Mennucci, G. A. Petersson, H. Nakatsuji, M. Caricato, X. Li, H. P. Hratchian, A. F. Izmaylov, J. Bloino, G. Zheng, J. L. Sonnenberg, M. Hada, M. Ehara, K. Toyota, R. Fukuda, J. Hasegawa, M. Ishida, T. Nakajima, Y. Honda, O. Kitao, H. Nakai, T. Vreven Jr. J. A. Montgomery, J. E. Peralta, F. Ogliaro, M. Bearpark, J. J. Heyd, E. Brothers, K. N. Kudin, V. N. Staroverov, R. Kobayashi, J. Normand, K. Raghavachari, A. Rendell, J. C. Burant, S. S. Iyengar, J. Tomasi, M. Cossi, N. Rega, N. J. Millam, M. Klene, J. E. Knox, J. B. Cross, V. Bakken, C. Adamo, J. Jaramillo, R. Gomperts, R. E. Stratmann, O. Yazyev, A. J. Austin, R. Cammi, C. Pomelli, J. W. Ochterski, R. L. Martin, K. Morokuma, V. G. Zakrzewski, G. A. Voth, P. Salvador, J. J. Dannenberg, S. Dapprich, A. D. Daniels, Ö. Farkas, J. B. Foresman, J. V. Ortiz, J. Cioslowski and D. J. Fox, Gaussian 09, Revision B02, Gaussian, Inc., Wallingford, CT, 2009 Search PubMed.
- M. Sheik-bahae, A. A. Said, T.-H. Wei, D. J. Hagan and E. W. Van Stryland, IEEE J. Quantum Electron., 1990, 26, 760–769 CrossRef CAS.
- S. G. Im, K. K. Gleason and E. A. Olivetti, Appl. Phys. Lett., 2007, 90, 152112–152114 CrossRef PubMed.
- C. Kvarnstrom, H. Neugebauer, S. Blomquist, H. J. Ahonen, J. Kankare and A. Ivaska, Electrochim. Acta, 1999, 44, 2739–2750 CrossRef CAS.
- A. Yildirima, S. Tarkuc, M. Ak and L. Toppare, Electrochim. Acta, 2008, 53, 4875–4882 CrossRef PubMed.
- G. Nie, H. Yang, J. Chen and Z. Bai, Org. Electron., 2012, 13, 2167–2176 CrossRef CAS PubMed.
- D. Bhattacharyya and K. K. Gleason, Chem. Mater., 2011, 23, 2600–2605 CrossRef CAS.
-
(a) P. Feibelman, Phys. Rev. B: Condens. Matter Mater. Phys., 1987, 35, 2626–2646 CrossRef CAS;
(b) J. E. Jaffe and A. C. Hess, J. Chem. Phys., 1996, 105, 10983–10998 CrossRef CAS PubMed;
(c) S. Hirata and S. Iwata, J. Chem. Phys., 1997, 107, 10075–10084 CrossRef CAS PubMed;
(d) J. Q. Sun and R. J. Bartlett, J. Chem. Phys., 1998, 109, 4209–4223 CrossRef CAS PubMed.
- M. Hiramoto, H. Fujiwara and M. Yokoyama, J. Appl. Phys., 1992, 72, 3781–3787 CrossRef CAS PubMed.
- T. Taima, M. Chikamatsu, Y. Yoshida, K. Saito and K. Yase, Appl. Phys. Lett., 2004, 85, 6412–6414 CrossRef CAS PubMed.
- V. I. Arkhipov, H. BÄassler, M. Deussen, E. O. GÄobel, R. Kersting, H. Kurz, U. Lemmer and R. F. Mahrt, Phys. Rev. B: Condens. Matter Mater. Phys., 1995, 52, 4932–4940 CrossRef CAS.
- V. I. Arkhipov and H. BÄassler, Phys. Stat. Sol., 2004, 201, 1152–1187 CrossRef CAS.
- N. A. Davidenko, M. A. Zabolotny, A. A. Ishchenko, N. G. Kuvshinskii and N. P. Borolina, High Energ. Chem., 2004, 38, 13–19 CrossRef CAS.
- L. Onsagar, Phys. Rev., 1938, 54, 554–557 CrossRef.
- V. C. Kishore, Ph.D. thesis, Cochin University of Science and Technology, India, 2008.
- D. Shuo-xing, Z. Jia-zen, Y. Pei-xian, H. Hai-ping and Y. Cheng, Acta Phys. Sin., 1995, 4, 663–669 Search PubMed.
- A. Thankappan, S. Thomas and V. P. N. Nampoori, Opt. Laser Technol., 2014, 58, 63–70 CrossRef CAS PubMed.
- G. S. He, G. C. Xu, P. N. Prasad, B. A. Reinhardt, J. C. Bhatt and A. G. Dillard, Opt. Lett., 1995, 20, 35–37 Search PubMed.
- N. K. M. N. Srinivas, S. V. Rao and D. N. Rao, J. Opt. Soc. Am. B, 2003, 20, 2470–2479 CrossRef CAS.
- R. L. Sutherland, Handbook of nonlinear optics, Dekker, New York, 1996 Search PubMed.
Footnote |
† Electronic supplementary information (ESI) available. See DOI: 10.1039/c4ra13024c |
|
This journal is © The Royal Society of Chemistry 2015 |