DOI:
10.1039/C4RA11920G
(Paper)
RSC Adv., 2015,
5, 10326-10331
Solar light driven dye degradation using novel organo–inorganic (6,13-pentacenequinone/TiO2) nanocomposite†
Received
7th October 2014
, Accepted 5th January 2015
First published on 7th January 2015
Abstract
The coupled semiconductor photocatalysts of 6,13-pentacenequinone/TiO2 (PQ/Ti) with different weight ratios were prepared. The PQ/TiO2 nanocomposite was prepared by a simple wet impregnation method. The prepared catalyst was characterized with various spectroscopic methods. XRD confirms the monoclinic and anatase phase of PQ and TiO2, respectively. FE-SEM showed the sheet like morphology of PQ, whereas the coupled catalyst depicts the formation of a homogeneous layer of TiO2 on the PQ surface in all three compositions. Optical studies by diffuse reflectance UV-Visible absorbance spectroscopy (DRS) indicate the band gap of PQ and TiO2 around 2.8 and 3.2 eV respectively. The absorbance in the range of 450 nm was also observed in the case of the prepared coupled catalyst confirming the loading of PQ on TiO2. The photocatalytic activity of the coupled catalyst was studied by observing the degradation of methylene blue (MB) dye under visible light irradiation. As compare to the individual components, the coupled organo–inorganic catalyst showed higher photocatalytic activity towards MB degradation. The PQ/TiO2 nanocomposite having 0.2 wt% PQ showed the highest rate of MB degradation i.e. Kapp = 5.2 × 10−2 min−1.
1. Introduction
Nowadays, industries are growing tremendously in order to fulfill human requirements. But, on the other hand this growth results in an increase in air and water pollution.1 Day by day the problem of pollution shows a serious impact on the ecosystem as well as on human beings.2 Primarily, water pollution shows a severe impact on the aquatic and terrestrial organisms.3,4 Industries are unable to design pollution free processes so one has to develop an eco-friendly method for water treatment.5 Conventional physical, chemical and biological methods have been utilized for water treatment.6 These methods include biological digestion, coagulation, chemical oxidation, clay minerals, flocculation, membrane separation, ozonation and various adsorbents.7–14 From these, adsorption is a very effective and economical method for the purification of waste water.15 All these techniques require a long time span, use of organic solvents and some special types of reactors. Overall, these techniques have certain merits as well as demerits. The scientific community is in search of suitable techniques for pollution abatement.16 Among the various industries, the textile industry is a major source of water pollution.17 Various dyes like methylene blue, methyl orange, methyl red, Rhodamine 6B, Rose Bengal, crystal violet and Congo red have been regularly utilized in the textile industry and the colored waste water is directly release into the eco-system.18–25 Some of the dyes are carcinogenic, mutagenic and toxic for human beings when they come into contact with mankind.26 To avoid such serious hazards, the development of an effective method is needed to treat the polluted water.27,28
Recently, heterogeneous photocatalysis have been exploited effectively for environmental purification.29 Photocatalysis involves the use of semiconductor oxide/sulphide in presence of light.30 Upon illumination with appropriate wavelength of light, the electron from valence band goes in to the conduction band creating electron–hole pairs.31 The formed electron and hole react with surface adsorbed oxygen and water molecule generating superoxide anion and OH radical, respectively. These OH and superoxide radicals have higher oxidizing and reducing potentials respectively and have capability of reducing as well as oxidizing large number of compounds. Till today, various oxide/sulphide semiconductors have been used viz. TiO2, ZnO, WO3, SnO2, Fe2O3, CdS, ZnS, SnS2, CdIn2S4 etc.32–40 Among the studied catalyst, TiO2 gained enormous attention due to its higher chemical and biological stability, cost-effective and non-toxic nature.16,34 However the main drawback associated with TiO2 for photocatalytic process is its wide band gap (3.0 eV for rutile and 3.2 eV for anatase phase, respectively).37,39 TiO2 requires UV light (<390 nm) for generating electron–hole pairs. Many researchers have reported the various methods for enhancing the photocatalytic activity of TiO2.41 It includes transition metal doping, non-metal doping, loading of noble metals and changing the synthesis methods.16,17,32,35 Recently, uses of coupled semiconductor have been utilized to enhance the photocatalytic activity; it involves use of CdS, WS2, MoS2, Ta2O5, WO3 coupled with ZnO and TiO2 respectively.34,42–45 Of late, use of carbon nano tubes and graphene coupled with semiconductor oxides have been reported, it showed enhanced photocatalytic activity.46 Recently our group synthesized some photocatalyst showing the higher photocatalytic activity towards dye degradation and Hydrogen generation via H2O and H2S splitting. Also few reports are available mentioning the effective use of organic semiconductors coupled with inorganic materials; Shang et al. reported the poly-(3-thiopheneacetic acid) coated Fe3O4 as photocatalyst for the efficient photocatalytic disinfection of pathogenic bacteria under solar light irradiation.47–59 Herein, we have reported synthesis of coupled organo–inorganic semiconductor photocatalyst of 6,13-pentacenequinone/TiO2 (PQ/Ti). We have prepared PQ/TiO2 nanocomposite with different weight ratios of PQ ranging from 0.01, 0.1, and 0.2 wt% by simple wet impregnation method and studied its photocatalytic activity towards aqueous methylene blue degradation under visible light irradiation. The nanocomposite obtained is characterized thoroughly for its structural and optical study.
