DOI:
10.1039/C4RA12851F
(Paper)
RSC Adv., 2015,
5, 5657-5663
Resveratrol improves fungal ribosylation capacity through a unique mechanism†
Received
21st October 2014
, Accepted 4th December 2014
First published on 5th December 2014
Abstract
Ribosylation is a significant modification conferring new complex and broadened cellular roles of compounds, but small organic molecules are rarely ribosylated. Here, we report the 3-O- and 4′-O-α-ribosylation of resveratrol, a phytoalexin that is exogenous to the fungus Daldinia eschscholzii IFB-TL01. The ribosylation is mechanistically due to the resveratrol-activated expression of the silent or less active fungal genes governing the ribosylation of non-fungal phytophenols. The resveratrol-induced ribosylation is also characterized by an increased expression of ribosyltransferases in concert with a rare ribosylating reaction using nicotinamide mononucleotide (NMN) as a ribose donor. The ribosylation-reduced toxicity of resveratrol to D. eschscholzii, along with the involvement of at least two p-glycoproteins in the glycosylation, suggests that such a glycosylation process may be a general strategy for the fungal detoxification of phenolic chemicals. The findings present an updated view of the ribosylation of small molecules, and provide direct chemogenetic evidence that will aid understanding of how phytophenols such as resveratrol function differently in the plant, microbial and animal kingdoms.
Introduction
Ribosylation plays key roles in a variety of biological processes, such as gene regulation, actin-clustering, defects in phagocytosis and cell death.1 Some functional proteins tend to be readily ribosylated in response to damaging factors, such as bacterial toxin exposure, and in this case covalent modifications lead to enzyme dysfunction owing to the incorporation of a ribose motif derived from nicotinamide adenine dinucleotide (NAD+).2 However, the ribosylation of small molecule organic compounds including natural products (secondary metabolites) is rarely detected in nature,3 and little information has been documented concerning the mechanism of the ribosylation of natural molecules. In contrast, glycosylation and xylosylation have been found to decorate an array of natural products, such terpenes, coumarins, flavonoids and macrolides, through the glycosyltransferase-catalyzed nucleophilic attack of nucleoside diphosphate (NDP)-sugars.4 Distinct from the widely occurring glycosylation, the mechanism underlying the ribosylation of small organic molecules remains largely elusive.
Daldinia eschscholzii IFB-TL01, a fungus belonging to the saprophytic and endophytic Xylariaceae family,5 is a versatile producer of phenolic polyketides.6 However, our continuous efforts have failed to detect any glycosylated metabolites from fungal cultivations accomplished under several laboratory cultivation conditions. Surprisingly, this observation disagrees with the presence of glycosyltransferase genes in the fungal genome.6 In light of the genomic plasticity,7 these genes are not evolutional excrescences, but their activation may depend on a particular combination of external factors such as nitrogen, carbon, pH and temperature.8 Resveratrol (3,5,4′-trihydroxystilbene, Fig. 1) is a phenolic phytochemical that accumulates in many plants, such as peanuts, grapes, blueberries, mulberries and cranberries.9 The natural phenol has been found to interfere with a variety of signaling pathways in mammal cells to exhibit anti-carcinogenic, anti-aging, anti-inflammatory, anti-diabetic and antioxidant actions.10 The study of the metabolic fate of resveratrol has determined its ability to be glycosylated into glucuronides in human cells,11 glucopyranosides in plant cells,12 or diglucosides by cyclodextrin glucanotransferase and sucrose phosphorylase from Thermoanaerobacter sp.13 In addition, resveratrol functions as a phytoalexin in response to microbial stresses.14 The engineering of resveratrol synthase-encoding genes in heterogenous plants such as Solanum lycopersicum and Oryza sativa can increase their resistance against fungal pathogens.15 These observations, along with the reported fungal glycosylation of stilbenoids,16 prompted us to use resveratrol as a xenochemical tool to investigate the glycosylating capacity of D. eschscholzii with an intention to obtain unprecedented fungal glycosides. Here, resveratrol is demonstrated to increase the ribosylation capacity of D. eschscholzii through the activation (or up-regulation) of silent (or less active) ribosyltransferase and p-glycoprotein genes, and the rare fungal ribosylation takes place uniquely through an undescribed mechanism using nicotinamide mononucleotide (NMN) as the ribosyl donor. As detailed below, the work reveals a conventionally undetectable ribosylation process that provides direct evidence for the fungal detoxification of phenolic chemicals and access to new ribosides with biological and/or biomedical significance.
Results and discussion
Ribosylation of resveratrol and fungal phenols in D. eschscholzii culture
To study the xenobiotic response of D. eschscholzii to resveratrol, the fungus was grown for seven days, and the afforded healthy mycelia were subsequently inoculated into media supplemented with resveratrol at varying concentrations. As expected, the fungal growth in Petri dishes was substantially attenuated in a dose-dependent manner by its exposure to resveratrol at 10, 25, 50 and 100 mg L−1 (Fig. 1A(a) and (b)), implying that this strain was sensitive to resveratrol. Examination of the strain in liquid culture exposed to resveratrol showed that the colour of the fermentation extracts turned to red, from the black of the control (free of resveratrol, Fig. 1A(c)), showing an induced change resulting from the resveratrol supplementation in the D. eschscholzii culture.
