DOI:
10.1039/C4RA08589B
(Paper)
RSC Adv., 2015,
5, 5580-5590
I2 catalyzed tandem protocol for synthesis of quinoxalines via sp3, sp2 and sp C–H functionalization†
Received
13th August 2014
, Accepted 8th December 2014
First published on 10th December 2014
Abstract
One-pot, atom-economic synthesis of quinoxalines has been achieved through generation of arylglyoxal from easily available ethylarenes, ethylenearenes and ethynearenes, and subsequent condensation with o-phenylenediamines. Catalytic I2 with TBHP as an oxidant in DMSO is the system of choice for this domino reaction involving C–H functionalization/oxidative cyclization. This metal-free, mechanistically distinct and functional group tolerant tandem approach could be a powerful complement to traditional approaches for the synthesis of quinoxalines.
Introduction
Quinoxaline is a widely explored nitrogen heterocyclic compound owing to its presence in numerous compounds and has promising applications in dyes,1,2 organic semiconductors,3 electroluminescent materials4 and anionic receptors.5 Furthermore, quinoxaline derivatives exhibit a broad range of biological activities such as antiviral, antibacterial, anti-inflammatory, anti-protozoal and kinase inhibitors.6 In addition, these are used as anticancer, antifungal, insecticidal and anthelmintic agents.7 Notably, quinoxaline is a key constituent of antibiotics such as echinomycin, levomycin and actinomycin.8 Quinoxaline derivatives also have promising applications in cavitands, chemically controllable switches and DNA cleaving agents.9
In view of the wide range of applications, various methods have been developed for the synthesis of quinoxaline derivatives. A highly explored method amongst these is the acid catalyzed condensation of 1,2-diaminobenzenes with 1,2-dicarbonyl compounds.10 Quinoxaline derivatives are also prepared by reaction of 1,2-diaminobenzenes with α-hydroxy ketones,11 epoxides,12 α-haloketones,13 α-tosyloxy ketones,14 α-ketocarboxylic acids,15 oxalic acid,16 diazenyl butenes,17 hydroxy acetylenes,18 alkynes,19 vicinal diols20 or diazoketones.21 A wide range of catalysts, e.g. molecular sieves,22 HClO4/SiO2,23 β-cyclodextrin,24 Me3SiCl,25 DABCO,26 transition metals (Mn, Ru, Pd and Cu),27 Ga(ClO4)3,28 ion-exchanged molybdophosphoric acid,29 KF-alumina30 and microwave irradiation31 have been employed, for these reactions.
However, many of these catalysts cannot be widely used owing to environmental and/or economical issues, including requirement of heavy metal catalysts, use of stoichiometric amounts, an excess amount of base, high temperature, incompatibility with functionalized substrates, long reaction time, low yield of products and multi step synthesis.
Therefore, development of economically viable procedures for the synthesis of quinoxaline derivatives from easily available multiform starting materials still continues to be a challenging task. In this context, summarizing the practical advantages of domino strategy of synthesis and continuation in our efforts towards development of facile, efficient, environment and eco-friendly synthetic protocols,32 herein we describe the synthesis of quinoxalines using I2/TBHP and DMSO in one step from ethylarenes (1), ethylenearenes (4) and ethynearenes (5) (Scheme 1). The method encompasses three primary processes-C–H functionalization, oxidation and condensation.
 |
| Scheme 1 Tandem metal-free synthesis of quinoxalines from ethylarenes or ethylenearenes or ethynearenes and o-phenylenediamines. | |
We first studied the action of I2/TBHP and DMSO on ethylbenzene (1a) for C–H functionalization and generation of phenylglyoxal, followed by in situ condensation with o-phenylenediamine.
