DOI:
10.1039/C4RA12428F
(Paper)
RSC Adv., 2015,
5, 10204-10210
Development of a highly efficient single-mode microwave applicator with a resonant cavity and its application to continuous flow syntheses†
Received
15th October 2014
, Accepted 5th January 2015
First published on 6th January 2015
Abstract
Microwave-assisted organic synthesis has many advantages and is widely applied to a variety of reactions. We have developed a new single-mode microwave applicator, specific to continuous flow synthesis, whose main feature is that it generates a uniform electromagnetic field inside its resonant cavity. Two well-known reactions, the Fischer indole synthesis and the Diels–Alder reaction, proceeded very quickly when a solution of substrates was pumped through a helical glass tube reactor inside the resonant cavity, under a pressure of 2.5 MPa. The desired products were obtained in high yields. This compact apparatus constitutes a new method for switching organic synthesis from batch to continuous flow, and enables continuous synthesis of products at a scale of 100 g h−1 or more.
Introduction
Organic syntheses using microwave (MW) applicators have made tremendous progress during the past three decades. In the beginning, most applicators used were modified domestic MW ovens. Subsequently, specialized MW applicators for chemical synthesis, equipped with small-scale batch reactors and temperature and pressure control functions, have been developed and commercially supplied. Consequently, the number of MW-assisted reactions has been increasing. MW irradiation has become one of the most popular heating methods for organic synthesis and has found applications in various fields, such as heterocyclic chemistry, natural product synthesis, and medicinal chemistry. Compared with traditional external heating methods that use oil baths, heating blocks, etc., the MW method enables direct and rapid heating of substrates and solvents and generates a more uniform heating profile, which significantly reduces reaction times and, in many cases, increases yields and saves energy and resources.1
However, scaling up of the MW-assisted organic synthesis is restricted by the limited penetration depth (e.g., about 1 cm in water at 25 °C, 2.45 GHz)1b,1c and irradiation power of microwaves. Although large multimode MW applicators2 and stop-flow synthesis3 that partly overcome these problems have been developed, different and more fundamental solutions are in great demand in order to make large-scale production possible.
Considering the advantages of flow synthesis, such as a large surface-to-volume ratio, which enables fast heating, as well as a relatively small flow reactor volume that minimizes the risk of accidents under high pressure and high temperature,4 we intended to combine the benefits of flow synthesis and MW synthesis. While the majority of continuous flow apparatuses use conductive heating equipment, such as oil baths, heated air, and heated metal blocks, continuous flow devices using modified batch MW applicators have also been developed.1,4d,5,6 Recently, new technologies for the MW-assisted continuous flow syntheses have been developed that use nonresonant MW applicators,7 the follow MW applicators equipped with small-volume reactors,8,9 special reactors using good MW-absorbing materials,6b,10 a transmission line short-circuited waveguide reactor,11 and transient processing through MW cavities in series.12 Despite these efforts, there is still a great need for improving these devices with respect to energy efficiency, fine tuning of irradiation frequency, and productivity per unit time. Additionally, in most of the reported apparatuses, temperature is monitored by IR sensors located outside the reactor, because of which the actual temperature of the reaction mixture is often underestimated.1f,13
We present herein a new single-mode, bench-top MW applicator for the continuous flow syntheses. This apparatus features the following: (1) highly efficient MW irradiation (up to 200 W) by generating a uniform electromagnetic field in its resonant cavity,14 (2) a wide and long helical tubular glass reactor (i.d. 3.6 mm, internal volume: 5.5–6.0 mL) in a resonant cavity,15 (3) an original control system that enables quick and fine adjustment to the best irradiation frequency in accordance with the changes in the electric permittivity of the reaction mixture,16 and (4) direct measurement of the temperature in the reaction mixture using a thermocouple set at the exit of the reactor. Using this apparatus, we have observed some of the characteristic advantages of MW heating, which are difficult to achieve by external heating and have demonstrated the continuous production of more than 100 g h−1 (0.67 mol h−1) of an indole derivative.
Results and discussion
The new apparatus for MW-assisted flow synthesis (Fig. 1a) consists of a MW generator, a resonant cavity (8 cm × 8 cm × 20 cm), a helical tubular borosilicate glass reactor (i.d. 3.6 mm, internal volume in the resonant cavity: 5.5–6.0 mL, see: Fig. 1b), a pumping system, and a control device. In particular, we have devised a novel system for generating a uniform electromagnetic field in the resonant cavity, which makes use of a solid-state device for telecommunications17 in order to generate the MW. This is the key to achieving quick and fine adjustments of the irradiation frequency, according to the changes in the electric permittivity of the reaction mixture. This device produces up to 200 W output in a frequency range covering 2.4 to 2.5 GHz. The irradiation power, reflected power, electric field in the cavity, temperature of the reaction mixture at the exit of the reactor (a thermocouple is set inside the helical tube reactor) and pressure of the reaction mixture are monitored and controlled in real time. The flow system is equipped with a back-pressure regulator next to the glass reactor, in order to maintain the pressure of the reaction mixture at up to 2.5 MPa. All these components are compactly assembled in the space of 160 cm × 60 cm × 60 cm.