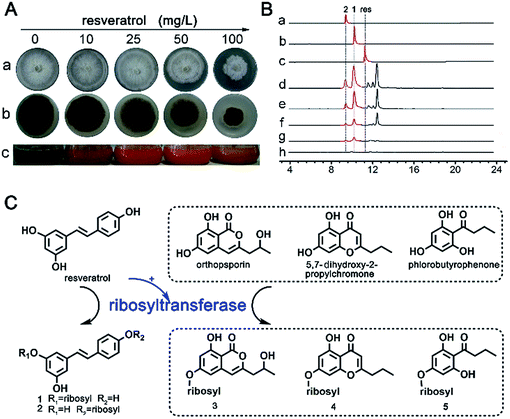 |
| Fig. 1 Ribosylation of resveratrol by D. eschscholzii. (A) Dose-dependent changes in the phenotype of the fungal strain when exposed to resveratrol. The top (a) and bottom (b) views of Petri dishes and liquid cultivations (c) kept at 28 °C for 7 days. (B) LC-MS chromatograms of extracts derived from liquid fungal cultures with resveratrol (res) supplemented at 100 (d), 50 (e), 25 (f), 10 (g) and 0 mg L−1 (h), using an extracted ion at m/z 383.11 arising from e.g. the [M + Na]+ ion of 3- and 4′-O-α-D-ribosyl-resveratrol (1 and 2). (C) Ribosyltransferase gene activation by resveratrol enabled/up-regulated ribosylations of orthosporin, 5,7-dihydroxy-2-propylchromone and phlorobutyrophenone; these fungal products failed to be detected as glycosides in our previous laboratory culture of the fungus. | |
To assess the alteration of fungal metabolites in the xenochemical-exposed cultures, extracts derived from the culture broths at varied resveratrol concentrations were separately collected and analyzed by LC-UV-MS (Fig. 1B). Encouragingly, a new peak on the chromatograph, corresponding to a UV curve resembling that of resveratrol, was found, and gave a quasimolecular ion at m/z 383.1106 requiring an elemental composition of C19H20O7Na (calcd weight, 383.1101). To elucidate the structure of the “newly appeared” metabolite, the resveratrol-supplemented fermentation was scaled up, and the afforded extract was fractionated by column chromatographies over silica gel and Sephadex LH-20, followed if necessary by semi-preparative HPLC, to yield a new metabolite, 3-O-α-D-ribosyl-resveratrol (1, Fig. 1B), whose structure was elucidated by subsequent interpretation of its MS, UV and NMR spectra (1H and 13C NMR, 1H–1H COSY, NOESY, HSQC and HMBC) as detailed in ESI.†
Resveratrol possesses 3-, 5- and 4′-hydroxy groups, the former two being chemically equivalent. The characterization of 3-O-α-D-ribosyl-resveratrol indicated the possible co-generation of its 4′-O-ribosylated counterpart. To address this probability, further scaled-up growth of the fungal strain was performed, followed by LC-MS tracing analysis for the presence of the proposed 4′-O-ribosyl derivative of resveratrol. As expected, 4′-O-α-D-ribosyl-resveratrol (2) was characterized as another ribosylated metabolite of resveratrol, as carried out for 1 (see above and ESI†). Further ribosylation of 1 or 2 was negligible, since no di- and tri-ribosyl derivatives of resveratrol were detected in the original extract or in the mother liquor resulting from the fractionation of the two resveratrol ribosides.
Prior to this work, no glycosidic metabolite could be isolated during our continuous chemical investigation on D. eschscholzii, which is capable of producing non-glycosidic phenolic polyketides.6 Surprisingly, in the fermentation of the strain with resveratrol at 50 mg L−1, more novel ribosylated phenols, 6-O-α-D-ribosyl-orthosporin (3, C17H20O9), 7-O-α-D-ribosyl-5-hydroxy-2-propylchromone (4, C17H20O8) and 4-O-α-D-ribosyl-phlorobutyrophenone (5, C15H20O8), were detected by LC-MS, and were further purified by semi-preparative HPLC (Fig. 1C). The aglycone motifs of the ribosides are derived from orthosporin, 5,7-dihydroxy-2-propylchromone and phlorobutyrophenone, which are all fungal metabolites.6 Thus, resveratrol can facilitate the in vivo ribosylation of some phenolic metabolites of the strain. In conclusion, these findings suggest that the genes responsible for ribosylation in D. eschscholzii are likely silent or poorly activated, but might be activated by resveratrol exposure to lead to the regioselective mono-ribosylation of resveratrol itself and some phenolic metabolites of the fungus.