Initially, the reaction of ethylbenzene (1a) with 1.0 equiv. I2 and 2.0 equiv. TBHP as an oxidant was carried out at 80 °C temperature for 1.0 h. o-Phenylenediamine (1.0 equiv.) in DMSO (3.0 mL) was added and heating was continued for 2.0 h to afford quinoxaline (3aa) in 28% yield (Table 1, entry 1). Use of 3.0 equiv. of TBHP resulted in slightly higher yield (39%, Table 1, entry 2). The reaction was then carried out at 100 and 120 °C, whereupon yields of 54 and 67% were obtained (Table 1, entries 3 and 4), thereby indicating a significant role played by temperature in this reaction. We next varied the iodine concentration from 0.5 equiv. to 0.1 equiv. in DMSO (3.0 mL) as a solvent with 3.0 equiv. of TBHP at 120 °C. The reaction with 0.5 equiv. of I2 led to 75% yield of product (Table 1, entry 5), whereas a higher yield of 84% was achieved with 0.3 equiv. of I2 (Table 1, entry 6). Use of 0.2 equiv. of I2 and 3.0 equiv. of TBHP in DMSO (3.0 mL) at 120 °C was found to be optimal resulting in 90% yield (Table 1, entry 7). A slight drop in the yield (85%) was observed when I2 concentration was reduced to 0.1 equiv. (Table 1, entry 8). In order to optimize the time required for formation of α-iodo acetophenone (B) from ethylbenzene (1a), few experiments (Table 1, entries 9–12) were carried out with varying time by using 0.2 equiv. of I2 and 3.0 equiv. of TBHP. The reaction time of 1.0 h was found to be the optimal time for this conversion. Similarly, few reactions (Table 1, entries 13–15) were performed to optimize the time needed for conversion of ethylbenzene (1a) to phenylglyoxal (C) with DMSO as a solvent. It was observed that reaction took 2.0 h for completion and formed phenylglyoxal (C) in 90% yield. Beside this we conducted some experiments (Table 1, entry 16–20) for optimization of the time necessary to form quinoxaline (3aa) after addition of o-phenylenediamine (2a) in DMSO. It was noticed that the reaction was completed in 2.0 h to afford quinoxaline (3aa) in 90% yield. We also tried simultaneous addition of all reaction components but it afforded the product only in trace amount thus proving the importance of sequential addition of the reactants (Table 1, entry 21). The other common oxidants namely DTBP, IBX, DMP, DIB, HTIB, DDQ, K2S2O8 and molecular O2 were also screened, but TBHP was found to be the most effective oxidant for this transformation (Table 1, entries 22–29). The reaction in absence of I2 was also attempted but it failed to give the desired product thereby highlighting the key role of I2 as a catalyst in this reaction (Table 1, entry 30). We screened catalysts such as NIS, KI, CuI and TBAI but all of these attempts resulted in low yields (19 to 35%, Table 1, entries 31–34). I2, therefore, is the most efficient catalyst for this reaction. No product was obtained when we conducted the reaction by using solvents such as DMF, 1,4-dioxane, THF, and toluene. These results confirm the dual role of DMSO in this reaction, as a solvent and a co-oxidant (Table 1, entries 35–38).
Table 1 Optimization of reaction conditions for tandem metal-free synthesis of quinoxaline from ethylbenzene and o-phenylenediaminea

|
Entry |
Catalyst (equiv.) |
Oxidant (equiv.) |
Solvent |
Time (h) |
Temp. (°C) |
Yieldg (%) |