2. Experimental
2.1. Synthesis of PQ/TiO2 nanocomposite
For the synthesis of PQ/TiO2 nanocomposite, anatase TiO2 Aldrich make was used as such, and 6,13 pentacenequinone was prepared in our laboratory. The PQ was prepared by simple mixing of 1,4-cyclohexadione and ortho-pthalaldehyde in mortar and pestle with 1
:
2 ratio for 30 min. without any solvent. A stock solution of PQ was prepared in 100 mL chloroform containing 5 mg of PQ. Appropriate amount of this solution was stirred with TiO2 dispersed in chloroform in order to get the 0.01, 0.1 and 0.2 wt% PQ respectively. Moreover, this mixture was stirred for 10 h, the excess solvent was naturally evaporated, the obtained powder containing PQ/TiO2 organo–inorganic nanocomposites was dried at 50 °C for 4 h followed by grinding, and used as such for further analysis.
3. Photocatalytic activity measurement
For the photocatalytic activity measurement of the prepared nanocomposites, the 100 mL aqueous methylene blue (MB) having 10 ppm MB was stirred in 150 mL conical flask. To this solution 50 mg of nanocomposite was added and stirred under 400 W mercury vapour lamp irradiation. The mercury vapour lamp was kept vertically in the quartz tube, provided with the water circulation arrangement in order to minimize the heating effect due to IR radiation. At regular intervals of time, fixed amount of aqueous solution were taken from the flask, centrifuged and the UV-visible absorption spectrum of the clear solution recorded using a double beam spectrophotometer. The decrease in the absorbance value at 664 nm wavelength, corresponding to the typical peak for the absorption spectra of MB, was utilized to determine the extent of degradation of MB and the photocatalytic activity of the sample with respect to irradiation time.
4. Results and discussion
4.1. X-ray analysis
The formed nanocomposite was characterized by powder X-ray diffractometry (XRD) to study the crystalline formed phases PQ/TiO2 catalyst. The powder XRD patterns of the PQ/TiO2 nanocomposites are plotted in Fig. 1. The diffraction peaks at 2θ = 25.3, 37.8, 48, 53.9, 55° can be indexed to the (101), (004), (200), (105) and (211) crystal planes of anatase TiO2 (JCPDS no. 21-1272). XRD pattern of pure PQ indicates the formation of crystalline monoclinic phase, the diffraction peaks at 14.7, 23.6 and 27.7° matches with (012), (112) and (104) reflection planes, respectively (JCPDS no. 47-2123). The monoclinic phase of PQ was observed in all three prepared compositions with varying the PQ wt. ratios (0.01 to 0.2 wt%). Even though, the amount of PQ used is too small but due to its higher crystalline nature, PQ showed slight hump in the XRD pattern of PQ/TiO2 nanocomposites. This confirms the formation of PQ/TiO2 nanocomposite. The crystallite size of TiO2 in composite was observed to be 23 nm which is smaller than pristine TiO2 (46 nm).