Mechanism of the fungal ribosylation
Glycotransferases catalyse the endogenous glycosylation of small molecule substrates using sugar nucleotides as glycosyl donors.4 The genome of D. eschscholzii (BioProject ID: PRJNA157267) contains as many as sixteen ribosylation-related genes potentially governing the expression of six ADP-ribosyltransferases (AT) and ten phosphoribosyltransferases (PT). Thus, a ribosyl transfer mechanism could be proposed for the fungal ribosylation. To confirm this assumption, we performed a resveratrol-exposed fungal cultivation, supplemented simultaneously with sodium dodecyl sulfate (SDS), a glycosyltransferase inhibitor16 that was found in this study to negligibly affect the growth of D. eschscholzii. As anticipated, resveratrol 3-O- and 4′-O-α-D-ribosides 1 and 2 became undetectable by the LC-UV-MS analysis of the fungal culture exposed to SDS at 0.5 mM (Fig. 2). This reinforced the idea that the ribosyltransferases might have contributed to the resveratrol ribosylation, and as ascertained above, their encoding genes might be conventionally inactive.
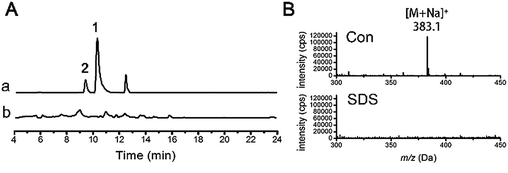 |
| Fig. 2 LC-MS assessment of resveratrol ribosylation in the absence (a) and presence (b) of the ribosyltransferase inhibitor SDS at 0.5 mM. Traces were monitored at EIC (extracted ion chromatogram) = m/z 383.1 (A) and ESI-MS at 10 min (B). Con = control. | |
To clarify the regulatory effect of resveratrol on the ribosyl-transferase gene expression of the fungus, RNAs isolated from the five-day cultures with (50 mg L−1) and without resveratrol were reverse-transcribed to obtain the corresponding cDNAs, with the mRNA transcriptional level of each ribosyltransferase assessed using a real-time PCR approach (Fig. 3). Among the sixteen ribosylation-related genes recognized from the genomic analysis, the expression of one AT- and five PT-encoding genes increased by at least 5-fold in the presence of resveratrol compared with that of the untreated group. However, the other five AT-encoding genes were negligibly affected by the addition of resveratrol. This suggests that resveratrol can increase the expression level of endogenous ribosyltransferases, with phosphoribosyl-transferases being more sensitive to resveratrol than ADP-ribosyltransferases. Therefore, the ribosylation of resveratrol was most probably catalyzed by a phosphoribosyltransferase in D. eschscholzii, although the particular enzyme failed to be identified through the gene knock-out approach because of the inevitable interference from compensatory ribosylation catalyzed by undeprivable isoenzymes.
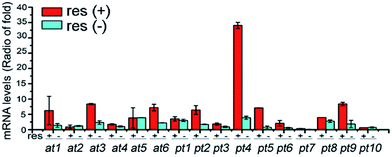 |
| Fig. 3 qRT-PCR comparison of the transcriptional levels of ADP-ribosyltransferase (at1–at6) and phosphoribosyltransferase (pt1–pt10) genes in D. eschscholzii with [res(+), 50 mg L−1] or without [res(−)] resveratrol supplementation. | |
Glycosyl donor for resveratrol ribosylation
We were curious about the glycosyl origin of the resveratrol ribosides, which is also of value in understanding the mechanism of the biological function of resveratrol. Exogenous D-ribose from culture or carbohydrate transformations17 was first presumed to be the glycosyl donor for the resveratrol ribosylation. However, resveratrol failed to be ribosylated upon its co-incubation with D-ribose (150 mg L−1) in the presence of laccase (10 U) prepared from the fungus as previously described.18 We were subsequently motivated to test the possible ribosylation by primary metabolic intermediates such as the ribosyl-containing NDP-sugars, nucleotides and coenzymes (e.g., NAD(H)s), both being active ribosylating agents.19 In fresh fungal homogenates (ultrasonicated thoroughly to liberate endogenous enzymes), resveratrol (2 mM) was allowed to react independently with four potential ribosyl donors, UDP, NAD+, AMP and ADP. The LC-UV-MS monitoring demonstrated that ribosides 1 and 2 were formed after incubation with NAD+ at 28 °C (Fig. 4). However, no resveratrol riboside could be detected in the reaction mixture even after 24 hour incubation with UDP, AMP and ADP. In view that NAD+ is relatively well known to ADP-ribosylate, but not monoribosylate, it should not be proposed as the direct donor for the resveratrol ribosylation. Further study showed that nicotinamide mononucleotide (NMN), a key intermediate produced in the NAD+ biosynthetic pathway, could prominently increase the yields of 1 and 2 under the same conditions as tested with NAD+, and the production of 2 was much higher than with NAD+-treatment (Fig. 4). Thus, in D. eschscholzii, NMN functions as an effective ribosyl donor for phenol ribosylations, which is catalyzed by phosphoribosyltransferase, whose expression can be substantially activated by resveratrol exposure. Moreover, NAD+ is probably cleaved by nucleotide pyrophosphatases to produce NMN for the ribosylation.20
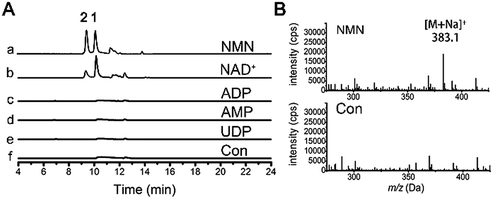 |
| Fig. 4 The ribosyl donor for resveratrol glycosylation. LC-MS monitoring of treatment of fungal homogenate with resveratrol, without (f) and with addition of NMN (a), NAD+ (b), ADP (c), AMP (d) and UDP (e). Traces were monitored at EIC = m/z 383.1 (A) and ESI-MS at 10 min (B). Con = control. | |
Previous investigations have demonstrated that the ribosylation of peptides is generally associated with ADP-ribosyltransferases using NAD+ as the source of ribose, and glycosylation of small molecule aglycones occurs by using NDP-sugars as donors. Distinct from this observation, our results suggest a novel mechanism for the phosphoribosyltransferase-catalyzed ribosylation of small organic molecules, with NMN as the ribose donor.
