DOI:
10.1039/C4RA12407C
(Review Article)
RSC Adv., 2015,
5, 11724-11732
Targeting cancer cells using aptamers: cell-SELEX approach and recent advancements
Received
15th October 2014
, Accepted 12th January 2015
First published on 12th January 2015
Abstract
Aptamers are short single stranded nucleic acid molecules, isolated from a pool of nucleic acid molecules by the method called SELEX (Systematic Evolution of Ligands by EXponential Enrichment) that can bind to the target with high affinity and specificity. A modified SELEX method where the targets are living whole cells is referred as cell-SELEX, and is of great interest because it opens up a simple and effective method for disease diagnostics and therapeutics. The cell-SELEX based approach and recent developments of the technique for identifying aptamers as well as their application in cancer diagnostics and therapeutics are summarized here.
Introduction
In spite of great effort and investments in cancer research over last few decades cancer remains one of the leading causes of suffering and death worldwide. A number of cancers are not detectable at early stage and therefore not treatable. On the other hand a large number of approved anticancer drugs have severe side effects and toxicity. Therefore, development of targeted delivery systems that could selectively target cancer cells and deliver the drug payload locally is of great significance.1 Identification of such targeting ligands will not only improve drug efficacy but will also reduce the systemic toxicity that is generally imparted by chemotherapeutics.
Aptamers are short single stranded oligonucleotides that can selectively bind to a target with high affinity and specificity because of their unique three dimensional structures. Even though aptamers are comparable with antibodies in terms of target recognition property, they are more favorable than antibodies because of several reasons. They are: (i) easy to synthesize and modify; (ii) mild- or non-immunogenic; (iii) easy to handle and store. Aptamers can be isolated from a pool of 1014–1016 molecules by an in vitro selection method known as SELEX (Systematic Evolution of Ligands by EXponential Enrichment).2,3 A wide variety of targets including small molecules (e.g., metal ions, small organic molecules, organic dyes, amino acids), polymeric molecules (e.g., peptides, proteins), and even more complex systems (e.g., virus, bacteria, whole organism, whole living cell) are utilized for identifying aptamers for various applications in diagnostics and targeted therapeutics. For e.g., an aptamer that has high affinity for vascular endothelial growth factor (VEFG), namely pegaptanib (Macugen®), has got approval from US Food and Drug Administration (FDA) for treating age related macular degeneration (AMD).4 Several other aptamer molecules are in different stages of clinical investigations.5,6
The traditional SELEX method starts with a chemically synthesized pool of oligonucleotides which contains a random region of 30–40 nucleotides in the middle and is flanked by constant PCR primer region of 15–20 nucleotides at both the ends. If the SELEX is done with DNA oligonucleotides, the oligonucleotide pool can directly be incubated with the target molecule. The unbound oligos are washed off and bound ones are eluted. The eluted single stranded DNA pool is amplified by polymerase chain reaction (PCR) followed by strand separation, which is then utilized for a second round of selection. In case of identifying an RNA aptamer, a pool of RNA oligonucleotide is prepared by in vitro transcription from the PCR amplified DNA double strand and incubated with the target molecule. After washing, the bound RNA pool is eluted and subjected to reverse transcription PCR, followed by an in vitro transcription to generate the RNA pool which is used in the second round of selection. This method is repeated several times and each time the washings are made harder in order to collect only those which are tightly bound to the target. Performing SELEX against a known protein or peptide though seemingly straightforward, may not be desired due to the fact that a purified protein or peptide target may differ from the actual cellular system and does not always take up the post-transcriptional modification which takes place in an actual cellular system.7 Previously, Cerchia and co-workers has shown that the aptamers isolated by whole-cell SELEX were unable to bind purified extracellular RETC634Y and also aptamers selected by targeting purified extracellular RETC634Y were unable to bind the membrane-bound RET.8 In another work Liu and co-workers have shown that an RNA aptamer selected against histidine-tagged EGFRvIII ectodomain, isolated from Escherichia coli expression, did not bind the full-length EGFRvIII protein isolated from baculovirus.9
In recent years, a modified SELEX method was developed where the target is the whole living cell itself and this method is known as cell-SELEX. As this method utilizes human cell as substrate, it has gained enormous interest for discovering new targeting molecules. Today, a large number of groups are working on cell-SELEX based strategy for detecting cancer cell specific markers and for developing new therapeutic modalities as this method offers multiple advantages.10–13 In cell based SELEX strategy, targets are a wide variety of proteins, lipids etc., those are expressed on live cell surface. At the same time this method does not require any prior knowledge of a specific cell surface marker. Instead, this method offers new possibilities in identifying unknown cell surface marker that can better differentiate a diseased cell from a normal cell. A variety of possibilities such as biomarker discovery, early detection tools, diagnosis, tumor cell enrichment as well as targeted drug delivery systems have opened up through this simple and effective method.
Cell-SELEX
Because of its immense potential cell-SELEX method (Fig. 1) has been widely used in clinical applications of cancer. Several groups are working to identify DNA aptamers those are specific to different cancer cell types. In a typical DNA aptamer selection the 5′-end of the sense strand and the forward PCR primer are labeled with fluorescein isothyocianate (FITC). This labeling helps monitoring the selection progress by flow cytometry. The reverse PCR primer is also labeled with biotin at the 5′-end, because the biotin labeling enables strand separation, which is essential before each round of selection. A negative selection is also done after the actual selection in each cycle to remove the non-specific binding. For this purpose one essentially needs a good negative control, which is often not known and thus not available for many cancer cell types.
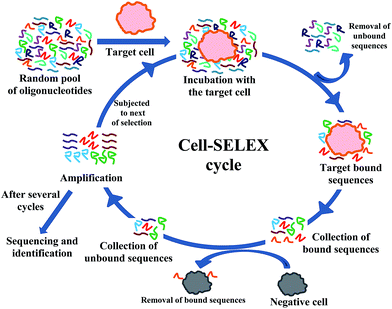 |
| Fig. 1 Schematic presentation of a typical cell-SELEX procedure. | |
Even though RNA strands are structurally more diverse than those of DNAs, reports on RNA aptamer selection using cell-SELEX are relatively less as compared to the DNA counterpart owing to their low nuclease stability. However, most of the groups have made modified RNA sequences by incorporating 2′-fluoro pyrimidines to improve their nuclease stability.11,14 These modifications are incorporated during the in vitro transcription process by using suitable 2′-fluoro modified nucleoside triphosphates. The cells are pre-treated with yeast tRNA and bovine serum albumin in order to reduce background binding. After the final selection the enriched pool is usually cloned into Escherichia coli and sequenced.