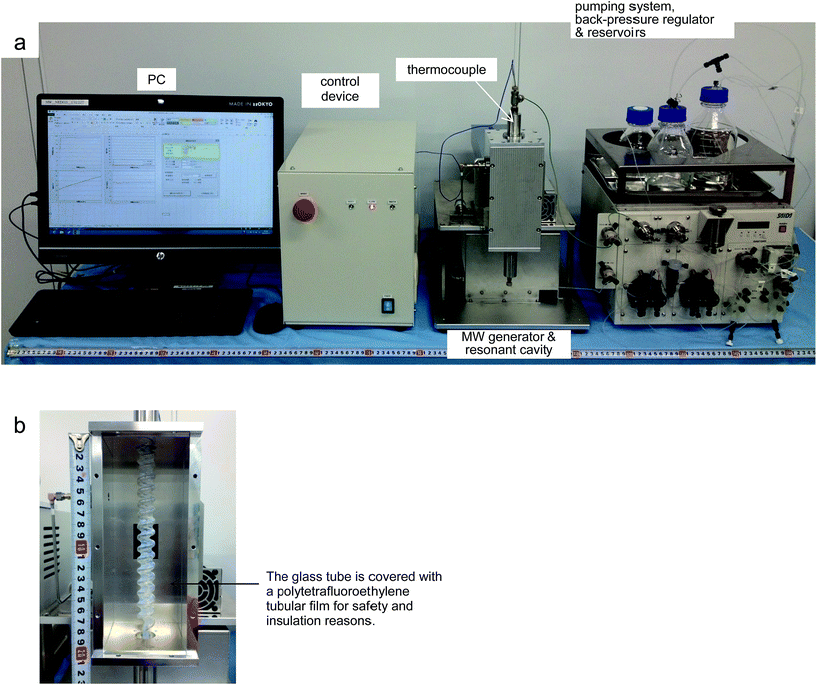 |
| Fig. 1 (a) Entire apparatus for MW-assisted flow synthesis. (b) The helical borosilicate glass tube reactor set in the resonant cavity. | |
In an initial study to evaluate the performance of this apparatus, acetonitrile (MeCN) was pumped into the reactor under a backpressure of 2.5 MPa, and the heating profile was recorded while changing the irradiation power (50–200 W) and the flow rate (5–20 mL min−1). In general, the temperature of MeCN at the exit of the reactor reached a steady state in less than 6 min after starting the flow. The temperature increased following the increase in irradiation power and the decrease in flow rate (for details, see: ESI†). Under some conditions, the temperature rose up to 230 °C; however, further increase of the irradiation power at the lower flow rate brought the MeCN to boiling, resulting in the disruption of the temperature, pressure, and electric field in the cavity. Therefore, the following studies were carried out under the conditions that did not cause either the solvents or the reactants to boil. The surface temperature of the entire helical tube reactor was directly monitored by a thermal imaging infrared camera, showing that the temperature continuously increased from the entrance of the reactor up to two thirds of its length and remained almost the same on the last third (for details, see: ESI†).
Next, we studied the temperature profiles of a dozen common organic solvents under identical conditions (backpressure 2.5 MPa, irradiation power 200 W, flow rate 20 mL min−1). During their passage through the reactor, in about 16 s, all solvents beside toluene were heated way above their boiling point under atmospheric conditions (the exit temperature of each solvent after reaching a steady state is given in Table 1). The exit temperature of each solvent seemed to be dependent on the physicochemical nature of the solvent and have a insignificant relation to its boiling point under atmospheric conditions, a trend that is never observed during external heating.18 The solvents examined were classified into four groups, based on the exit temperature, as follows: (1) 100–110 °C, hydrocarbons such as n-hexane and toluene; (2) close to 150 °C, aprotic less polar solvents such as ethyl acetate (EtOAc) and cyclopentyl methyl ether; (3) 180–200 °C, protic solvents such as alcohols and acetic acid (AcOH); and (4) above 210 °C, aprotic polar solvents such as N,N-dimethyl formamide and dimethyl sulfoxide. These data are in accordance with the characteristic feature of MW heating, viz., a heating profile that is dependent on the physicochemical nature of each solvent. It is also worth noting that many solvents were heated to over 150 °C in about 16 s (residence time) with an irradiation power of 200 W and a flow rate 20 mL min−1, an achievement which was due to the high efficiency of the MW irradiation and the high adjustment ability of this apparatus.