Ribosylation as a detoxifying mechanism of D. eschscholzii upon exposure to resveratrol
The nematode Caenorhabditis elegans has been reported to detoxify alkaloidal and phenolic toxins through glucosylation.21 The glucosylation-based detoxification mechanism is also adopted by the bacterial pathogen Helicobacter pylori for the deactivation of 7-dehydrocholesterol,22 by the phytopathogenic fungus Sclerotinia sclerotiorum for metabolizing the cruciferous phytoalexins, camalexin, brassinin, cyclobrassinin and brassilexin,23 and by plants, such as Nicotiana tabacum for naphthol inactivation24 and Arabidopsis thaliana for detoxifying xenobiotic chemicals including benzoxazolin-2(3H)-one25 and 3,4-dichloroaniline.26 Resveratrol is a phytoalexin15 and is toxic to D. eschscholzii (see above). We hypothesized that the ribosylation of resveratrol could contribute to the fungal detoxification of the phytoalexin. Accordingly, resveratrol and its ribosides were tested at 0.5 mM in 96-well plates using the fungus as a target microbe. As hypothesized, the fungal germination that appeared in the plates treated with 1 and 2 was much stronger than that discerned in the resveratrol-treated group (Fig. 5A). Thus, the fungicidal effect of resveratrol to D. eschscholzii is diminished significantly by the fungal ribosylation of the phytoalexin.
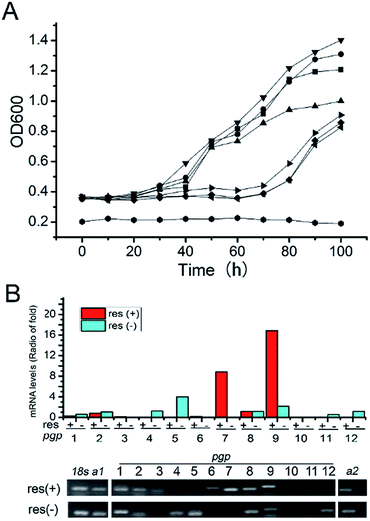 |
| Fig. 5 Detoxification of resveratrol to D. eschscholzii. (A) The susceptibility of D. eschscholzii toward resveratrol and its ribosides. Spores of D. eschscholzii were incubated separately in malt-extract culture at 28 °C with exposure to resveratrol, and ribosides 1 and 2 (0.5 mM). The germination rates of these spores were determined according to the OD600. ▼: wild type strain without addition of any test compound. ▲, ■ and ●: wild type strain supplemented with resveratrol, 1 and 2, respectively. ◆, ◀ and ▶: resveratrol-exposed cultures of Δpgp3, Δpgp6 and Δpgp11 strains, respectively. : the malt-extract medium taken as a control. The data are the mean of triplicates with S.D. ≤ 5%. (B) qRT-PCR comparison of the transcriptional levels and gel electrophoresis for qRT-PCR products of p-glycoprotein genes (pgp1–pgp12) from the strain with [res(+), 50 mg L−1] or without [res(−)] exposure to resveratrol, using 18S rRNA (18s) and actin-encoding genes (a1 and a2) as controls. | |
To gain more information about the detoxification machinery of D. eschscholzii for resveratrol, we scrutinized the genomic information of the fungus, which indicates that the fungus carries a total of forty-three ATP-binding cassette (ABC) transporter genes, with twelve predicted to encode p-glycoproteins (pgps). Previous studies have shown that the ABC transporters are functional proteins capable of transporting secondary metabolites,27 and one of its subfamilies, pgp, is involved in drug resistance in mammals.28 However, the functions of ABC proteins have been largely unknown for fungi, although increased expression of an ABC transporter gene has been discerned in Aspergillus fumigatus.29 Real-time PCR analysis was therefore performed to examine the presumed regulation of pgp genes in D. eschscholzii by resveratrol (50 mg L−1, 5-day exposure), with 18S rRNA and two actin encoding genes allotted as internal references. Three pgp genes, pgp3, pgp6 and pgp7, were substantially up-regulated (Fig. 5B). In particular, these three pgp genes are usually inactive in the intact strain (Fig. 5B), but can be activated by the fungal exposure to resveratrol.