Biomarker identification
DNA aptamers. Elucidation of differentially expressed protein markers on cell surface is of great clinical importance. It can not only help detecting and monitoring a disease, but also can help selecting a therapeutic approach as well.15,16 Combination of 2D gel electrophoresis and advanced mass spectrometry has made soluble biomarker identification easy these days. However, conventional biomarker identification method, involving surface protein extraction step using detergent, is still a challenge because it tends to diminish the signal from mass spectrometry.17 One of the great advantages of the cell-SELEX method is that it can identify new biomarkers, over expressed on a cell surface. Aptamer based biomarker identification by means of mass spectroscopic analysis of an unknown marker protein has been demonstrated by several groups in recent years (Table 1).7
Table 1 Cell-SELEX generated aptamers having been useful in biomarker identification (or validation) and targeted therapy
Selected aptamer |
Oligo-type |
Cell line |
Biomarker |
Delivery vehicle/targeting |
References |
Identified |
Validated |
Aptamer III.1 |
DNA |
YPEN-1 |
Mouse pigpen |
— |
— |
10 |
TN-9.4, TTA-1 |
2′-F-Py RNA, 2′-F-Py, 2′-OMe-Pu RNA |
U251 |
— |
Tenascin-C |
— |
10 |
GBI-10 |
DNA |
U251 |
Tenascin-C |
— |
|
12 |
Sgc8, Sgc8c |
DNA |
CCRF-CEM |
PTK-7 |
— |
Various therapeutic agent delivery |
13, 18 and 36–46 |
S6 |
RNA |
SK-BR-3 |
— |
HER-2 |
— |
26 |
TD05 |
DNA |
Ramos |
IGHM |
— |
Photosensitizer chlorine e6(c) and dye delivery |
19, 20, 47 and 48 |
D4 |
2′-F-Py RNA |
PC12 |
— |
RET |
Blocks RET-dependent intracellular signaling pathway |
24 |
A07 |
2′-F-Py RNA |
CHO |
— |
TbRIII |
Inhibits association of TGF-β2 with TbRIII |
25 |
Several |
DNA |
CD16α and c-Met |
— |
— |
Tumor cell lysis |
28 |
E10, E37 |
2′-F-Py RNA |
HET-SR-1(HM) |
— |
— |
Disrupt migration and invasion of tumor cells |
29 |
Several |
2′-F-Py RNA |
U87MG |
— |
— |
Inhibit intracellular signal transduction pathway |
30 |
TOV6 |
DNA |
TOV-21G |
STIP1 |
STIP1 |
Blocks cell invasion |
22 and 23 |
Daniels et al.12 have generated DNA aptamer, GBI-10, against U251 glioblastoma cell lines. They have then identified the GBI-10 binding counterpart on the extracellular matrix, tenascin-C, by means of affinity purification followed by LC MS/MS analysis. Tenascin-C is large glycoprotein that is highly expressed in the microenvironment of most solid tumors and potentially involved in tumorigenesis including metastasis and angiogenesis.
In another work, Blank et al.10 have identified a DNA aptamer (aptamer III.1) that binds to adenovirus-12 SV40-transformed YPEN-1 rat endothelial cell line. A ligand-mediated magnetic DNA affinity purification followed by mass spectrometry has successfully indentified an endothelial protein, rat homologue of mouse pigpen.
Shangguan et al.13 initially generated a DNA aptamer that binds to CCRF-CEM cells which are human precursor T-cell acute lymphoblastic leukemia cells. In the following work a potential biomarker of T-cell acute lymphoblastic leukemia was identified as PTK7 18 which is a transmembrane receptor tyrosine kinase-like molecule.
An immunoglobulin μ heavy chain (IGHM) is one of the major components of B-cell receptor complex expressed in Burkitt's lymphoma cells. A DNA aptamer, TD05, which was first selected by cell-SELEX method for Burkitt's lymphoma cell line Ramos,19 was later successfully utilized in identifying IGHM.20
There is also one report on DNA aptamer selection on HF and HA cell lines which are nontumorigenic revertants of HeLa cells and express the HPV E6 and E7 oncoproteins. However, these aptamers do not bind to the parental HeLa cells which is the HPV transformed cervical carcinoma cell and do not express the above mentioned oncoproteins.21 This indicates that the binding counterpart of these aptamers are absent on the surface of HPV transformed cervical carcinoma cell line. Therefore, as proposed by Graham et al., these aptamers could be used to identify biomarkers related to carcinogenesis.
In a more recent work Simaeys and co-workers have identified a stress-induced phosphoprotein 1 (STIP 1) as a marker cell surface protein for ovarian cancer cell line TOV-21G.22 They have utilized formaldehyde induced crosslinking of the DNA aptamer TOV 6 and its target protein and extraction of aptamer–protein complex for protein identification. This DNA aptamer was generated earlier by a cell-SELEX method done against TOV-21G, reported by the same group.23
Biomarker validation
RNA aptamers. Unlike the DNA aptamers, there are no reports on discovery of biomarkers by mass-spectroscopic and proteomics analysis utilizing RNA aptamers yet. However, there are reports on several biomarkers, found as the binding counterpart of the RNA aptamers (Table 1), confirmed by experimental evidences. For example, the D4 aptamer, generated by cell-SELEX method against RET expressing PC12 cells, was found to be binding to other RET expressing cell lines and also inhibited RET and ERK phosphorylation. This actually validates that the human RET receptor was the bona fide target of the aptamer D4.24Ohuchi et al. have generated 2′-F pyrimidine modified RNA aptamers against Chinese hamster ovary (CHO) cells which express transforming growth factor-β (TGF-β) type III receptor.25 Aptamer A07, generated from this study, was found to inhibit the association of TGF-β2 with TbRIII in vitro. This validates that the binding counterpart of aptamer A07 is indeed TbRIII receptor.
In another work Kang et al.26 have indentified S6 aptamer against HER-2 overexpressing SK-BR-3. They have validated HER-2 as the binding counterpart of S6 aptamer by measuring its affinity towards another HER-2 overexpressing cell line, NIH-3T3/HER2.