Reaction conditions: 1a (1.0 mmol), catalyst and oxidant was heated for 1.0 h in sealed tube, 2a (1.0 mmol) in solvent (3.0 mL) was added and heating continued for 2.0 h. Reaction conditions: 1a (1.0 mmol), I2 (0.2 mmol) and TBHP (3.0 mmol) was heated for 0.5–2.0 h in sealed tube. Reaction conditions: 1a (1.0 mmol), I2 (0.2 mmol), TBHP (3.0 mmol) and DMSO (3.0 mL) was heated for 1.0–3.0 h in sealed tube. Reaction conditions: 1a (1.0 mmol), I2 (0.2 mmol) and TBHP (3.0 mmol) was heated for 1.0 h in sealed tube, 2a (1.0 mmol) in DMSO (3.0 mL) was added and heating continued for 0.5–3.0 h. Reaction condition: 1a (1.0 mmol), 2a (1.0 mmol), I2 (0.2 mmol) and TBHP (3.0 mmol) was heated in DMSO (3.0 mL) in sealed tube at 120 °C for 3.0 h. Reaction condition: 1a (1.0 mmol) and TBHP (3.0 mmol) was heated in sealed tube at 120 °C for 1.0 h, 2a (1.0 mmol) in DMSO (3.0 mL) was added and heating continued for 2.0 h. Isolated yield. DTBP = di-tert-butyl peroxide, IBX = o-iodoxy-benzoic acid, TBHP = tert-butyl hydroperoxide, DMP = 1,1,1-triacetoxy-1,1-dihydro-1,2-benziodoxol-3(1H)-one, DIB = (diacetoxyiodo)benzene, HTIB = [hydro(tosyloxy)iodo]benzene, DDQ = 2,3-dichloro-5,6-dicyano-1,4-benzoquinone, K2S2O8 = potassium persulfate. |
1 |
I2 (1.0) |
TBHP (2) |
DMSO |
3.0 |
80 |
28 |
2 |
I2 (1.0) |
TBHP (3) |
DMSO |
3.0 |
80 |
39 |
3 |
I2 (1.0) |
TBHP (3) |
DMSO |
3.0 |
100 |
54 |
4 |
I2 (1.0) |
TBHP (3) |
DMSO |
3.0 |
120 |
67 |
5 |
I2 (0.5) |
TBHP (3) |
DMSO |
3.0 |
120 |
75 |
6 |
I2 (0.3) |
TBHP (3) |
DMSO |
3.0 |
120 |
84 |
7 |
I2 (0.2) |
TBHP (3) |
DMSO |
3.0 |
120 |
90 |
8 |
I2 (0.1) |
TBHP (3) |
DMSO |
3.0 |
120 |
85 |
9 |
I2 (0.2)b |
TBHP (3) |
— |
0.5 |
120 |
63 |
10 |
I2 (0.2)b |
TBHP (3) |
— |
1.0 |
120 |
95 |
11 |
I2 (0.2)b |
TBHP (3) |
— |
1.5 |
120 |
76 |
12 |
I2 (0.2)b |
TBHP (3) |
— |
2.0 |
120 |
57 |
13 |
I2 (0.2)c |
TBHP (3) |
DMSO |
1.0 |
120 |
71 |
14 |
I2 (0.2)c |
TBHP (3) |
DMSO |
2.0 |
120 |
90 |
15 |
I2 (0.2)c |
TBHP (3) |
DMSO |
3.0 |
120 |
82 |
16 |
I2 (0.2)d |
TBHP (3) |
DMSO |
0.5 |
120 |
45 |
17 |
I2 (0.2)d |
TBHP (3) |
DMSO |
1.0 |
120 |
59 |
18 |
I2 (0.2)d |
TBHP (3) |
DMSO |
1.5 |
120 |
73 |
19 |
I2 (0.2)d |
TBHP (3) |
DMSO |
2.0 |
120 |
90 |
20 |
I2 (0.2)d |
TBHP (3) |
DMSO |
3.0 |
120 |
84 |
21 |
I2 (0.2)e |
TBHP (3) |
DMSO |
3.0 |
120 |
Trace |
22 |
I2 (0.2) |
DTBP (3) |
DMSO |
3.0 |
120 |
0 |
23 |
I2 (0.2) |
IBX (3) |
DMSO |
3.0 |
120 |
41 |
24 |
I2 (0.2) |
DMP (3) |
DMSO |
3.0 |
120 |
Trace |
25 |
I2 (0.2) |
DIB (3) |
DMSO |
3.0 |
120 |
Trace |
26 |
I2 (0.2) |
HTIB (3) |
DMSO |
3.0 |
120 |
Trace |
27 |
I2 (0.2) |
DDQ (3) |
DMSO |
3.0 |
120 |
Trace |
28 |
I2 (0.2) |
K2S2O8 (3) |
DMSO |
3.0 |
120 |
Trace |
29 |
I2 (0.2) |
O2 (1 atm) |
DMSO |
3.0 |
120 |
0 |
30 |
—f |
TBHP (3) |
DMSO |
3.0 |
120 |
— |
31 |
NIS (0.2) |
TBHP (3) |
DMSO |
3.0 |
120 |
26 |
32 |
KI (0.2) |
TBHP (3) |
DMSO |
3.0 |
120 |
19 |
33 |
CuI (0.2) |
TBHP (3) |
DMSO |
3.0 |
120 |
23 |
34 |
TBAI (0.2) |
TBHP (3) |
DMSO |
3.0 |
120 |
35 |
35 |
I2 (0.2) |
TBHP (3) |
DMF |
3.0 |
120 |
— |
36 |
I2 (0.2) |
TBHP (3) |
1,4-Dioxane |
3.0 |
120 |
— |
37 |
I2 (0.2) |
TBHP (3) |
THF |
3.0 |
120 |
— |
38 |
I2 (0.2) |
TBHP (3) |
Toluene |
3.0 |
120 |
— |
With the optimized reaction conditions in hand, we explored the scope and limitations of this transformation by using substituted ethylarenes. To our delight, the reaction was found to be robust and unaffected by the nature as well as the position of the substituent present in the aromatic ring. Ethylarenes bearing halogen substituents such as –F, –Cl and –Br at the ortho and para positions reacted smoothly with o-phenylenediamine to offer the corresponding quinoxalines 3ba, 3ca and 3da in yields ranging from 85–92%. The reaction of ethylarenes with electron donating substituents such as –Me, –OMe proceeded easily with o-phenylenediamine furnishing the corresponding quinoxalines 3ea, 3fa and 3ga in good yields (82–86%). The reaction of ethylarenes bearing an electron withdrawing substituent such as –NO2 with o-phenylenediamine resulted in formation of quinoxaline 3ha in excellent yield (91%).