Table 1 The exit temperature of common organic solvents heated using a continuous-flow MW apparatusa
Organic solvent |
Temperature (°C) at the exit of the reactorb |
Boiling point (°C) under atmospheric conditions |
Each organic solvent at room temperature was pumped through the reactor at the flow rate of 20 mL min−1 under a back-pressure of 2.5 MPa, with a MW irradiation of 200 W. Solvent temperature after reaching a steady state, measured directly using a thermocouple installed at the exit of the reactor. |
n-hexane |
101 |
69 |
Toluene |
109 |
111 |
EtOAc |
148 |
77 |
Cyclopentyl methyl ether |
151 |
106 |
MeOH |
178 |
65 |
EtOH |
185 |
79 |
n-PrOH |
198 |
97 |
AcOH |
200 |
118 |
MeCN |
209 |
82 |
N,N-Dimethyl formamide |
220 |
153 |
N,N-Dimethyl acetamide |
225 |
165 |
Dimethyl sulfoxide |
>250 |
189 |
With these basic results in hand, we next applied our MW flow apparatus to the Fischer indole synthesis (Fig. 2).19–22 A 1.0 M solution of cyclohexanone (1) in AcOH (solution A) and a 1.1 M solution of phenylhydrazine (2) in MeCN (solution B) were separately pumped into the reactor at the same flow rate via a mixer installed just before the reactor. The conditions were varied by changing the flow rate of the mixed solution (13–17 mL min−1) and the irradiation power (130–190 W) and each reaction was evaluated using the GC yield of 1,2,3,4-tetrahydro-1H-carbazole (4) measured after the exit temperature reached a steady state (Table 2, upper line of each cell). We found that the higher yields of 4 were obtained when the exit temperature was also higher. At the same flow rate, a better yield of 4 was obtained by increasing the irradiation power; however, the reaction mixture began to boil at around 240 °C.
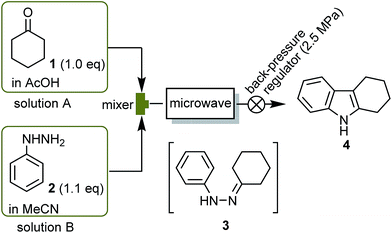 |
| Fig. 2 Preparation of 1,2,3,4-tetrahydro-1H-carbazole (4) from 1 and 2 using the continuous-flow MW apparatus. | |
Table 2 The GC yield of 1,2,3,4-tetrahydro-1H-carbazole (4) and the exit temperature under various conditions by changing the flow rate, irradiation power, and concentration of 1
Flow ratec (mL min−1) and residence timed |
Concentration (M) of 1 |
GC yield (%) of 4a and exit temperature (°C)b |
Irradiation power (W) |
130 |
150 |
170 |
190 |
n-Decane was used as the internal standard. The exit temperature of the reaction mixture after reaching a steady state, measured directly using a thermocouple at the exit of the reactor. Flow rate of a 1 : 1 mixture of solutions A and B. The residence time in the reactor (internal volume 5.2 mL) is shown in the parenthesis. A 1.0 M solution of 1 in AcOH and a 1.1 M solution of 2 in MeCN were pumped into the reactor at the same flow-rate under the back-pressure of 2.5 MPa and MW irradiation of the given power. Not available due to boiling of the reaction mixture. A 2.0 M solution of 1 in AcOH and a 2.2 M solution of 2 in MeCN were used, and the remainder of the procedure was the same as described in footnote e. |
13 (24 s) |
1.0e |
54, 205 |
83, 232 |
—f |
—f |
2.0g |
63, 219 |
—f |
—f |
—f |
15 (21 s) |
1.0e |
35, 189 |
43, 199 |
60, 213 |
81, 233 |
2.0g |
50, 206 |
70, 232 |
—f |
—f |
17 (18 s) |
1.0e |
14, 156 |
22, 173 |
38, 194 |
51, 209 |
2.0g |
27, 180 |
37, 195 |
59, 219 |
—f |
Interestingly, when the concentration of solutions A and B was doubled (2.0 M of solution A and 2.2 M of solution B), under the same conditions, the reaction produced a higher yield of 4, while also exhibiting higher exit temperatures than the reaction using 1.0 M of solution A (Table 2, compare the upper and lower lines in each cell). These phenomena proves that the substrates (1 and 2) and/or intermediate 3 absorbed the MW more effectively when concentrated, speeding up the reaction, which is another characteristic feature of MW heating.
Having established the optimum conditions for the continuous production of 4, we next examined a 100 g scale synthesis. Continuous operation was conducted by pumping a 2.0 M solution of A and a 2.2 M solution of B at a constant rate (total flow rate of 15 mL min−1), maintaining the exit temperature at around 240 °C to avoid boiling the reaction mixture. Consequently, as much as 115 g of 4 were produced during a 1 h operation (75% yield based on 1).