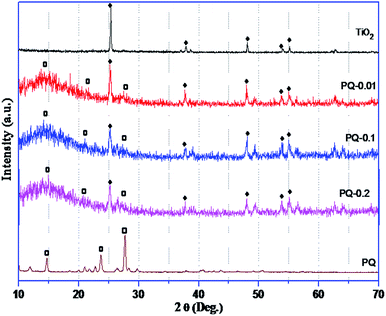 |
| Fig. 1 XRD pattern of photocatalyst (6,13-pentacenequinone/TiO2) where (♦) and (□) indicates peaks due to TiO2 and PQ respectively. | |
4.2. Morphological study by FE-SEM
FE-SEM micrographs of PQ/TiO2 nanocomposites are depicted in Fig. 2. The FESEM shows the uniform loading of TiO2 on PQ surfaces, particularly 0.01 and 0.1 wt% loading depicts the homogeneous distribution of PQ and TiO2 (Fig. 2a and d). Here, we expect loading of PQ on TiO2 surface but instead of PQ loading on TiO2 we obtained TiO2 loaded PQ nanocomposites.
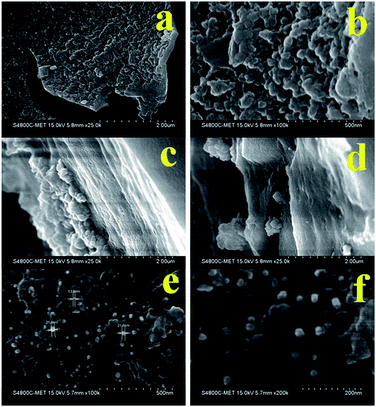 |
| Fig. 2 FESEM micrographs of coupled semiconductor photocatalyst (6,13-pentacenequinone/TiO2) where (a and b) 0.01 PQ; (c and d) 0.1 PQ and (e and f) 0.2 wt% PQ on TiO2 respectively. | |
This might be because of large flat surface (micron size) of PQ formation due to solid-state reaction technique. The dipole–dipole interaction among the individual PQ molecules lead the formation of micron sized plates. The existence of spherical nano sized TiO2 particles (10 to 25 nm) at the surface of PQ matrix is observed for higher PQ loading (Fig. 2e and f).
4.3. TEM analysis
The TEM analysis of PQ/TiO2 nanocomposite with 0.2 wt% PQ is depicted in Fig. 3.
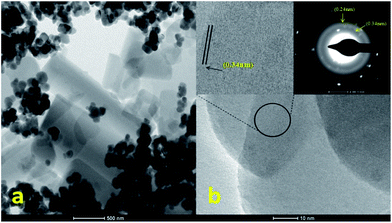 |
| Fig. 3 TEM micrograph of coupled semiconductor photocatalyst with 0.2 wt% PQ/TiO2 (6,13-pentacenequinone/TiO2), and SAED pattern PQ/TiO2 is shown in inset of (b). | |
The spherical shaped TiO2 particles having size in the range of 10–25 nm and sheet like PQ plates are clearly visible. The size of PQ plates is in the range of submicron size, having diameter around 200 to 500 nm and length around 2 to 3 μm. The homogeneous distributions of TiO2 particles on PQ plates are clearly visible. The high resolution image depicts the lattice spacing of 0.34 nm which corresponds to the (101) plane of anatase TiO2 (inset of Fig. 3b). The SAED pattern clearly indicates the (101) and (103) planes confirming presence of anatase TiO2 and this result supports the XRD analysis (inset of Fig. 3b).
4.4. UV-visible absorbance study
The diffuse reflectance UV-Visible absorbance spectra of PQ/TiO2 nanocompositeare shown in Fig. 4. The pristine PQ showed broad absorbance centered at 420 nm, and absorbance edge around 450 nm indicating band gap of 2.8 eV. Anatase TiO2 is having band gap of 3.2 eV. The prepared nanocomposites showed absorbance profiles same as anatase TiO2 however additional absorbance was observed around 425 nm. The presence of hump at 425 nm indicates the existence of PQ on TiO2 surface. Intensity of the PQ peak at 425 nm in the prepared nanocomposites increases with raise of PQ concentration (shown in inset of Fig. 4). Further, the PQ/TiO2 composite indicate blue shift in the absorbance profile as compare to pristine PQ and TiO2. This blue shift might be because of lowering of particle size of TiO2 (crystallite size 23 nm) during the synthesis of PQ/TiO2 nanocomposites due to vigorous stirring.