Table 2 I2/TBHP catalyzed synthesis of quinoxalines from ethylarenes and o-phenylenediaminesab
Reaction conditions: 1 (1.0 mmol), I2 (0.2 mmol) and TBHP (3.0 mmol) was heated for 1.0 h at 120 °C in sealed tube, 2 (1.0 mmol) in DMSO (3.0 mL) was added and heating continued for 2.0 h. Isolated yield. |
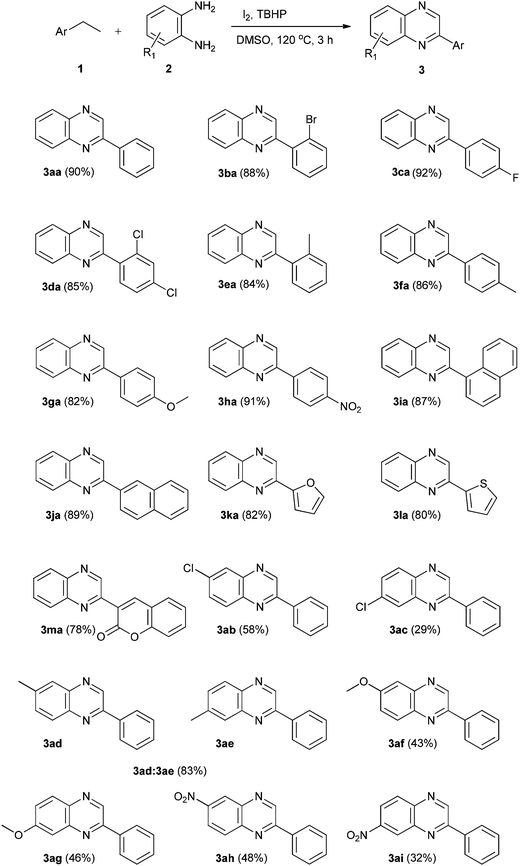 |
Similarly, 1-ethylnaphthalene and 2-ethylnaphthalene also offered the corresponding quinoxalines 3ia and 3ja in 87 and 89% respectively. Notably, heteroarylethanes such as 2-ethylfuran, 2-ethylthiophene and 3-ethyl-2H-benzopyran-2-one afforded the corresponding quinoxalines 3ka, 3la and 3ma in quantitative yields. To investigate the effect of substituent on reactivity and regioselectivity, different o-phenylenediamines such as 4-chloro, 4-methyl, 4-methoxy and 4-nitro-o-phenylenediamine were reacted with ethylbenzene (1a). The reaction of 4-chloro-o-phenylenediamine with ethylbenzene (1a) formed regioisomers 3ab and 3ac in 2
:
1 ratio with a combined yield of 87% while 4-methyl-o-phenylenediamine produced inseperable mixture of regioisomers 3ad and 3ae in 1
:
4 ratio which is confirmed by 1H NMR with 83% overall yield. 4-Methoxy-o-phenylenediamine reacted smoothly with ethylbenzene (1a) and offered regioisomers 3af and 3ag in 1
:
1 ratio with a total yield of 89%. 4-Nitro-o-phenylenediamine on reaction with ethylbenzene (1a) resulted in formation of regioisomers 3ah and 3ai in 3
:
2 ratio with 80% overall yield (Table 2).
Having obtained encouraging results with ethylarenes, we turned our attention to C–H functionalization of ethylenearenes followed by oxidative cyclization with o-phenylenediamines. When we reacted styrene (4a) with o-phenylenediamine (2a) under optimized conditions, C–H functionalization followed by oxidative cyclization occurred smoothly to offer quinoxaline 3aa in 92% yield. Ethylenearenes bearing halogen substituents such as –Cl and –Br at the meta and para positions also reacted smoothly with o-phenylenediamine to form the corresponding quinoxalines 3na and 3oa in excellent yields (90 and 93% respectively). The reaction of ethylenearenes with electron donating substituents –Me, –OMe proceeded to furnish the respective quinoxalines 3fa and 3pa in good yields (88 and 78% respectively). The reaction of ethylenearenes bearing an electron withdrawing substituent (–NO2) with o-phenylenediamine afforded quinoxaline 3ha in excellent yield (92%).