With the success in the Fischer indole synthesis clearly demonstrating the utility of our apparatus, we next turned our attention to another representative organic transformation. The Diels–Alder (DA) reaction of acetylenedicarboxylates and furans is known to be very sensitive to the reaction temperature. Under conventional external heating conditions, the reaction is generally conducted at temperatures not exceeding 110 °C (refluxing toluene) for a long time, such as 10 h or more, because the retro DA reaction and/or decomposition takes place at higher temperatures.23 MW-heating was also applied to the DA reactions, in which significant decomposition was observed above 150 °C.24 We assumed that our MW-assisted flow reactor would be suitable to overcome these difficulties with the expectation that the high reaction temperature applied for a short period of time would be not only sufficient to promote the DA reaction, but also effective in suppressing the undesired reactions. To illustrate our idea, we applied our MW apparatus to the DA reaction of diethyl acetylenedicarboxylate (5) and furan (6) as a model case (Fig. 3).
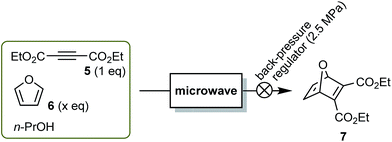 |
| Fig. 3 A DA reaction of 5 and 6 using the continuous-flow MW apparatus. | |
A mixture of 5 (1.0 M) and 6 (2.0 M) in n-PrOH25 was pumped into the reactor with the flow rate and irradiation power varying over a range of 1–10 mL min−1 and 20–80 W, respectively (Table 3, upper line of each cell). This extensive survey revealed some characteristic tendencies of the reaction, as follows: as seen from Table 3, an optimum irradiation power could be determined for every specific flow rate, and vice versa.26 The combination of parameters that gave the maximum yield shifted from the upper left to the lower right of Table 3. At this point, the exit temperature also increased to more than 200 °C (compare 1.2 mL min−1, 30 W, 71% and 5.0–6.0 mL min−1, 70 W, 70%). That is, the highest yield was achieved only when the reaction mixture underwent high temperatures for as short a time as possible. However, we could not run the reaction at an exit temperature above 200 °C because the temperature fluctuated. The best yield of 7 was 76% at a flow rate of 1.0 mL min−1 with 30 W irradiation.
Table 3 The NMR yield of the product (7) and the exit temperature under various conditions by changing the flow rate, irradiation power, and concentration of 6
Flow rate (mL min−1) and residence timec |
Concentration (M) of 6 |
NMR yield (%) of 7a and exit temperatureb (°C) |
Irradiation power (W) |
20 |
30 |
40 |
50 |
60 |
70 |
80 |
The yield was based on the ratio of 5 and 7 determined by 1H NMR analysis of a crude product. The exit temperature of the reaction mixture was directly measured by a thermocouple installed at the exit of the reactor, after reaching a steady state. The residence time in the reactor (internal volume 6.0 mL) is shown in the parenthesis. A solution of 5 (1.0 M) and 6 (2.0 M) in n-PrOH was pumped into the reactor at the given flow rate with the given irradiation power, under the back-pressure of 2.5 MPa. A solution of 5 (1.0 M) and 6 (4.0 M) in n-PrOH was pumped into the reactor at the given flow rate with the given irradiation power under the back-pressure of 2.5 MPa. The exit temperature of the reaction mixture fluctuated in the range of 200–210 °C. |
1.0 (6.0 min) |
2.0d |
52, 106 |
76, 135 |
67, 162 |
43, 180 |
|
|
|
4.0e |
|
78, 136 |
59, 168 |
|
|
|
|
1.2 (5.0 min) |
2.0d |
50, 112 |
71, 140 |
61, 167 |
50, 180 |
|
|
|
4.0e |
|
79, 142 |
65, 169 |
|
|
|
|
1.5 (4.0 min) |
2.0d |
42, 113 |
69, 139 |
70, 168 |
49, 186 |
|
|
|
4.0e |
|
81, 144 |
72, 168 |
|
|
|
|
2.0 (3.0 min) |
2.0d |
|
60, 140 |
67, 171 |
67, 194 |
63, 200 |
|
|
4.0e |
|
|
80, 171 |
69, 190 |
|
|
|
2.5 (2.4 min) |
2.0d |
|
|
70, 169 |
70, 190 |
61, 205 |
|
|
4.0e |
|
|
80, 171 |
74, 191 |
|
|
|
3.0 (2.0 min) |
2.0d |
|
|
63, 165 |
69, 184 |
67, 205 |
|
|
4.0e |
|
|
|
82, 189 |
77, >200f |
|
|
4.0 (1.5 min) |
2.0d |
|
|
55, 159 |
61, 175 |
69, 196 |
|
|
4.0e |
|
|
|
83, 189 |
82, 195 |
|
|
5.0 (1.2 min) |
2.0d |
|
|
|
48, 167 |
62, 189 |
70, 205 |
|
4.0e |
|
|
|
77, 170 |
85, 194 |
77, >200f |
|
6.0 (1.0 min) |
2.0d |
|
|
|
54, 170 |
49, 182 |
70, 200 |
|
4.0e |
|
|
|
73, 162 |
78, 180 |
79, >200f |
|
8.0 (45 s) |
2.0d |
|
|
|
|
|
48, 182 |
64, 200 |
4.0e |
|
|
|
|
73, 172 |
76, 187 |
81, >200f |
10.0 (36 s) |
2.0d |
|
|
|
|
|
56, 182 |
64, 200 |
4.0e |
|
|
|
|
|
73, 177 |
78, >200f |
The use of a double amount of 6 (4.0 M) generally resulted in a better yield of 7 under any of the conditions used (Table 3, lower line of each cell), and the yield was increased to 85% (flow rate 5.0 mL min−1, irradiation power 60 W, exit temperature 194 °C). The continuous-flow reaction of a solution of 5 (1.1 M) and 6 (4.4 M) in n-propanol (n-PrOH) produced, during 5 min, 4.9 g of 7 (76% isolated yield). This is the highest productivity per unit time (1.0 g min−1) achieved, in comparison with the similar DA reactions under continuous MW flow synthesis.10,13c Thus, the continuous-flow MW apparatus enabled, at high flow rate (∼4 mL min−1), the rapid heating (residence time ≤1.5 min) and rapid cooling necessary to minimize the retro DA reaction that usually occurs at such temperatures (190–200 °C).