Targeted therapy
Several nucleic acid based approaches are known those are of great interest for therapeutic applications such as antisense oligonucleotides, DNAzymes, ribozymes, short hairpin RNA, short interfering RNA, micro RNA and aptamers. All these strategies though are efficient in knocking down a target mRNA, need a delivery carrier for bringing these therapeutic molecules into cytosol.27 Certain aptamers, on the other hand, has dual role of both targeting a specific receptor for cellular uptake and having a therapeutic effect.10,24,28–30 Several aptamers have been designed to deliver other cargo molecules such as siRNA, cytotoxin, chemotherapeutic agents, photodynamic therapeutic agents etc.27,31,32 and referencestherein,33
DNA aptamers. It is mentioned previously in the ‘Biomarker identification’ section that a DNA aptamer, sgc8, was identified for CCRF-CEM cells.13 This sgc8 aptamer not only bound to PTK7 expressed on CCRF-CEM cell membrane, it also got internalized.36 Later on, sgc8c aptamer was obtained by truncating the original sgc8 aptamer.37 This truncated sequence was covalently conjugated to doxorubicin (Dox), an anticancer drug, for a targeted therapeutic application in leukemia.38 The sgc8c–Dox conjugates were shown to be more cytotoxic towards CCRF-CEM cells than for promylocytic leukemia cell line NB-4. This cytotoxicity is comparable with the free Dox which suggests that sgc8c–Dox conjugates are selectively delivered to the CCRF-CEM cells. The parent sgc8 aptamer was also non-covalently conjugated to daunorubicin, in another work, and shown to deliver daunorubicin to T-cell acute lymphoblastic leukemia cells.39 There are several other studies where sgc8 and sgc8c are being exploited as drug delivery platform in the form of nanostructures,40,41 and in conjugation with G-quadruplex,42 drug-loaded liposome,43 Au–Ag nanorods,44,45 other aptamer.35,46Mallikaratchy et al. have used TD05 aptamer, selected against the Burkitt's lymphoma cell line Ramos,19 to deliver photosensitizer chlorin e6(c) for phototherapeutic targeting of tumor cells. They have found that the TD05–Ce6 complex was able to kill targeted Ramos cells by more than 50% over untargeted cells.47 In another report, TDO5–PEG–lipid conjugates were allowed to form micelle which was then found to increase the binding affinity by 750 fold and also to efficiently deliver a dye inside the cell.48 This platform, with increased affinity and delivery capability can further be exploited to deliver chemotherapeutic drugs as well.
Interestingly in 2011, Boltz and co-workers were successful in mediating tumor cell lysis by forming a chimeric DNA.28 They combined the aptamers selected against CD16α which is expressed on nature killer cells, with the aptamers obtained against c-Met that is over expressed in tumor cells. The CD16α is important in antibody-dependent cellular cytotoxicity whereas c-Met is involved in cancer proliferation, migration and invasion.
2′-F pyrimidine RNA aptamers. Cerchia et al. have generated nuclease-resistant 2′-F pyrimidine modified aptamers that recognize the extracellular domain of human receptor tyrosine kinase, RETC634Y, by targeting PC12 cells which are known to express this mutant receptor protein.24 Remarkably, one of these aptamers blocked RET-dependent intracellular signaling pathways by interfering with receptor dimerization.Aptamers selected by cell-SELEX method often binds to a protein expressed on the cell surface as evident from several marker discovery efforts. However, for a targeted therapy approach it is not always necessary to know the binding counterpart of the aptamer. Interestingly, Zueva et al. has developed aptamers, E10 and E37, by cell-SELEX method which can not only specifically recognize highly metastatic HET-SR-1 (HM) cell line, they can also disrupt migration and invasion of tumor cells.29 This group used HET-SR (LM) cell lines for counter selection which was similar to that of HM cells in terms of tumorigenicity and growth properties but of less metastatic potential compared to those of the HM cells. In a similar work done by Cerchia et al. the malignant human glioma cell line U87MG was specifically recognized over similar but poorly tumorigenic T98G cell line by a number of aptamers generated by cell-SELEX method.30 These aptamers were able to inhibit a specific intracellular signal transduction pathway too.
Diagnostic application
Imaging tool. Aptamers can be useful as diagnostic tool by easy attachment with fluorescent dyes or different nanoparticles suitable for detection and capture.31,34 A number of aptamers are screened for diagnostic application but many of them are actually done with the aptamers selected through a conventional SELEX procedure against peptide or protein and attempted for imaging and diagnostics in a real cellular system. The ones which are not evolved from a cell-SELEX method are not discussed here.An aptamer previously generated by a cell-SELEX method for CCRF-CEM cells, a T-cell acute lymphocytic leukemia cell line, was conjugated with gold nanoparticles to evaluate their potential for detection purpose. It was found that the aptamer conjugated to the gold nanoparticles could colorimetrically detect the target CCRF-CEM cells with high selectivity and sensitivity over those of the control Burkitt's lymphoma Ramos cells.49
In a very recent work Li et al. have identified DNA aptamer LXL-1 and truncated LXL-1-A, that bind selectively to metastatic breast cancer cells MDA-MB-231 with high affinity.50 They have utilized FAM-labeled LXL-1-A and found 76% detection rate against breast cancer tissue with metastasis in regional lymph nodes.
Wang et al. have developed a prostate cancer cell PC-3 specific DNA aptamer Wy-5a.51 They were able to stain and distinguish the sections from high risk group with metastatic characteristics from benign prostatic hyperlapsia by fluorescently labeling the Wy-5a. Even though an RNA aptamer for prostate specific membrane antigen (PSMA) is very well known and several groups have worked on aptamer-mediated drug delivery to PSMA expressing LNCaP cells utilizing the same,32 and referencestherein this recent work by Wang et al. is noteworthy for this shows the need of different molecular probes even for same cancer type and hence the utility of cell-SELEX for developing aptamers.
Two different groups have worked on two different cell types of glioblastoma to generate DNA aptamers and showed their application as imaging probes. The GBM128 and GBM131 were selected against human glioblastoma U118-MG cells by Kang et al.52 This group has further tested these aptamers on clinical glioma tissues and validated their binding specificity over normal brain tissues. In a recent work, Xidong Wu53 and co-workers have identified a DNA aptamer U2, against EGFRvIII overexpressing glioma U87-EGFRvIII cells where they have used U87MG cells as negative control. In this work they have investigated the tumor uptake of the 188Re-labelled aptamer in EGFRvIII overexpressing glioblastoma xenografts by radionuclide imaging.