As shown by phylogenetic analysis, these pgp genes are significantly diverse in sequence, and can be classified into three different subfamilies of ABC transporter proteins (Fig. S1†),30 Disruption of the above activated pgp genes was carried out using a modified method of split-PCR (Fig. S2†).31 Toxicity assays showed that upon treatment with resveratrol at 0.5 mM, the D. eschscholzii pgp-3 and pgp-6 mutants were much more susceptible to resveratrol than the wild strain (Fig. 5A). Pgp3 belongs to the full-size superfamily B involved in the oxidative stress response, and is required for host penetration, an initial key step for the microbial colonization of host tissues.32 The pgp3 knockout strain of D. eschscholzii showed reduced production of resveratrol riboside 2, a “detoxification” product of the phytoalexin (Fig. 6). Pgp6, possessing an excessive C-terminal relative to other pgps, is unique, with low (46%) identity to the most similar ABC transporters described to date, and belongs to the superfamily G whose biochemical function remains elusive.25 Thus, pgp6 represents a new-type of ABC transporter. Our data showed that the pgp6 knockout strain of D. eschscholzii was unable to transform resveratrol into ribosides 1 or 2 (Fig. 6). In addition, a gene-deletion experiment as control was carried out with the gene pgp11, a lipid transporter under the superfamily A,33 which showed no transcriptional change between the resveratrol-treated and -untreated strains (Fig. 5B). As expected, the deletion of pgp11 did not affect the generation of ribosides 1 and 2 (Fig. 6). The data collectively suggest that resveratrol can be detoxified by the resistance machinery in the fungus, and the fungal p-glycoproteins pgp3 and pgp6 are involved in the detoxification process.
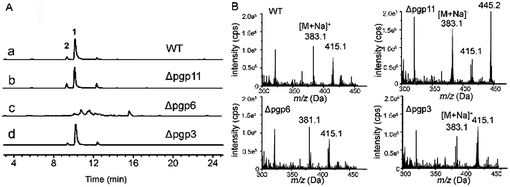 |
| Fig. 6 LC-MS evaluated transformation of resveratrol into 1 and 2 by wild-type (a), and pgp11- (b), pgp6- (c) and pgp3-deleted (d) strains of D. eschscholzii. Traces were monitored at EIC = m/z 383.1 (A) and ESI-MS at 10 min (B). | |
Experimental section
Strain, cultivation, and metabolite fractionation
D. eschscholzii IFB-TL01 has been previously described.6 The fresh mycelium taken from the fungal colony in the Petri dish was inoculated in malt-extract medium (20 g L−1 malt extract, 20 g L−1 sucrose, 1 g L−1 peptone), followed by resveratrol (150 mg L−1)-supplemented cultivation for 7 days at 28 °C and 200 rpm min−1. The broth was extracted with EtOAc, and in vacuo evaporation of the solvent gave a residue which was subjected to column chromatography over silica gel (100 g, 200–300 mesh, 50 × 3 cm) eluted with CH2Cl2–MeOH mixtures (v/v 100
:
0, 100
:
1, 100
:
2, 100
:
4, 100
:
8, 100
:
16, 100
:
32, 0
:
100). Further purification of the “100
:
16” eluate was accomplished using gel filtration over Sephadex LH-20 in MeOH, followed by semi-preparative HPLC (MeOH–H2O, 3
:
7) to give 1 (Rt = 30.9 min) and 2 (Rt = 29.7 min). Purification of the “100
:
4” eluate using Sephadex LH-20 and HPLC (MeOH–H2O, 53
:
47) gave 3 (Rt = 14.0 min). Purification of the “100
:
4” eluate using Sephadex LH-20 and HPLC (MeOH–H2O, 45
:
55) gave 4 (Rt = 20.0 min) and 5 (Rt = 24.0 min).
LC-UV-MS analysis
The filtrates of the fungal fermentation were extracted with EtOAc, and in vacuo evaporation of solvent from the extract gave a residue which was dissolved in MeOH and filtered using a 0.45 μm membrane filter. The LC-MS measurements were performed on an Agilent 1200 series LC system, hyphenated with an Agilent 6210 TOF mass spectrometer (Agilent Technologies, Palo Alto, CA, USA) and with an Agilent ZORBAX Eclipse Plus C18 column (3.5 μm, 100 mm × 4.6 mm), using MeOH as mobile phase.
Glycosyltransferase inhibition by SDS
To test the effect of glycosyltransferase inhibitor on the ribosylation of resveratrol, the fungus strain was grown with resveratrol at 50 mg L−1 to the logarithmic phase (28 °C, 48 h), then SDS at 0.5 mM was added into the culture, which was incubated at 28 °C for another 7 days. The filtrate of the fermentation was extracted with EtOAc, and analyzed by LC-MS.