Conclusions
We have developed a new MW apparatus for continuous-flow synthesis that features highly efficient single-mode MW irradiation by generating a uniform electromagnetic field in its resonant cavity. The quick and fine adjustment of the irradiation frequency was achieved by obtaining immediate feedback on the reflected power and electric field in the cavity, and the best resonance was maintained in accordance with the continuous change in the electric permittivity of the reaction mixture. With a back-pressure of 2.5 MPa, this apparatus is able to heat polar solvents and reactants up to >250 °C under maximum 200 W-irradiation, with the solution passing through the tube reactor for as short a time as 16 s. The thermocouple installed at the exit of the reactor displayed the temperature of the reaction mixture in real time.
The practical usefulness of this apparatus was demonstrated using two typical organic reactions: the Fischer indole synthesis and the DA reaction. In this way we have shown that our system combines the characteristic advantages of the MW-assisted heating, viz., the solvent- and reactant-dependent, highly effective absorption of the MW energy, with those of the flow synthesis, viz., the continuous production, the precise control of the reaction temperature, and the rapid heating and rapid cooling. Thus, this small bench-top apparatus can help synthesize products at a scale of more than 100 g h−1. Further testing of our new synthetic tool in a wide range of organic reactions is now in progress in our laboratory.
Experimental
The continuous preparation of 1,2,3,4-tetrahydro-1H-carbazole (4) during 60 min using the continuous flow MW apparatus
A solution of cyclohexanone (1) in AcOH (2.0 M) and a solution of phenylhydrazine (2) in MeCN (2.2 M) were pumped into the reactor at the same flow rate (total flow rate of 15 mL min−1) with the MW irradiation of 145 W. After 5 min, the exit temperature reached a steady state at 240 °C. The collection of the crude reaction mixture into a flask was started at this time and continued during the following 60 min, while the exit temperature kept at around 240 °C by adjusting the irradiation power in a range of 130–150 W to avoid boiling the reaction mixture. The time-course of the exit temperature and the irradiation power are shown in Fig. S6 of ESI.† The crude reaction mixture was placed in a refrigerator (ca. 4 °C) for 3 h, and the precipitate was filtered to give 52.6 g of 1,2,3,4-tetrahydro-1H-carbazole (4). The filtrate was concentrated and the residue was dissolved in EtOAc and washed with brine. The organic layer was separated, dried with MgSO4, and concentrated in vacuo. The residue was purified by recrystallization from MeCN to provide 47.1 g of 4. The mother liquor was concentrated in vacuo and purified by column chromatography on silica gel (n-hexane/EtOAc = 10
:
1) to give 15.3 g of 4. Each product was obtained as a pale brown solid, and its purity was examined by 1H NMR analysis to be more than 95%. Thus, 115 g (75% yield based on 1) of 4 was obtained in total. Mp 112–115 °C (lit.22 114–116 °C). δH (500 MHz; CDCl3; Me4Si) 1.87–1.95 (m, 4H, C(2)-H2 and C(3)-H2), 2.71–2.75 (m, 4H, C(1)-H2 and C(4)-H2), 7.06–7.13 (m, 2H, C(6)-H and C(7)-H), 7.28 (d, J = 8 Hz, 1H, C(8)-H), 7.46 (d, J = 8 Hz, 1H, C(5)-H), 7.66 (br s, 1H, NH). δC (125 MHz; CDCl3) 20.9, 23.19, 23.21, 23.3, 110.1, 110.3, 117.7, 119.1, 120.9, 127.8, 134.0, 135.6.