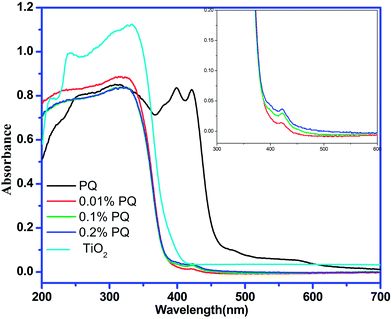 |
| Fig. 4 DRS spectra of coupled semiconductor photocatalyst (6,13-pentacenequinone/TiO2). | |
To validate the possible interaction between PQ and TiO2, we have analysed the PQ/TiO2 composites with FTIR. The C
O stretching frequency in PQ was observed around 1672 cm−1.47 In case of prepared PQ/TiO2 composites the same C
O stretching frequency observed at 1697 cm−1. This shift in frequency clearly indicates the formation of PQ/TiO2 composites. This suggests the possible interaction of carbonyl group of PQ with TiO2.
4.5. Photocatalytic MB degradation
After completing the structural analysis of PQ/TiO2 organo–inorganic nanocomposites the photocatalytic activity of samples was studied by degrading the 100 mL aqueous solution of 10 ppm MB under illumination of 400 W mercury vapour lamp using 50 mg of catalyst. The graph of % MB remained as functions of irradiation time are depicted in Fig. 5.
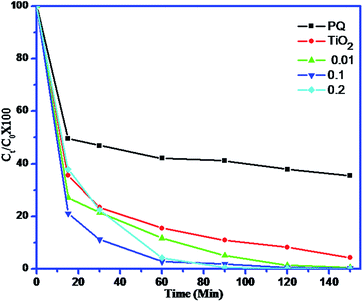 |
| Fig. 5 Graph of percent MB remained vs. irradiation time over PQ/TiO2 coupled photocatalyst. | |
The PQ degrades almost 60% of MB within 90 min. of irradiation while pure TiO2 degrades 90% respectively. The prepared nanocomposite showed degradation of MB almost higher than 95%, especially, PQ loaded with 0.2 wt% showed 99.5% MB degradation within 90 min. higher among the prepared compositions.
The PQ/TiO2 nanocomposite shows enhanced photocatalytic activity; this increase in activity was due to the effective electron–hole separation due to coupling of PQ and TiO2 catalysts and also increases in the absorbance range of catalyst in the visible region. In order to study the kinetics of photocatalytic MB degradation, the graph of ln
Co/Ct vs. irradiation time are plotted and shown in Fig. 6. It is well known that the photocatalytic dye degradation reaction follows Langmuir–Hinshelwood model and slope of the graph ln
Co/Ct vs. irradiation time gives the apparent rate constant (Kapp). In our case, we observed the highest apparent rate constant value for PQ/TiO2 nanocomposite having 0.2 wt% PQ. The observed rate constant for the MB degradation is Kapp = 5.2 × 10−2 min−1. The rate constant values are depicted in Table 1. To investigate the stability of the PQ/TiO2 photocatalyst, we also carried out the recycle study and found almost same degradation even after 5 recycles. The detailed recycle study is given in ESI, Fig. S2.†
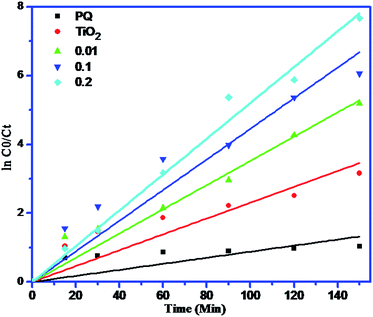 |
| Fig. 6 Kinetic plot of photocatalytic MB degradation vs. irradiation time over PQ/TiO2 coupled photocatalyst. | |
Table 1 Rate constant values of photocatalytic MB degradation reaction using PQ/TiO2 coupled photocatalyst
Sr. no. |
Name of the catalyst |
Rate (Kapp) (min−1) |
Standard error |
1 |
PQ |
0.88 × 10−2 |
0.00164 |
2 |
TiO2 |
2.30 × 10−2 |
0.00222 |
3 |
0.01 wt% PQ/TiO2 |
3.50 × 10−2 |
0.00174 |
4 |
0.10 wt% PQ/TiO2 |
4.40 × 10−2 |
0.00302 |
5 |
0.20 wt% PQ/TiO2 |
5.20 × 10−2 |
0.00149 |
The detailed photocatalytic mechanism of MB degradation using PQ/TiO2 nanocomposites is shown in Fig. 7.