Reaction of resveratrol with potential ribosyl donors
The D. eschscholzii cells afforded after grown at 28 °C for 7 days in the presence of resveratrol (50 mg L−1) were suspended in Tris–HCl buffer (20 mM, pH 7.5) and ultrasonicated to liberate the enzyme. The presumed sugar donors (UDP, NAD+, NMN, AMP, and ADP) were separately added at 4 mM to test tubes preloaded with 2 mL of the enzyme mixture, containing resveratrol at 2 mM, followed by incubation at 28 °C. The filtrates of the reaction mixtures taken from the tubes were analyzed by LC-MS to monitor the production of resveratrol ribosides 1 and 2. Equivalently-processed enzyme mixture, without exposure to resveratrol or any saccharide donor, was taken as a control.
Real-time PCR analysis
The total RNA of the fungus, with or without treatment with resveratrol for 4 days, was isolated using RNAiso Plus (Takara). cDNA was generated from equal amounts of RNAs of the assayed strains using a PrimeScript II 1st Strand cDNA Synthesis Kit (Takara). Quantitative real-time PCR (qRT-PCR) was performed with a One Step SYBR PrimeScript RT-PCR Kit (Takara) using a C1000 Thermal Cycler (Bio-Rad) and quantified using a CFX96 Real-Time System (Bio-Rad). The primers used for qRT-PCR are tabulated in the ESI.† The expression of assayed genes was normalized to the endogenous 18S rRNA gene and actin-encoding genes for variation in RNA quantity and quality.
Susceptibility test
A modified broth-based method was used to examine the growth rate of D. eschscholzii and its mutants. Strains were grown on PDA plates for 10 days, the spores were collected and were diluted to a starting inoculum of 1 × 104 CFU mL−1 (OD600 = 0.4) in malt-extract medium. They were further incubated with resveratrol or its ribosides at 0.5 mM in 96-well microdilution plates (200 μL) and were cultivated at 28 °C for 0–100 h. The germination rates of the stains were determined from the OD600.
Knockout of pgp genes
The fungus was grown on potato dextrose agar plates (200 g L−1 boiled potato, 20 g L−1 sucrose, 15 g L−1 agar) for 10 days at 28 °C. The spores were collected and treated with 10 mg L−1 glucanex in order to harvest the protoplasts. To knockout a pgp gene of interest, two split-marker cassettes were constructed. Each cassette contains sequences flanking the target gene (pgpU for upstream sequence and pgpD for downstream one) fused to an incomplete gene fragment of hygromycin as a selection marker. Both of the cassettes were electroporated (1.4 kV, 25 mF, 800 Ω) into the above protoplasts. Mutated colonies were selected in PDA medium containing hygromycin (250 μg mL−1), and identified using diagnostic PCR. Primers used in this work are listed in the ESI.†
Accession codes
The nucleotide sequences of pt4, pgp3, pgp6, pgp7 and pgp11 have been deposited at the GenBank under accession numbers KJ462507, KF030722, KF030723, KF030724 and KF030725.
Conclusions
In summary, resveratrol up-regulates the expression of the ribosylation-related genes of D. eschscholzii, and the activated ribosyltransferase gene expressions enable the ribosylation of phytophenol and four fungal phenols. The resveratrol ribosylation requires the sugar donor nicotinamide mononucleotide (NMN) which is likely attacked by the resveratrol oxygen atoms on a nucleophilic substitution basis to generate the α-configuration of the anomeric carbon. Biochemically, at least two p-glycoproteins associated with drug resistance are involved in the resveratrol ribosylation. A further biological implication lies in the substantial toxicity reduction of resveratrol to D. eschscholzii, suggesting a general ribosylation-based detoxification mechanism concerning the fungal endurance to the phytoalexin. Providing access towards a new phenolic riboside library, this work adds direct chemogenetic evidence to aid understanding of the molecular mechanism of resveratrol, which is active in a broad range of prokaryotic and eukaryotic cells. The investigation also suggests that the silent or less active fungal genes may be a “reserved arsenal”, ready to cope with challenges and hostilities. The research methods in this study are significant to the basic and applied sciences of natural product drug discovery and promote the development of research on the technology of interdisciplinary subjects. Additionally, with the discovery and modification of new drug molecules, it paves a new way for drug discovery.
Acknowledgements
This work was co-financed by the NSFC (91413120, 21132004, 81421091 & 21302091) and MOST grants (2013AA092901), in addition to support from the Natural Science Foundation of Jiangsu Province (BK2012308) and State Key Laboratory of Bioreactor Engineering.
References
- C. Gatsogiannis, A. E. Lang, D. Meusch, V. Pfaumann, O. Hofnagel, R. Benz, K. Aktories and S. Raunser, Nature, 2013, 495, 520–523 CrossRef CAS PubMed; A. A. Sauve, BBA, Biochim. Biophys. Acta, Proteins Proteomics, 2010, 1804, 1591–1603 CrossRef PubMed.