The Diels–Alder reaction of diethyl acetylenedicarboxylate (5) and furan (6) by the continuous flow MW apparatus
A solution of diethyl acetylenedicarboxylate (5) (1.1 M) and furan (6) (4.4 M) in n-PrOH was pumped into the reactor at the flow rate of 5.0 mL min−1 with the MW irradiation of 60 W. After 5 min, the exit temperature reached a steady state at 194 °C. The collection of the crude reaction mixture was started at this time and continued during the following 5 min, while the exit temperature kept at 194 °C. The crude product was concentrated in vacuo, and the residue was purified by column chromatography on silica gel (n-hexane/EtOAc = 10
:
1 to 3
:
1) to give diethyl 7-oxabicyclo[2.2.1]hepta-2,5-diene-2,3-dicarboxylate (7)10 (4.91 g, 76%) as a slightly yellowish oil. δH (500 MHz; CDCl3; Me4Si) 1.32 (t, J = 7 Hz, 6H, CH3 × 2), 4.27 (q, J = 7 Hz, 4H, CH2 × 2), 5.68 (s, 2H, CH–O × 2), 7.22 (br s, 2H, vinyl-H × 2). δC (125 MHz; CDCl3) 14.1 (CH3), 61.4 (CH2), 85.1 (C–O), 143.2 (vinyl-CH), 152.7 (vinyl-C), 163.1 (C
O).
Acknowledgements
This work was financially supported by Shizuoka Industrial Foundation, NEDO Support Program for Ventures Involved in Innovation and Practical Application FY2012, and University of Shizuoka.
Notes and references
- For selected recent reviews:
(a) M. B. Gawande, S. N. Shelke, R. Zboril and R. S. Varma, Acc. Chem. Res., 2014, 47, 1338 CrossRef CAS PubMed;
(b) S. Horikoshi and N. Serpone, Catal. Sci. Technol., 2014, 4, 1197 RSC;
(c) A. de la Hoz and A. Loupy, Microwaves in Organic Synthesis, Wiley-VCH, Weinheim, 3rd edn, 2013 Search PubMed;
(d) R. S. Varma, in Innovations in Green Chemistry and Green Engineering, ed. P. T. Anastas and J. B. Zimmerman, Springer, New York, 2013, ch. 5, pp. 115–156 Search PubMed;
(e) A. Chawla and P. Kaur, Int. Res. J. Pharm., 2013, 4, 49 CrossRef;
(f) D. Garella, E. Borretto, A. Di Stilo, K. Martina, G. Cravotto and P. Cintas, Med. Chem. Commun., 2013, 4, 1323 RSC;
(g) C. O. Kappe, B. Pieber and D. Dallinger, Angew. Chem., Int. Ed., 2013, 52, 1088 CrossRef CAS PubMed;
(h) A. Majumder, R. Gupta and A. Jain, Green Chem. Lett. Rev., 2013, 6, 151 CrossRef CAS;
(i) C. O. Kappe, A. Stadler, D. Dallinger, R. Mannhold, H. Kubinyi and G. Folkers, Microwaves in Organic and Medicinal Chemistry, Wiley-VCH, Weinheim, 2nd edn, 2012 Search PubMed;
(j) H. M. A. Hassan, S. Harakeh, K. A. Sakkaf and I. Denetiu, Aust. J. Chem., 2012, 65, 1647 CrossRef CAS;
(k) A. Dastan, A. Kulkarni and B. Török, Green Chem., 2012, 14, 17 RSC;
(l) S. Sadler, A. R. Moeller and G. B. Jones, Expert Opin. Drug Discovery, 2012, 7, 1107 CrossRef CAS PubMed.
- For selected recent examples, see:
(a) D. Dallinger, H. Lehmann, J. D. Moseley, A. Stadler and C. O. Kappe, Org. Process Res. Dev., 2011, 15, 841 CrossRef CAS;
(b) M. D. Bowman, J. R. Schmink, C. M. McGowan, C. M. Kormos and N. E. Leadbeater, Org. Process Res. Dev., 2008, 12, 1078 CrossRef CAS.
- For selected recent examples, see:
(a) M. Schön, M. Schnürch and M. D. Mihovilovic, Mol. Diversity, 2011, 15, 639 CrossRef PubMed;
(b) J. A. Marafie and J. D. Moseley, Org. Biomol. Chem., 2010, 8, 2219 RSC;
(c) M. C. Bagley, T. Davis, M. C. Dix, V. Fusillo, M. Pigeaux, M. J. Rokicki and D. Kipling, Future Med. Chem., 2010, 2, 1417 CrossRef CAS PubMed.