Apart from the aforementioned aptamers, several others are generated by cell-SELEX method for various cancer cell types which can potentially be used for cancer diagnosis by imaging e.g., small cell lung cancer,54,55 non-small cell lung cancer,56 acute myeloid leukemia,57 colorectal cancer,58 liver cancer59 and cancer stem cells.60 Further applicability of these aptamers are yet to be demonstrated.
Enrichment and detection. Many a times early detection of rare cancer cells become difficult because for their low occurrence in the mixture of normal cells. Therefore, enrichment of those diseased cells is often needed before a successful detection.In an attempt to enrich the CCRF-CEM cells, Tan et al. have done a two nanoparticle assay with sgc8 aptamer.61 They have conjugated sgc8 aptamers separately with magnetic as well as fluorescent nanoparticles. Both of these nanoparticle conjugated aptamers were bound only to the target CCRF-CEM cells in a mixture of target and control cells. These aptamer bound target cells could then be extracted from the mixture by using magnetic field where as they could be detected by fluorescent microscopy or flow cytometry. It was also found that these magnetically captured cells when treated with fluorescent nanoparticle–aptamer conjugates, showed enhancement of detection efficiency by 100-folds.
They have further demonstrated the multiplex capability of this method by using three dye-doped nanoparticles with non-overlapping emission and excitation spectra.62 These three dye-doped nanoparticles were conjugated with three different aptamers specific for three different cell lines. Along side three sets of magnetic nanoparticle-aptamer conjugates were prepared by using three different aptamers. This two nanoparticle based assay could efficiently sort cells from a complex mixture.
In 2009 Phillips et al.63 used the same DNA aptamer mentioned above to capture and detect low concentration of the cancer cells. They have designed a microfluidic device in which the aptamer sgc8, linked to biotin at the 3′-end and fluorescein at the 5′-end, was immobilized within the channel. The surface of the channel then could capture target cells from a mixture of target and control cells in >80% capture efficiency and with 97% purity.
Aptamer logic-gates. Another problem in cancer cell detection is the accuracy. Many a time cancer cell-surface marker is also found to be present in normal cells in a smaller quantity. This can pose serious consequences for cancer therapy. A brilliant design, based on simultaneous multiple cell-surface marker identification, is proposed by Tan and co-workers recently.64,65 They have utilized more than one aptamer, selected against only one cancer cell type, to make Boolean logical operation systems. This method essentially exploits binding induced folding of aptamer. These aptamers are duplexed with rationally designed reporter sequences which get displaced and generate output signal once the aptamers are folded to bind to the target cell surface markers. More than one aptamer for one cell type, meaning more than one signal for one cell type, gives the accuracy of cancer cell detection. This logic-based programmable generalized system was not only utilized for accurate disease diagnosis, it was also used for photodynamic-based therapy by replacing the fluorescence reporter tag with chlorin e6, a photosensitizer. This design was found to have decreasing detection and therapeutic efficiency when tested for high-order logic operations at its present stage. However, with rational modifications such as introducing signal amplification method and different therapeutic modes will turn this advanced logic-based platform to a successful disease cell diagnostic and therapeutic technique, even for cells with complicated marker profiles.
Potential problems and advancements of cell-SELEX strategy
As cell-SELEX evolved from the traditional SELEX and has produced many promising diagnostic and therapeutic aptamers,66 it has further evolved to cell-internalization and in vivo SELEX to deal with potential problems associated with cell-SELEX. The problems and recent advancements are discussed in the following section.
Biostability and bioavailability. Like any nucleic acid based drug, aptamers also face problem of nuclease stability inside a cell when it comes to real application.67 Most of the aptamers selected through cell-SELEX method are either DNA oligos or 2′-F pyrimidine modified RNA oligos. There are two reports on 2′-OMe modified RNA as well.11,68 There are several other examples of aptamers made with small modified building blocks.14 However, small chemical modifications face rather big challenge in the SELEX process because of their poor recognition by polymerases. A recent work by Lucile and co-workers,69 on successful enzymatic incorporation of Locked Nucleic Acid (LNA) nucleotides in RNA has opened up the possibility of generating new class of LNA aptamers with better nuclease stability. However, these kinds of small modifications in the nucleotides may not protect the aptamers fully from biodegradation as overall low molecular weight of the aptamers subject them to quick renal clearance. Earlier works have shown that attaching the aptamers with high molecular weight molecules such as polyethylene glycol,70 and cholesterol70,71 slowed down the renal clearance of the aptamers.While some groups are engaged in improving the stability of the aptamers by means of different chemical modifications and finding out new suitable modifications, others have concentrated in finding out the most suitable candidate aptamer from well-established and widely used modifications i.e., 2′-F pyrimidine, 2′-OMe, by making changes in the selection strategy itself. Such methodological advancements are discussed below.