- T. Jank and K. Aktories, Proc. Natl. Acad. Sci. U. S. A., 2013, 110, 4163–4164 CrossRef CAS PubMed; K. Aktories, Nat. Rev. Microbiol., 2011, 9, 487–498 CrossRef PubMed.
- S. Quan, T. Imai, Y. Mikami, K. Yazawa, E. R. Dabbs, N. Morisaki, S. Iwasaki, Y. Hashimoto and K. Furihata, Antimicrob. Agents Chemother., 1999, 43, 181–184 CAS.
- G. J. Williams, C. Zhang and J. S. Thorson, Nat. Chem. Biol., 2007, 3, 657–662 CrossRef CAS PubMed; A. D. Harper and M. Bar-Peled, Plant Physiol., 2002, 130, 2188–2198 CrossRef PubMed; C. J. Thibodeaux, C. E. Melancon and H. W. Liu, Nature, 2007, 446, 1008–1016 CrossRef PubMed.
- J. Chen, L. C. Zhang, Y. M. Xing, Y. Q. Wang, X. K. Xing, D. W. Zhang, H. Q. Liang and S. X. Guo, PLoS One, 2003, 8, e58268 Search PubMed.
- Y. L. Zhang, H. M. Ge, W. Zhao, H. Dong, Q. Xu, S. H. Li, J. Li, J. Zhang, Y. C. Song and R. X. Tan, Angew. Chem., Int. Ed., 2008, 47, 5823–5826 CrossRef CAS PubMed; Y. L. Zhang, J. Zhang, N. Jiang, Y. H. Lu, L. Wang, S. H. Xu, W. Wang, G. F. Zhang, Q. Xu, H. M. Ge, J. Ma, Y. C. Song and R. X. Tan, J. Am. Chem. Soc., 2011, 133, 5931–5940 CrossRef PubMed; W. Fang, S. Ji, N. Jiang, W. Wang, G. Y. Zhao, S. Zhang, H. M. Ge, Q. Xu, A. H. Zhang, Y. L. Zhang, Y. C. Song, J. Zhang and R. X. Tan, Nat. Commun., 2012, 3, 1039 CrossRef PubMed.
- S. Raffaele and S. Kamoun, Nat. Rev. Microbiol., 2012, 10, 417–430 CAS; J. P. McCutcheon and N. A. Moran, Nat. Rev. Microbiol., 2012, 10, 13–26 Search PubMed.
- C. P. Woloshuk and W. B. Shim, FEMS Microbiol. Rev., 2013, 37, 94–109 CrossRef CAS PubMed.
- G. J. Soleas, E. P. Diamandis and D. M. Goldberg, Clin. Biochem., 1997, 30, 91–113 CrossRef CAS.
- S. J. Park, F. Ahmad, A. Philp, K. Baar, T. Williams, H. Luo, H. Ke, H. Rehmann, R. Taussig, A. L. Brown, M. K. Kim, M. A. Beaven, A. B. Burgin, V. Manganiello and J. H. Chung, Cell, 2012, 148, 421–433 CrossRef CAS PubMed; S. Bae, E. M. Lee, H. J. Cha, K. Kim, Y. Yoon, H. Lee, J. Kim, Y. J. Kim, H. G. Lee, H. K. Jeung, Y. H. Min and S. An, Mol. Cells, 2011, 32, 243–249 CrossRef PubMed.
- L. X. Wang, A. Heredia, H. J. Song, Z. J. Zhang, B. Yu, C. Davis and R. Redfield, J. Pharm. Sci., 2004, 93, 2448–2457 CrossRef CAS PubMed.
- H. Imai, M. Kitagawa, K. Ishihara, N. Masuoka, K. Shimoda, N. Nakajima and H. Hamada, Biosci., Biotechnol., Biochem., 2012, 76, 1552–1554 CrossRef CAS PubMed.
- T. Desmet, W. Soetaert, P. Bojarova, V. Kren, L. Dijkhuizen, V. Eastwick-Field and A. Schiller, Chem.–Eur. J., 2012, 18, 10786–10801 CrossRef CAS PubMed.
- I. Ahuja, R. Kissen and A. M. Bones, Trends Plant Sci., 2012, 17, 73–90 CrossRef CAS PubMed; A. Hammerbacher, S. G. Ralph, J. Bohlmann, T. M. Fenning, J. Gershenzon and A. Schmidt, Plant Physiol., 2011, 157, 876–890 CrossRef PubMed; P. Jeandet, B. Delaunois, A. Conreux, D. Donnez, V. Nuzzo, S. Cordelier, C. Clement and E. Courot, BioFactors, 2010, 36, 331–341 CrossRef PubMed.
- I. Ahuja, R. Kissen and A. M. Bones, Trends Plant Sci., 2012, 17, 73–90 CrossRef CAS PubMed; B. Delaunois, S. Cordelier, A. Conreux, C. Clement and P. Jeandet, Plant Biotechnol. J., 2009, 7, 2–12 CrossRef PubMed.