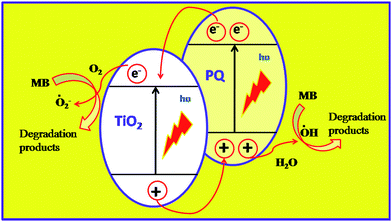 |
| Fig. 7 Mechanism of photocatalytic MB degradation over PQ/TiO2 coupled photocatalyst. | |
Upon illumination with light the electron–hole pairs are produced on the semiconductor surface, both the catalyst able to generate the electron–hole pairs. The PQ absorbs visible light and generated conduction band electron transfer to the conduction band of TiO2. Further, we studied the stability of PQ/TiO2 catalyst. For this purpose, 50 mg of PQ/TiO2 was stirred in 100 mL DI water for 12 h, then the catalyst separated using centrifugation and UV-Visible spectra of supernatant solution was taken to check the presence of PQ in the solution (ESI, Fig. S1†). The linear spectrum in the range of 250 to 800 nm confirms that the leaching of PQ was not occurring which confirms the stability of catalyst.
This process lowers the possibility of electron–hole pair recombination and enhances the rate of degradation. Due to this reason the nanocomposite catalyst shows higher activity as compare to pure catalyst.
5. Conclusions
The PQ/TiO2 nanocomposite was successfully synthesized via simple wet-impregnation having PQ/Ti ratios (0.01, 0.1, 0.2 wt%). XRD indicates the presence of monoclinic phase of PQ in the prepared organo–inorganic nanocomposites with anatase TiO2. DRS clearly show absorbance in the visible region because of PQ. FE-SEM of prepared nanocomposites indicates the homogeneous distribution of TiO2 in PQ matrix. The photocatalytic activity was evaluated by following the degradation of MB and found to be more for PQ/TiO2 nanocomposite having 0.2 wt% PQ. The highest apparent rate constant among the prepared catalyst is 5.2 × 10−2 min−1 using PQ/TiO2 catalyst having 0.2 wt% PQ. The proposed method can also be used to synthesize other semiconductor–PQ nanocomposites.
Acknowledgements
One of the authors Mr Vikram U. Pandit gratefully acknowledges Council of Scientific and Industrial Research (CSIR), New Delhi, India for financial support. Authors are grateful to Dr D. P. Amalnerkar, Executive Director, C-MET, for providing the facilities and useful discussion.
References
- J. C. Colmenares and R. Luque, Chem. Soc. Rev., 2014, 43, 765 RSC.
- R. L. Naylor, R. J. Goldburg, J. H. Primavera, N. Kautsky, M. C. Beveridge, J. Clay, C. Folke, J. Lubchenco, H. Mooney and M. Troell, Nature, 2000, 405, 1017 CrossRef CAS PubMed.
- K. P. Singh, S. Gupta, A. Kumar and D. Mohan, Chem. Res. Toxicol., 2014, 27, 741 CrossRef CAS PubMed.
- A. M. Nyman, K. Schirmer and R. Ashauer, Environ. Sci. Technol., 2014, 48, 5946 CrossRef CAS PubMed.
- K. D. Bladon, M. B. Emelko, U. Silins and M. Stone, Environ. Sci. Technol., 2014, 48, 8936 CrossRef CAS PubMed.
- T. J. Morgan and R. Kandiyoti, Chem. Rev., 2014, 114, 1547 CrossRef CAS PubMed.
- C. Higman and S. Tam, Chem. Rev., 2014, 114, 1673 CrossRef CAS PubMed.
- B. Acemiocglu, J. Colloid Interface Sci., 2004, 274, 371 CrossRef PubMed.
- C. Namasivayam and D. Kavitha, Dyes Pigm., 2002, 54, 47 CrossRef CAS.