(i) Cell-internalization-SELEX. Aptamer based detection or imaging does not always need internalization of the aptamer but applications such as targeted drug delivery essentially needs the aptamer to be internalized. There are multiple reports on selected aptamers, found to be internalized by fluorescence based imaging. While expected, it is not guaranteed that the aptamers generated by cell-SELEX method will always get inside the cell. To overcome this problem several groups have focused on selecting aptamers which are internalized, not just surface bound by modifying the existing cell-SELEX method, namely, cell internalization-SELEX (Fig. 2).72–74 In this method both the unbound and surface-bound sequences are removed by harsh washing condition and only the internalized sequences are recovered after cell lysis with proteinase and detergent. Thus Wu et al.72 have identified DNA aptamers which are transported efficiently inside human chronic lymphocytic leukemia B cells (Table 2). In a similar approach Kolesnikova et al. have successfully generated tRNA derivatives that can be delivered inside isolated mitochondria.75 There are reports on selection of internalized aptamers performed on different non-cancer cell types i.e., vascular smooth muscle cell,76 TrkB-expressing HEK cells,77 HIV infected HEK293T78 and C8166 cells.79
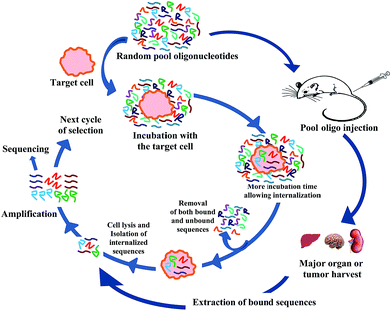 |
| Fig. 2 Schematic presentation of cell-internalization and in vivo SELEX procedure. | |
Table 2 Aptamers generated through cell-internalization SELEX and their application
Selected internalized aptamer |
Oligo-type |
Cell line |
Application |
References |
R10-60 |
DNA |
Ramos |
Increased internalization and immunostimulation |
80 |
DR15-8 |
Several |
2′-F-Py RNA |
N202.1A |
Si-RNA based targeting |
70 |
XEO2, XEO2 mini, XEO6, XEO6 mini, XEO9 |
2′-OMe-Py |
PC3 and LNCaP |
NP-mediated drug delivery |
68 |
More interestingly, Theil and co-workers80 have identified internalized RNA (2′-F pyrimidine modified) aptamers by modified cell-internalization SELEX method against HER2 over expressing mouse mammary carcinoma cells N202.1A. They have demonstrated that these aptamers could successfully be used to deliver siRNAs against Bcl-2 which is known to be over expressed in HER2+ cells. By successful delivery of the siRNA and thus silencing Bcl-2 they actually made the N202.1A cells sensitized toward cisplatin chemotherapy. These N202.1A cells were otherwise resistant toward chemotherapy because of Bcl-2 over expression (Table 2). While most of the modified RNA aptamers utilized in different selection methods are the 2′-F pyrimidine modified ones, Xiao et al.68 have tried 2′-OMe modified RNA (all except GTP were 2′-OMe modified triphosphates) for this purpose. They have not only generated multiple aptamers against PC3 and LNCaP cells, they have also demonstrated that these aptamers could enhance the therapeutic efficacy of docetaxel by efficiently carrying docetaxel loaded hybrid polymer NP inside the cell (Table 2).
(ii) In vivo selection. Cellular uptake efficacy of aptamer based drug carrier is not the only important parameter for intracellular availability of drug molecule. Several other pharmacokinetic parameters such as absorption, distribution, metabolism and excretion are also very important criterion and can well restrict the viability of a drug molecule for the clinical use. Very interestingly, Mi et al. have attempted to do aptamer selection on tumor cells of a living tumor mice model (Fig. 2).81 Intravenous injection of 2′-F pyrimidine modified RNA molecules into hepatic tumor implanted mice followed by liver tumor harvest and extraction of bound RNA sequences in 14 cycles have successfully generated candidate aptamer 14–16. This aptamer not only showed selective binding to tumor cell extract, but also showed localized binding to the intra-hepatic tumors when injected via tail vein. Peptide mass fingerprinting analysis determined that the aptamer 14–16 was bound to RNA helicase p68 which was known to be over expressed in colorectal cancer. While promising, generalizing this method for selecting aptamer specifically and distinguishably against other organs i.e., kidney, heart, lung etc. might be of great challenge. Of particular problem is getting the aptamers to brain. However, Cheng et al.82 have attempted to generate aptamers from 2′-F pyrimidine modified RNA pool which actually was bound to brain capillary endothelia and penetrated into the parenchyma of pool injected mice (injected in the tail vain). The mechanism of penetration is not yet clear at this point. Interestingly, though these aptamers were localized non-specifically in kidney and liver tissues, they were clearly found to be enriched in the brain tissue, presumably due to the fact that they get degraded sooner in kidney and liver.
(iii) Cell-SELEX with artificially expanded genetic information system (AEGIS). A library of nucleic acids with higher binding affinity and functionality together with greater stability than the pool of natural ones has always been of interest to the researchers of this field. Aptamers have been made with six different sugar-modified synthetic nucleic acid building blocks, collectively called xeno-nucleic acids (XNA)83 which improves the aptamer characteristics. Another class of functionally diverse aptamers, called SOMAmers (slow off-rate modified aptamers),84 has also emerged which utilizes chemically modified nucleobases. These modifications are inspied by amino-acid side chains and have produced aptamers against ‘difficult’ targets. Kimoto et al.85 have generated DNA aptamers containing hydrophobic unnatural base Ds which exclusively pairs with sterically complimentary unnatural base Px. However, Kimoto group have not maintained a standard SELEX protocol and incorporated the modified nucleotides in predetermined positions instead, due to the problem of amplification and sequencing of these modified oligonucleotides.A library containing artificially expanded genetic information system (AEGIS) is reported very recently by Sefah and co-workers.86 This work is remarkable in another respect that these unnatural AEGIS exploit base pairing as means of recognition, unlike the ones reported by Kimoto et al. or other expanded genetic alphabets.87 These modified nucleotides are independently replicable. They could successfully identify these AEGIS-aptamers with the help of next generation sequencing. This group has also successfully generated ZAP-2012 aptamer by cell-SELEX against breast cancer MDA-MB-231 cells.
High-throughput sequencing and bioinformatic analysis. The number of reports on aptamers against various cancer types has significantly increased after the introduction of cell-SELEX method. However, one of the biggest problems in this methodology especially for RNA based aptamers, is the monitoring of the selection progression. Introduction of next generation high-throughput sequencing (HTS) to the SELEX field has greatly improved the scenario. It has not only produced a wider spectrum of selected candidate aptamers, in combination with modern bioinformatic tools it has opened up the possibilities of much detailed analysis at each cycle of the selection.88,89 Thus next generation sequencing has opened up the possibility of generating the candidate aptamers at earlier cycles also.82,90 In recent years, several groups have applied this technique for analyzing and monitoring the selection progression in a cell-SELEX86,91 and cell-internalization SELEX.75,76 The great usefulness of this technique was evident particularly in the successful cell-SELEX done with AEGIS by Sefah et al.86 which was otherwise impossible as analyzing these modified aptamers would have been impossible, by conventional cloning.Thiel and co-workers76 have published predictive tools for identifying candidate aptamer from a list of selected aptamer sequences generated by HTS technique. They have brilliantly done both sequence-based and structure-based analysis by means of ‘edit distance’ and ‘tree distance’ analysis. They have also shown how HTS was utilized in each cycle for assessing fold enrichment which was key to monitor the selection progress.