- H. X. Ge, J. Zhang, C. Kai, J. H. Liu and B. Y. Yu, Appl. Microbiol. Biotechnol., 2012, 93, 2357–2364 CrossRef CAS PubMed.
- Z. Ahmed, EJB Electron. J. Biotechnol., 2011, 4, 1–9 Search PubMed.
- W. Fang, S. Ji, N. Jiang, W. Wang, G. Y. Zhao, S. Zhang, H. M. Ge, Q. Xu, A. H. Zhang, Y. L. Zhang, Y. C. Song, J. Zhang and R. X. Tan, Nat. Commun., 2012, 3, 1039 CrossRef CAS PubMed; M. Marzorati, B. Danieli, D. Haltrich and S. Riva, Green Chem., 2005, 7, 310–315 RSC.
- R. W. Gantt, P. Peltier-Pain, S. Singh, M. Zhou and J. S. Thorson, Proc. Natl. Acad. Sci. U. S. A., 2013, 110, 7648–7653 CrossRef CAS PubMed; H. Lin, Org. Biomol. Chem., 2007, 5, 2541–2554 Search PubMed.
- R. K. Gholson, Nature, 1966, 212, 933–935 CrossRef CAS.
- G. S. Stupp, S. H. von Reuss, Y. Izrayelit, R. Ajredini, F. C. Schroeder and A. S. Edison, ACS Chem. Biol., 2013, 8, 309–313 CrossRef CAS PubMed.
- H. Shimomura, K. Hosoda, D. J. McGee, S. Hayashi, K. Yokota and Y. Hirai, J. Bacteriol., 2013, 195, 359–367 CrossRef CAS PubMed.
- M. S. C. Pedras, S. Hossain and R. B. Snitynsky, Phytochemistry, 2011, 72, 199–206 CrossRef CAS PubMed; A. C. Sexton, Z. Minic, A. J. Cozijnsen, M. S. C. Pedras and B. J. Howlett, Fungal Genet. Biol., 2009, 46, 201–209 CrossRef PubMed.
- G. Taguchi, M. Nakamura, N. Hayashida and M. Okazaki, Plant Sci., 2003, 164, 231–240 CrossRef CAS.
- S. R. Baerson, A. Sanchez-Moreiras, N. Pedrol-Bonjoch, M. Schulz, I. A. Kagan, A. K. Agarwal, M. J. Reigosa and S. O. Duke, J. Biol. Chem., 2005, 280, 21867–21881 CrossRef CAS PubMed.
- C. Loutre, D. P. Dixon, M. Brazier, M. Slater, D. J. Cole and R. Edwards, Plant J., 2003, 34, 485–493 CrossRef CAS.
- L. Cuthbertson, V. Kos and C. Whitfield, Microbiol. Mol. Biol. Rev., 2010, 74, 341–362 CrossRef CAS PubMed.
- C. F. Higgins, Nature, 2007, 446, 749–757 CrossRef CAS PubMed; M. S. Jin, M. L. Oldham, Q. Zhang and J. Chen, Nature, 2012, 490, 566–569 CrossRef PubMed.
- J. W. Slaven, M. J. Anderson, D. Sanglard, G. K. Dixon, J. Bille, I. S. Roberts and D. W. Denning, Fungal Genet. Biol., 2002, 36, 199–206 CrossRef CAS.
- A. Kovalchuk and A. J. M. Driessen, BMC Genomics, 2010, 11, 177 CrossRef PubMed.
- H. V. Colot, G. Park, G. E. Turner, C. Ringelberg, C. M. Crew, L. Litvinkova, R. L. Weiss, K. A. Borkovich and J. C. Dunlap, Proc. Natl. Acad. Sci. U. S. A., 2006, 103, 10352–10357 CrossRef CAS PubMed.
- A. Kovalchuk and A. J. M. Driessen, BMC Genomics, 2010, 11 DOI:10.1186/1471-2164-11-177; N. Shitan, I. Bazin, K. Dan, K. Obata, K. Kigawa, K. Ueda, F. Sato, C. Forestier and K. Yazaki, Proc. Natl. Acad. Sci. U. S. A., 2003, 100, 751–756 CrossRef PubMed; K. Terasaka, J. J. Blakeslee, B. Titapiwatanakun, W. A. Peer, A. Bandyopadhyay, S. N. Makam, O. R. Lee, E. L. Richards, A. S. Murphy, F. Sato and K. Yazaki, Plant Cell, 2005, 17, 2922–2939 CrossRef PubMed.
- J. J. Wenzel, A. Piehler and W. E. Kaminski, Front. Biosci., 2007, 12, 3177–3193 CrossRef CAS PubMed.
Footnotes |
† Electronic supplementary information (ESI) available. See DOI: 10.1039/c4ra12851f |
‡ Guo-Yan Zhao and Jing-Yang Fan contributed equally to the work. |
|
This journal is © The Royal Society of Chemistry 2015 |