- For selected recent reviews, see:
(a) T. Fukuyama, T. Totoki and I. Ryu, Green Chem., 2014, 16, 2042 RSC;
(b) H. Amii, A. Nagaki and J. Yoshida, Beilstein J. Org. Chem., 2013, 9, 2793 CrossRef PubMed;
(c) S. G. Newman and K. F. Jensen, Green Chem., 2013, 15, 1456 RSC;
(d) J. Wegner, S. Ceylan and A. Kirschning, Adv. Synth. Catal., 2012, 354, 17 CrossRef CAS;
(e) C. Wiles and P. Watts, Green Chem., 2012, 14, 38 RSC;
(f) T. N. Glasnov and C. O. Kappe, Chem.–Eur. J., 2011, 17, 11956 CrossRef CAS PubMed For selected examples, see: ;
(g) T. Razzaq, T. N. Glasnov and C. O. Kappe, Eur. J. Org. Chem., 2009, 1321 CrossRef CAS.
- For a recent review, see: E. Gjuraj, R. Kongoli and G. Shore, Chem. Biochem. Eng. Q., 2012, 26, 285 CAS.
- For recent examples, see:
(a) S. Christiaens, X. Vantyghem, M. Radoiu, J. Jacques and V. Eynde, Molecules, 2014, 19, 9986 CrossRef PubMed;
(b) I. R. Baxendale, C. Hornung, S. V. Ley, J. de M. M. Molina and A. Wikström, Aust. J. Chem., 2013, 66, 131 CrossRef CAS;
(c) B. Reichart, G. Tekautz and C. O. Kappe, Org. Process Res. Dev., 2013, 17, 152 CrossRef CAS;
(d) M. C. Bagley, V. Fusillo, R. L. Jenkins, M. C. Lubinu and C. Mason, Beilstein J. Org. Chem., 2013, 9, 1957 CrossRef PubMed;
(e) F. Ullah, Q. Zang, S. Javed, P. Porubsky, B. Neuenswander, G. H. Lushington, P. R. Hanson and M. G. Organ, Synthesis, 2012, 44, 2547 CrossRef CAS PubMed;
(f) A. M. Rodriguez, A. Juan, M. V. Gómez, A. Moreno and A. de la Hoz, Synthesis, 2012, 44, 2527 CrossRef CAS PubMed;
(g) F. Bergamelli, M. Iannelli, J. A. Marafie and J. D. Moseley, Org. Process Res. Dev., 2010, 14, 926 CrossRef CAS.
-
(a) P. Öhrngren, A. Fardost, F. Russo, J. S. Schanche, M. Fagrell and M. Larhed, Org. Process Res. Dev., 2012, 16, 1053 CrossRef;
(b) V. Konda, J. Rydfjord, J. Sävmarker and M. Larhed, Org. Process Res. Dev., 2014, 18, 1413 CrossRef CAS and references cited therein.
- A single-mode microwave applicator equipped with a straight quartz glass tube reactor (i.d. 1.5 mm × 10 cm) was developed. See: M. Nishioka, M. Miyakawa, Y. Daino, H. Kataoka, H. Koda, K. Sato and T. M. Suzuki, Ind. Eng. Chem. Res., 2013, 52, 4683 CrossRef CAS.
- Our prototype apparatus (irradiation power: 30 W, resonant cavity length: 10 cm, back pressure: 1 MPa) equipped with a helical tube borosilicate glass tube reactor (i.d.: 3.0 mm, inner volume: ca. 2 mL) was preliminarily used for the synthesis of silver nanoparticles. See: S. Horikoshi, T. Sumi and N. Serpone, Chem. Eng. Process., 2013, 73, 59 CrossRef CAS PubMed.
- M. J. Karney, K. A. Porter, E. K. Barnhardt and G. S. Vanier, RSC Adv., 2013, 3, 7106 RSC.
- R. Morschhäuser, M. Krull, C. Kayser, C. Boberski, R. Bierbaum, P. A. Püschner, T. N. Glasnov and C. O. Kappe, Green Process. Synth., 2012, 1, 281 Search PubMed.
- N. G. Patil, F. Benaskar, E. V. Rebrov, J. Meuldijk, L. A. Hulshof, V. Hessel and J. C. Schouten, Org. Process Res. Dev., 2014, 18, 1400 CrossRef CAS.
-
(a) C. O. Kappe, Chem. Soc. Rev., 2013, 42, 4977 RSC;
(b) J. Rydfjord, F. Svensson, M. Fagrell, J. Sävmarker, M. Thulin and M. Larhed, Beilstein J. Org. Chem., 2013, 9, 2079 CrossRef PubMed;
(c) G. Shore and M. G. Organ, Chem. Commun., 2008, 838 RSC;
(d) N. E. Leadbeater, S. J. Pillsbury, E. Shanahan and V. A. Williams, Tetrahedron, 2005, 61, 3565 CrossRef CAS PubMed.