Conclusion
In spite of its great potential, aptamers are not yet in the market as popular drug, targeting tool or diagnostic agent except for Macugen®. Recent advancements of aptamer selection method by directly targeting the diseased cells or even a mouse model show the immense potential of the aptamer based approach for identifying different cell surface markers. Though complex, performing a selection directly on a living cell has many advantages and increasing number of reports on aptamers against various cell types are coming up as the promise of the field. Yet most of the aptamers selected by this method are DNA oligos. The RNA aptamers, even with more structural diversity, face challenge for their low nuclease resistance inside a cellular media. The 2′-F pyrimidine modifications are currently in wide use to address this issue. Aptamers with other sugar-modified XNA nucleotides, locked nucleic acids (LNA) etc. have now come up with the possibilities to generate diverse RNA aptamers with nuclease stability. Recent report on cell-SELEX with artificially expanded genetic information system (AEGIS) has opened up the way to structurally and functionally diverse possibilities of aptamers. It is also important to generate the aptamers rapidly and essentially in a cost-effective manner for them to be available in the market for real application. High throughput sequencing technology together with modern bioinformatic analysis is now being applied successfully to reduce the number of selection cycle as well as finding best candidate aptamers. Thus, combination of recent advancements in biotechnology, nanotechnology and different detection methods together with advancements in the cell-internalization or in vivo SELEX technology is going to revolutionize the potential of chemotherapeutics and also the area of clinical diagnostics.
References
- E. Gullotti and Y. Yeo, Mol. Pharmaceutics, 2009, 6, 1041 CrossRef CAS PubMed.
- A. D. Ellington and J. W. Szostack, Nature, 1990, 346, 818 CrossRef CAS PubMed.
- C. Tuerk and L. Gold, Science, 1990, 249, 505 CAS.
- E. W. M. Ng, D. T. Shima, P. Calias, E. T. Cunningham Jr, D. R. Guyer and A. P. Adamis, Nat. Rev. Drug Discovery, 2006, 5, 123 CrossRef CAS PubMed.
- A. D. Keefy, S. Pai and A. Ellington, Nat. Rev. Drug Discovery, 2010, 9, 537 CrossRef PubMed.
- H. Sun, X. Zhu, P. Y. Lu, R. R. Rosato, W. Tan and Y. Zu, Mol. Ther.–Nucleic Acids, 2014, 3, e182 CrossRef CAS PubMed.
- M. Ye, J. Hu, M. Peng, J. Liu, J. Liu, H. Liu, X. Zhao and W. Tan, Int. J. Mol. Sci., 2012, 13, 3341 CrossRef CAS PubMed.
- L. Cerchia, F. Ducongé, C. Pestourie, J. Boulay, Y. Aissouni, K. Gombert, B. Tavitian, V. Franciscis and D. Libri, PLoS Biol., 2005, 3, e123 CrossRef PubMed.
- Y. Liu, C. Kuan, J. Mi, X. Zhang, B. M. Clary, D. D. Bigner and B. A. Sullenger, Biol. Chem., 2009, 390, 137 CAS.
- M. Blank, T. Weinschenk, M. Priemer and H. Schluesener, J. Biol. Chem., 2001, 276, 16464 CrossRef CAS PubMed.
- B. J. Hicke, C. Marion, Y. Chang, T. Gould, C. K. Lynott, D. Parma, P. G. Schmidt and S. Warren, J. Biol. Chem., 2001, 276, 48644 CrossRef CAS PubMed.
- D. A. Daniels, H. Chen, B. J. Hicke, K. M. Swiderek and L. Gold, Proc. Natl. Acad. Sci. U. S. A., 2003, 100, 15416 CrossRef CAS PubMed.
- D. Shangguan, Y. Li, Z. Tang, Z. C. Cao, H. W. Chen, P. Mallikaratchy, K. Sefah, C. J. Yang and W. Tan, Proc. Natl. Acad. Sci. U. S. A., 2006, 103, 11838 CrossRef CAS PubMed.
- M. Kuwahara and N. Sugimoto, Molecules, 2010, 15, 5423 CrossRef CAS PubMed.
- Y. M. Chang, M. J. Donovan and W. Tan, J. Nucleic Acids, 2013, 817350 Search PubMed.
- A. Cibiel, D. M. Dupont and F. Duconge, Pharmaceuticals, 2011, 4, 1216 CrossRef CAS PubMed.
- P. Dua, S. Kim and D. K. Lee, Methods, 2011, 54, 215 CrossRef CAS PubMed.
- M. Ye, J. Hu, M. Peng, J. Liu, J. Liu, H. Liu, X. Zhao and W. Tan, Int. J. Mol. Sci., 2012, 13, 3341 CrossRef CAS PubMed.
- Z. Tang, D. Shangguan, K. Wang, H. Shi, K. Sefah, P. Mallikratchy, H. W. Chen, Y. Li and W. Tan, Anal. Chem., 2007, 79, 4900 CrossRef CAS PubMed.
- P. Mallikaratchy, Z. Tang, S. Kwame, L. Meng, D. Shangguan and W. Tan, Mol. Cell. Proteomics, 2007, 6, 2230 CAS.
- J. C. Graham and H. Zarbl, PLoS One, 2012, 7, e36103 CAS.
- D. V. Simaeys, D. Lopez-Colon, K. Sefah, R. Sutphen, E. Jimenez and W. Tan, PLoS One, 2010, 5, e13770 Search PubMed.
- D. V. Simaeys, D. Turek, C. Champanhac, J. Vaizer, K. Sefah, J. Zhen, R. Sutphen and W. Tan, Anal. Chem., 2014, 86, 4521 CrossRef PubMed.
- L. Cerchia, F. Ducongé, C. Pestourie, J. Boulay, Y. Aissouni, K. Gombert, B. Tavitian, V. Franciscis and D. Libri, PLoS Biol., 2005, 3, e123 CrossRef PubMed.
- S. P. Ohuchi, T. Ohtsu and Y. Nakamura, Biochimie, 2006, 88, 897 CrossRef CAS PubMed.
- H. S. Kang, Y. M. Huh, S. Kim and D. Lee, Bull. Korean Chem. Soc., 2009, 30, 1827 CrossRef CAS.
- K. Germer, M. Leonard and X. Zhang, Int. J. Biochem. Mol. Biol., 2003, 4, 27 Search PubMed.
- A. Boltz, B. Piater, L. Toleikis, R. Guenther, H. Kolmar and B. Hock, J. Biol. Chem., 2011, 286, 21896 CrossRef CAS PubMed.
- E. Zueva, L. I. Rubio, F. Duconge and B. Tavitian, Int. J. Cancer, 2011, 128, 797 CrossRef CAS PubMed.