- D. T. Sponza and H. Celebi, Bioresour. Technol., 2012, 104, 100 CrossRef CAS PubMed.
- M. Isik and D. T. Sponza, Bioresour. Technol., 2005, 96, 633 CrossRef CAS PubMed.
- R. Molinari, F. Pirillo, M. Falco, V. Loddo and L. Palmisano, Chem. Eng. Process., 2004, 43, 1103 CrossRef CAS PubMed.
- U. Bali, Dyes Pigm., 2004, 60, 187 CrossRef CAS.
- T. LaPara, C. Nakatsu, L. Pantea and J. Alleman, Water Res., 2001, 35, 4417 CrossRef CAS.
- Y. Liu, Y. Zheng and A. Wang, J. Environ. Sci., 2010, 22, 486 CrossRef CAS.
- K. Vinodgopal, D. E. Wynkoop and P. V. Kamat, Environ. Sci. Technol., 1996, 30, 1660 CrossRef CAS.
- N. L. Stock, J. Peller, K. Vinodgopal and P. V. Kamat, Environ. Sci. Technol., 2000, 34, 1747 CrossRef CAS.
- K. Bhattacharyya, J. Majeed, K. K. Dey, P. Ayyub, A. K. Tyagiand and S. R. Bharadwaj, J. Phys. Chem. C, 2014, 118, 15946 CAS.
- J. Gong, J. Liu, X. Chen, Z. Jiang, X. Wen, E. Mijowska and T. Tang, J. Mater. Chem. A, 2014, 2, 7461 CAS.
- Y. Xie, B. Yan, H. Xu, J. Chen, Q. Liu, Y. Deng and H. Zeng, ACS Appl. Mater. Interfaces, 2014, 6, 8845 CAS.
- N. Baliarsingh, K. M. Parida and G. C. Pradhan, Ind. Eng. Chem. Res., 2014, 53, 3834 CrossRef CAS.
- L. Zhang and J. M. Cole, ACS Appl. Mater. Interfaces, 2014, 6, 3742 CAS.
- J. X. Jiang, Y. Li, X. Wu, J. Xiao, D. J. Adams and A. I. Cooper, Macromolecules, 2013, 46, 8779 CrossRef CAS.
- K. Mohanty, J. T. Naidu, B. C. Meikap and M. N. Biswas, Ind. Eng. Chem. Res., 2006, 45, 5165 CrossRef CAS.
- S. Lan, L. Liu, R. Li, Z. Leng and S. Gan, Ind. Eng. Chem. Res., 2014, 53, 3131 CrossRef CAS.
- G. Fang, Y. Wu, X. Dong, C. Liu, S. He and S. Wang, J. Agric. Food Chem., 2013, 61, 3834 CrossRef CAS PubMed.
- A. Basu and G. S. Kumar, J. Agric. Food Chem., 2014, 62, 7955 CrossRef CAS PubMed.
- Y. Zhu, Y. M. Wang, S. Y. Zhao, P. Liu, C. Wei, Y. L. Wu, C. K. Xia and J. M. Xie, Inorg. Chem., 2014, 53, 7692 CrossRef CAS PubMed.
- M. V. Rao, K. Rajeshwar, V. R. Vernerker and J. Dubow, J. Phys. Chem., 1980, 84, 1987 CrossRef CAS.
- A. Mills, R. H. Davies and D. Worsley, J. Chem. Soc. Rev., 1993, 22, 417 RSC.
- A. Mills, S. Morns and R. Davies, J. Photochem. Photobiol., A, 1993, 70, 183 CrossRef CAS.
- Z. Wen, W. Wu, Z. Liu, H. Zhang, J. Li and J. Chen, Phys. Chem. Chem. Phys., 2013, 15, 6773 RSC.
- L. Li, X. L. Liu, M. Gao, W. Hong, G. Z. Liu, L. Fan, B. Hu, Q. H. Xia, L. Liu, G. W. Song and Z. S. Xu, J. Mater. Chem. A, 2014, 2, 1795 CAS.
- J. S. Jang, H. G. Kim, P. H. Borse and J. S. Lee, Int. J. Hydrogen Energy, 2007, 32, 4786 CrossRef CAS PubMed.
- J. Zhang, B. Li, W. Yang and J. Liu, Ind. Eng. Chem. Res., 2014, 53, 10629 CrossRef CAS.