- The nonresonant applicator (ref. 7a) is very different from our applicator. That is, in the former one, MW was applied to the reaction mixture in a straight tube reactor by mean of a helical antenna, and the axial field applicator allowed the MW field to be concentrated axially inside the coil. On the other hand, our applicator generates a uniform electromagnetic field in the resonant cavity, which uses a solid-state device for telecommunications. The inner volume of the reactor (about 1 mL), maximum irradiation power (150 W), and total flow rate (up to 4.2 mL min−1) of the former apparatus are smaller than those of ours (6 mL, 200 W, and 20 mL min−1, respectively).
- The use of a straight tube reactor (ref. 8) placed parallel to the electric field in the resonance cavity has
a limitation of its diameter expansion because the higher electric permittivity ε′ of a solvent readily causes the mismatching of the energy propagation and results in the reflection of the inlet microwave in front of the cavity. On the other hand, we used a helical tube reactor with a bigger inner diameter 3.6 mm (ca. 6 times the cross-sectional area compared to the reactor of (ref. 8)) based on our considerations of such a MW boundary condition (in detail, see: ESI†), which also enabled the higher flow rate (up to 20 mL vs. up to 3.0 mL for (ref. 8)). In addition, based on the computer simulation, we confirmed that a rectangular-parallelepiped resonator could generate axially symmetrical electric field distribution around the helical tube that was similar to that generated by a circular cylinder like as (ref. 8). Our rectangular resonator has some advantages, such as easy construction, easy adjustment of the iris’s width, and easy connection to rectangular waveguide.
- Compared with our prototype apparatus, the new reactor used in this work has made significant improvements on the irradiation power (200 W), resonant cavity length (20 cm), back pressure (2.5 MPa), tube reactor (i.d. 3.6 mm, inner volume: 5.5–6.0 mL), rapid response by using the latest programmable logic controller, and several safety measures. Thereby, the productivity of organic compounds, pursuit performance of the resonant frequency and operational safety have been dramatically increased.
- A resonator-type equipment requires irradiation at a specific resonant frequency. For this purpose, a solid-state oscillation device is more suitable to produce the specific frequency with constant output then a magnetron, which is generally used as microwave source, but can hardly control the oscillated frequency.
- These differences arise from the different parameters involved in the energy transfer. The temperature increase due to external heating is dependent only on the heat capacity of the solvents, while that achieved through MW irradiation is caused by the vibration of the electromagnetic field, and is affected by the polarity of molecule and the intermolecular restraint force of the vibrational motion.
- There have been reports of some Fischer indole synthesis performed using microflow reactors with a heat block,20 a microwave batch reactor,21 and continuous-flow microwave applicators.7,22.
- B. Wahab, G. Ellames, S. Passey and P. Watts, Tetrahedron, 2010, 66, 3861 CrossRef CAS PubMed.
- M. Desroses, K. Wieckowski, M. Stevens and L. R. Odell, Tetrahedron Lett., 2011, 52, 4417 CrossRef CAS PubMed.
- M. C. Bagley, R. L. Jenkins, M. C. Lubinu, C. Mason and R. Wood, J. Org. Chem., 2005, 70, 7003 CrossRef CAS PubMed.
- For selected examples, see:
(a) N. Sultan, R. Guillot, L. Blanco and S. Deloisy, Synthesis, 2013, 45, 2018 CrossRef CAS PubMed;
(b) M.-A. Raheem and W. Tam, Synth. Commun., 2013, 43, 260 CrossRef CAS;
(c) Y. De Xing and N. Z. Huang, J. Org. Chem., 1982, 47, 140 CrossRef.
- For microwave batch reactions, see: (ref. 13d) and references cited therein. For microwave continuous flow reactions, see: (ref. 8 and 13c).
- As a preliminary study, mixtures of 5 (1.0 M) and 6 (2.0 M) in several organic solvents were pumped into the reactor at a flow rate of 3 mL min−1 and the irradiation power was varied (50–70 W). This showed that n-PrOH is the most suitable solvent in terms of the yield of reaction product 7 and the moderate boiling point of the solvent that allows an easy workup.
- The decrease in the yield at higher temperatures was most probably due to the retro DA reaction of 7. This hypothesis was demonstrated by pumping a 1.0 M solution of 7 in n-PrOH into the reactor under 60 W irradiation at flow rate of 3.0 mL min−1 (residence time 2.0 min, exit temperature 197 °C). The end product was a 2
:
1 mixture of 7 and 5. On the other hand, the isolated 7 did not suffer any change if kept at room temperature for 27 h.
Footnote |
† Electronic supplementary information (ESI) available: General description of the microwave applicator, the exit temperature profile of MeCN by using the continuous flow MW apparatus under various conditions, the surface temperature of the helical tube reactor, comparison of our results with the related reactions using MW-assisted continuous flow reactors and a MW-assisted batch reactor, full experimental details, copies of spectral data. See DOI: 10.1039/c4ra12428f |
|
This journal is © The Royal Society of Chemistry 2015 |