- L. Cerchia, C. L. Esposito, A. H. Jacobs, B. Tavitian and V. de Franciscis, PLoS One, 2009, 4, e7971 Search PubMed.
- J. G. Bruno, Pharmaceuticals, 2013, 6, 340 CrossRef CAS PubMed.
- J. Zhou and J. J. Rossi, Oligonucleotides, 2011, 21, 1 CrossRef CAS PubMed.
- P. Ray, M. A. Cheek, M. L. Sharaf, N. Li, A. D. Ellington, B. A. Sullenger, B. R. Shaw and R. R. White, Nucleic Acid Ther., 2012, 22, 295 CAS.
- Y. Zhang, Y. Chen, D. Han, I. Ocsoy and W. Tan, Bioanalysis, 2010, 2, 907 CrossRef CAS PubMed.
- G. Zhu, M. Ye, M. J. Donovan, E. Song, Z. Zhao and W. Tan, Chem. Commun., 2012, 48, 10472 RSC.
- Z. Xiao, D. Shangguan, Z. Cao, X. Fang and W. Tan, Chemistry, 2008, 14, 1769 CrossRef CAS PubMed.
- D. Shangguan, Z. Tang, P. Mallikaratchy, Z. Xiao and W. Tan, ChemBioChem, 2007, 8, 603 CrossRef CAS PubMed.
- Y. F. Huang, D. Shangguan, H. Liu, J. A. Phillips, X. Zhang, Y. Chen and W. Tan, ChemBioChem, 2009, 10, 862 CrossRef CAS PubMed.
- S. M. Taghdisi, K. Abnous, F. Mosaffa and J. Behravan, J. Drug Targeting, 2010, 18, 277 CrossRef CAS PubMed.
- S. M. Douglas, I. Bachelet and G. M. Church, Science, 2012, 335, 831 CrossRef CAS PubMed.
- Y.-L. Luo, Y.-S. Shiao and Y.-F. Huang, ACS Nano, 2011, 5, 7796 CrossRef CAS PubMed.
- K. Wang, M. You, Y. Chen, D. Han, Z. Zhu, J. Huang, K. Williams, C. J. Yang and W. Tan, Angew. Chem., Int. Ed., 2011, 50, 6098 CrossRef CAS PubMed.
- H. Kang, M. B. O'Donoghue, H. Liu and W. Tan, Chem. Commun., 2010, 46, 249 RSC.
- Y.-F. Huang, K. Sefah, S. Bamrungsap, H.-T. Chang and W. Tan, Langmuir, 2008, 24, 11860 CrossRef CAS PubMed.
- H. Kang, A. C. Trondoli, G. Zhu, Y. Chen, Y.-J. Chang, H. Liu, Y.-F. Huang, X. Zhang and W. Tan, ACS Nano, 2011, 5, 5094 CrossRef CAS PubMed.
- G. Zhu, L. Meng, M. Ye, L. Yang, K. Sefah, M. B. O'Donoghue, Y. Chen, X. Xiong, J. Huang, E. Song and W. Tan, Chem.–Asian J., 2012, 7, 1630 CrossRef CAS PubMed.
- P. Mallikaratchy, Z. Tang and W. Tan, ChemMedChem, 2008, 3, 425 CrossRef CAS PubMed.
- Y. Wu, K. Sefah, H. Liu, R. Wang and W. Tan, Proc. Natl. Acad. Sci. U. S. A., 2010, 107, 5 CrossRef CAS PubMed.
- C. D. Medley, J. E. Smith, Z. Tang, Y. Wu, S. Bamrungsap and W. Tan, Anal. Chem., 2008, 80, 1067 CrossRef CAS PubMed.
- X. Li, W. Zhang, L. Liu, Z. Zhu, G. Ouyang, Y. An, C. Zhao and C. J. Yang, Anal. Chem., 2014, 86, 6596 CrossRef CAS PubMed.
- Y. Wang, Y. Luo, T. Bing, Z. Chen, M. Lu, N. Zhang, D. Shangguan and X. Gao, PLoS One, 2014, 9, e100243 Search PubMed.
- D. Kang, J. Wang, W. Zhang, Y. Song, X. Li, Y. Zou, M. Zhu, Z. Zhu, F. Chen and C. J. Yang, PLoS One, 2012, 10, e42731 Search PubMed.
- X. Wu, H. Liang, Y. Tan, C. Yuan, S. Li, X. Li, G. Li, Y. Shi and X. Zhan, PLoS One, 2014, 9, e90752 Search PubMed.
- H. W. Chen, C. D. Medley, K. Sefah, D. Shangguan, Z. Tang, L. Meng, J. E. Smith and W. Tan, ChemMedChem, 2008, 3, 991 CrossRef CAS PubMed.
- T. Kunii, S. Ogura, M. Mie and E. Kobatake, Analyst, 2011, 136, 1310 RSC.
- Z. Zhao, L. Xu, X. Shi, W. Tan, X. Fang and D. Shangguan, Analyst, 2009, 134, 1808 RSC.
- K. Sefah, Z. W. Tang, D. H. Shangguan, H. Chen, D. Lopez-Colon, Y. Li, P. Parekh, J. Martin, L. Meng, J. A. Phillips, Y. M. Kim and W. Tan, Leukemia, 2009, 23, 235 CrossRef CAS PubMed.
- K. Sefah, L. Meng, D. L. Colon, E. Jimenez, C. Liu and W. Tan, PLoS One, 2010, 5, e14269 Search PubMed.
- D. Shangguan, L. Meng, Z. C. Cao, Z. Xiao, X. Fang, Y. Li, D. Cardona, R. P. Witek, C. Liu and W. Tan, Anal. Chem., 2008, 80, 721 CrossRef CAS PubMed.
- K. Sefah, K.-M. Bae, J. A. Phillips, D. W. Siemann, Z. Su, S. McClellan, J. Vieweg and W. Tan, Int. J. Cancer, 2013, 132, 2578 CrossRef CAS PubMed.
- J. K. Herr, J. E. Smith, C. D. Medley, D. Shangguan and W. Tan, Anal. Chem., 2006, 78, 2918 CrossRef CAS PubMed.
- J. E. Smith, C. D. Medley, Z. Tang, D. Shangguan, C. Lofton and W. Tan, Anal. Chem., 2007, 79, 3075 CrossRef CAS PubMed.
- J. A. Phillips, Y. Xu, Z. Xia, Z. H. Fan and W. Tan, Anal. Chem., 2009, 81, 1033 CrossRef CAS PubMed.