- L. Zhou, Y. Shao, J. Liu, Z. Ye, H. Zhang, J. Ma, Y. Jia, W. Gao and Y. Li, ACS Appl. Mater. Interfaces, 2014, 6, 7275 CAS.
- J. Lu, P. Zhang, A. Li, F. Su, T. Wang, Y. Liu and J. Gong, Chem. Commun., 2013, 49, 5817 RSC.
- Y. Fang, Z. Li, B. Yang, S. Xu, X. Hu, Q. Liu, D. Han and D. Lu, J. Phys. Chem. C, 2014, 118, 16113 CAS.
- L. A. Nole, S. B. Ruiz, T. L. Pineda, O. P. Perez and F. R. Roman, J. Mater. Chem. A, 2013, 1, 5509 Search PubMed.
- Y. Zhang, R. Selvaraj, M. Sillanpaa, Y. Kim and C. W. Tai, Ind. Eng. Chem. Res., 2014, 53, 11720 CrossRef CAS.
- C. Lin, Y. Song, L. Cao and S. Chen, Nanoscale, 2013, 5, 4986 RSC.
- W. Ho, J. C. Yu, J. Lin, J. Yu and P. Li, Langmuir, 2004, 20, 5865 CrossRef CAS.
- M. Miyauchi, A. Nakajima, T. Watanabe and K. Hashimoto, Chem. Mater., 2002, 14, 4714 CrossRef CAS.
- P. Zhang, J. Zhang and J. Gong, Chem. Soc. Rev., 2014, 43, 4395 RSC.
- N. Huo, Q. Yue, J. Yang, S. Yang and J. Li, ChemPhysChem, 2013, 14, 4069 CrossRef CAS PubMed.
- Y. Qu and X. Duan, Chem. Soc. Rev., 2013, 42, 2568 RSC.
- V. U. Pandit, S. S. Arbuj, U. P. Mulik and B. B. Kale, Environ. Sci. Technol., 2014, 48, 4178 CrossRef CAS PubMed.
- K. Shang, B. Sun, J. Sun, J. Li and S. Ai, New J. Chem., 2013, 37, 2509 RSC.
- Y. Park and R. C. Advincula, Chem. Mater., 2011, 23, 4273 CrossRef CAS.
- H. Park, M. Gutierrez, X. Wu, W. Kim and X. Y. Zhu, J. Phys. Chem. C, 2013, 117, 10974 CAS.
- Y. Guo, Y. Li, J. Xu, X. Liu, J. Xu, J. Lv, C. Huang, M. Zhu, S. Cui, L. Jiang, H. Liu and S. Wang, J. Phys. Chem. C, 2008, 112, 8223 CAS.
- S. Zhang, R. Sakai, T. Abe, T. Iyoda, T. Norimatsu and K. Nagai, ACS Appl. Mater. Interfaces, 2011, 3, 1902 CAS.
- T. Kida, R. Oshima, K. Nonaka, K. Shimanoe and M. Nagano, J. Phys. Chem. C, 2009, 113, 19986 CAS.
- K. Maeda, K. Sekizawa and O. Ishitani, Chem. Commun., 2013, 49, 10127 RSC.
- R. Abe, K. Shinmei, K. Hara and B. Ohtani, Chem. Commun., 2009, 3577 RSC.
- K. E. deKrafft, C. Wang and W. Lin, Adv. Mater., 2012, 24, 2014 CrossRef CAS PubMed.
- S. Yang, H. Yang, H. Ma, S. Guo, F. Cao, J. Gong and Y. Deng, Chem. Commun., 2011, 47, 2619 RSC.
- R. Liu, M. Zhang, G. W. McPherson and W. Tang, Chem. Commun., 2013, 49, 4376 RSC.
- S. Yang, Q. Yue, F. Wu, N. Huo, Z. Chen, J. Yang and J. Li, J. Alloys Compd., 2014, 597, 91 CrossRef CAS PubMed.
Footnote |
† Electronic supplementary information (ESI) available: Fig. S1. Leaching study of PQ/TiO2 coupled photocatalyst. See DOI: 10.1039/c4ra11920g |
|
This journal is © The Royal Society of Chemistry 2015 |