Likewise, 1-ethylenenaphthalene also produced the corresponding quinoxaline 3ia with 89% yield whereas, the reaction of heteroarylethylenes such as 2-vinylfuran and 3-vinyl-2H-benzopyran-2-one formed quinoxalines 3ka and 3ma in 84 and 80% yield respectively. Next, we studied the effect of substituted o-phenylenediamines on reactivity and regioselectivity. 4-Chloro-o-phenylenediamine reacted easily with styrene (4a) to form regioisomers 3ab and 3ac in 2
:
1 ratio with an overall yield of 91%. The reaction of 4-methoxy-o-phenylenediamine with styrene (4a) produced regioisomers 3af and 3ag in 1
:
1 ratio with a combined yield of 93%. 4-Nitro-o-phenylenediamine reacted smoothly with styrene (4a) to offer regioisomers 3ah and 3ai in 3
:
2 ratio with a total yield of 84% (Table 3).
Table 3 I2/TBHP catalyzed synthesis of quinoxalines from ethylenearenes and o-phenylenediaminesab
Reaction conditions: 4 (1.0 mmol), I2 (0.2 mmol) and TBHP (3.0 mmol) was heated for 1.0 h at 120 °C in sealed tube, 2 (1.0 mmol) in DMSO (3.0 mL) was added and heating continued for 2.0 h. Isolated yield. |
 |
With results of ethylarenes and ethylenearenes in hand, we conducted the reaction of phenylacetylene (5a) with o-phenylenediamine (2a) under optimized reaction conditions and obtained quinoxaline 3aa in excellent yield (89%). We also found that substituted (halogens, Me, OMe and NO2) ethynearenes reacted smoothly and afforded the corresponding quinoxalines in good to excellent yields (81–90%). 2-Ethynylnaphthalene gave quinoxaline 3ja in 85% yield. To study the reaction scope of o-phenylenediamines, we carried out the reaction of 4-chloro-o-phenylenediamine with phenylacetylene (5a). Notably, a mixture of regioisomers 3ab and 3ac in 2
:
1 ratio was obtained with an overall yield of 79%. The reaction of 4-methyl-o-phenylenediamine with phenylacetylene (5a) showed higher regioselectivity and formed regioisomers 3ad and 3ae in 1
:
4 ratio with a total yield of 85%. 4-Methoxy-o-phenylenediamine reacted with phenylacetylene (5a) to produce regioisomers 3af and 3ag in 1
:
1 ratio with a combined yield of 91%. 4-Nitro-o-phenylenediamine reacted smoothly with phenylacetylene (5a) to afford regioisomers 3ah and 3ai in 3
:
2 ratio with total yield of 81% (Table 4).
Table 4 I2/TBHP catalyzed synthesis of quinoxalines from ethynearenes and o-phenylenediaminesab