- M. You, L. Peng, N. Shao, L. Zhang, L. Qiu, C. Cui and W. Tan, J. Am. Chem. Soc., 2014, 136, 1256 CrossRef CAS PubMed.
- M. You, G. Zhu, T. Chen, M. J. Donovan and W. Tan, J. Am. Chem. Soc., DOI:10.1021/ja509263k.
- S. Ohuchi, BioRes. Open Access, 2012, 1, 265 CrossRef CAS PubMed.
- W. Tan, M. J. Donovan and J. Jiang, Chem. Rev., 2013, 113, 2842 CrossRef CAS PubMed.
- Z. Xiao, E. L. Nissenbaum, F. Alexis, A. Lupta, B. A. Teply, J. M. Chan, J. Shi, E. Digga, J. Cheng, R. Langer and O. C. Farokhzad, ACS Nano, 2012, 6, 696 CrossRef CAS PubMed.
- L. Crouzier, C. Dubois, S. L. Ewards, L. H. Lauridsen, J. Wengel and R. N. Veedu, PLoS One, 2012, 7, e35990 CAS.
- J. M. Healy, S. D. Lewis, M. Kurz, R. M. Boomer, K. M. Thompson, C. Wilson and T. G. McCauley, Pharm. Res., 2004, 21, 2234 CrossRef CAS.
- C. P. Rusconi, J. D. Roberts, G. A. Pitoc, S. M. Nimjee, R. R. White, G. Quick Jr, E. Scardino, W. P. Fay and B. A. Sullenger, Nat. Biotechnol., 2004, 22, 1423 CrossRef CAS PubMed.
- C. C. N. Wu, J. E. Castro, M. Motta, H. B. Cottam, D. Kyburz, T. J. Kipps, M. Corr and D. A. Carson, Hum. Gene Ther., 2003, 14, 849 CrossRef CAS PubMed.
- A. Yan and M. Levy, Methods Mol. Biol., 2014, 1103, 241 Search PubMed.
- W. H. Thiel, K. W. Thiel, K. S. Flenker, T. Bair, A. J. Dupuy, J. O. McNamara II, F. J. Miller and P. H. Giangrande, Methods Mol. Biol., 2015, 1218, 187 Search PubMed.
- O. Kolesnikova, H. Kazakova, C. Comte, S. Steinberg, P. Kamenski, R. P. Martin, I. Tarassov and N. Entelis, RNA, 2010, 16, 926 CrossRef CAS PubMed.
- W. H. Thiel, T. Bair, A. S. Peek, X. Liu, J. Dassie, K. R. Stockdale, M. A. Behlke, F. J. Miller Jr and P. H. Giangrande, PLoS One, 2012, 7, e43836 CAS.
- Y. Z. Huang, F. J. Hernandez, B. Gu, K. R. Stockdale, K. Nanapaneni, T. E. Scheetz, M. A. Behlke, A. S. Peek, T. Bair and P. H. Giangrande, et
al., Mol. Pharmacol., 2012, 82, 623 CrossRef CAS PubMed.
- N. van Bel, A. T. Das and B. Berkhout, J. Virol., 2014, 88, 1870 CrossRef PubMed.
- T. T. Baig, J. M. Lanchy and J. S. Lodmell, J. Virol., 2009, 83, 802 CrossRef CAS PubMed.
- K. W. Thiel, L. I. Hernandez, J. P. Dassie1, W. H. Thiel, X. Liu, K. R. Stockdale, A. M. Rothman, F. J. Hernandez, J. O. McNamara II and P. H. Giangrande, Nucleic Acids Res., 2012, 40, 6319 CrossRef CAS PubMed.
- M. Jing, Y. Liu, Z. N. Rabbani, Z. Yang, J. H. Urban, B. A. Sullenger and B. M. Clary, Nat. Chem. Biol., 2010, 6, 22 CrossRef PubMed.
- C. Cheng, Y. H. Chen, K. A. Lennox, M. A. Behlke and B. L. Davidson, Mol. Ther.–Nucleic Acids, 2013, 2, e67 CrossRef PubMed.
- V. B. Pinheiro, A. I. Taylor, C. Cozens, M. Abramov, M. Renders, S. Zhang, J. C. Chaput, J. Wengel, S. Peak-Chew, S. H. McLaughlin, P. Herdewijn and P. Holliger, Science, 2012, 336, 341 CrossRef CAS PubMed.
- L. Gold, D. Ayers, J. Bertino, C. Bock, A. Bock, E. N. Brody, J. Carter, A. B. Dalby, B. E. Eaton and T. Fitzwater, et al., PLoS One, 2010, 5, e15004 CAS.
- M. Kimoto, R. Yamashige, K. Matsunaga, S. Yokoyama and I. Hirao, Nat. Biotechnol., 2013, 31, 453 CrossRef CAS PubMed.
- K. Sefah, Z. Yang, K. M. Bradley, S. Hoshika, E. Jiménez, L. Zhang, G. Zhu, S. Shanker, F. Yue, D. Turek, W. Tan and S. A. Benner, Proc. Natl. Acad. Sci. U. S. A., 2014, 111, 1449 CrossRef CAS PubMed.
- D. Malyshev, Y. J. Seo, P. Ordoukhanian and F. E. Romesberg, J. Am. Chem. Soc., 2009, 131, 14620 CrossRef CAS PubMed.
- A. Ozer, J. M. Pagano and J. T. Lis, Mol. Ther.–Nucleic Acids, 2014, 3, e183 CrossRef CAS PubMed.
- T. Schutze, B. Wilhelm, N. Greiner, H. Braun, F. Peter, M. Morl, V. A. Erdmann, H. Lehrach, Z. Konthur, M. Menger, P. F. Arndt and J. Glokler, PLoS One, 2011, 6, e29604 Search PubMed.
- K. Szeto, D. R. Latulippe, A. Ozer, J. M. Pagano, B. S. White, D. Shalloway, J. T. Lis and H. G. Craighead, PLoS One, 2013, 8, e82667 Search PubMed.
- S. Meyer, J. P. Maufort, J. Nie, R. Stewart, B. E. McIntosh, L. R. Conti, K. M. Ahmad, H. T. Soh and J. A. Thomson, PLoS One, 2013, 8, e71798 CAS.
|
This journal is © The Royal Society of Chemistry 2015 |