Reaction conditions: 5 (1.0 mmol), I2 (0.2 mmol) and TBHP (3.0 mmol) was heated for 1.0 h at 120 °C in sealed tube, 2 (1.0 mmol) in DMSO (3.0 mL) was added and heating continued for 2.0 h. Isolated yield. |
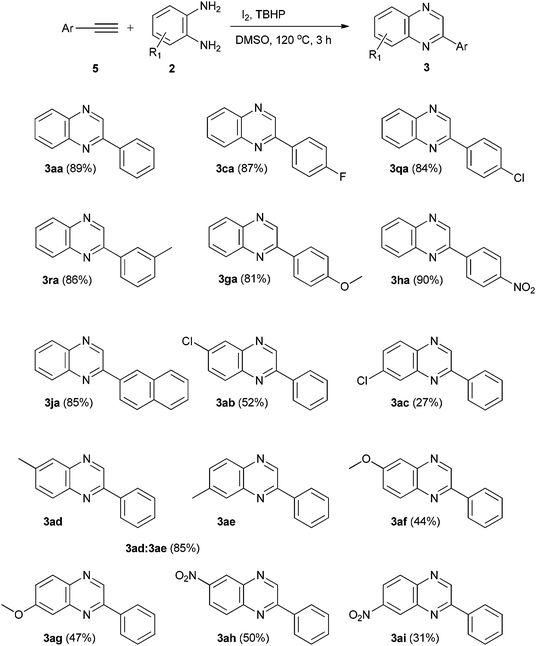 |
A few controlled experiments (Scheme 2) were performed in order to support our proposed mechanism. To demonstrate the involvement of TBHP in the formation of α-iodoacetophenone (B), a key intermediate, ethylbenzene (1a) or styrene (4a) or phenylacetylene (5a) were reacted with I2 and DMSO at 120 °C (Scheme 2a). These reactions failed to produce the α-iodoacetophenone (B), proving that TBHP plays a crucial role in the formation of α-iodoacetophenone (B) through radical intermediates. When ethylbenzene (1a) or styrene (4a) or phenylacetylene (5a) were treated with I2/TBHP in absence of DMSO (Scheme 2b), the reaction solely formed α-iodoacetophenone (B). Compound 1a on reaction with I2/TBHP (Scheme 2c) resulted in successive formation of acetophenone (A) and α-iodoacetophenone (B), an important intermediate, which is also obtained from compound 4a and 5a (Scheme 2d). α-Iodoacetophenone (B) was finally converted into phenylglyoxal (C) in presence of DMSO. It ascertained the requirement of DMSO as a solvent and a co-oxidant. Phenylglyoxal (C) reacted with o-phenylenediamine (2a) in presence of I2 and DMSO to form quinoxaline (3aa) exclusively (Scheme 2e). The reaction of compound 1a, 4a and 5a with o-phenylenediamine (2a) in presence of I2 and TBHP gave quinoxaline 3aa in low yield (Scheme 2f). Ethylbenzene (1a), styrene (4a) and phenylacetylene (5a) did not react with o-phenylenediamine (2a) in DMSO thereby clearly highlighting the role of I2/TBHP in C–H functionalization (Scheme 2g).
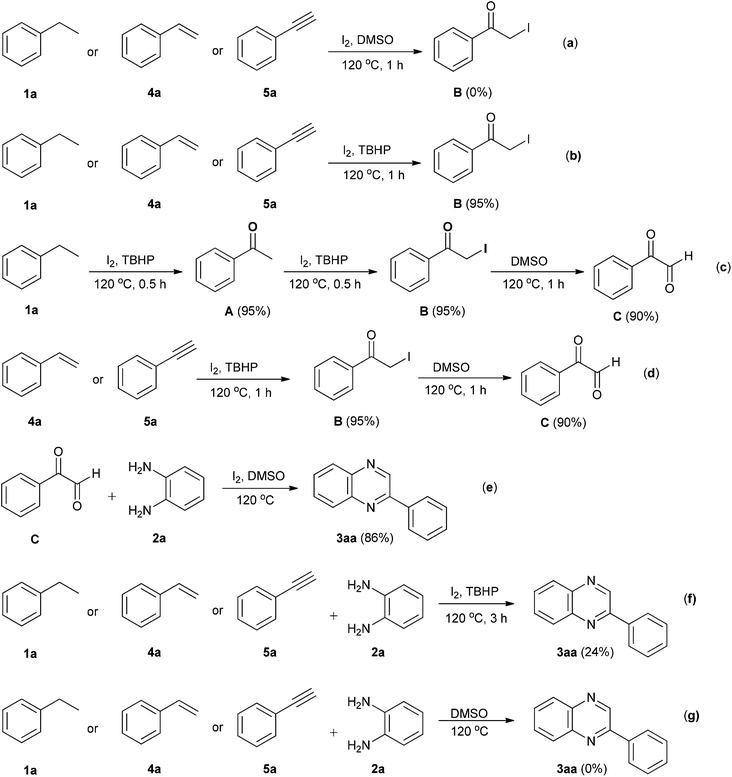 |
| Scheme 2 Control experiment. | |
A plausible mechanism for this transformation is presented in Scheme 3. Ethylbenzene (1a) is oxidized to acetophenone (A) by I2/TBHP via a radical mechanism with 1-phenylethanol as an intermediate product. Formation of acetophenone was confirmed by TLC, GC-MS and 1HNMR. Acetophenone (A) on iodination is converted into α-iodoacetophenone (B). This key intermediate is also produced from styrene (4a) and phenylacetylene (5a) by oxidation/iodination with I2/TBHP as depicted in Scheme 3. α-Iodoacetophenone (B) is further transformed via Kornblum oxidation into phenylglyoxal (C) which on reaction with o-phenylenediamine (2a) gives intermediate D. Cyclization of D followed by oxidation offer quinoxaline (3aa).
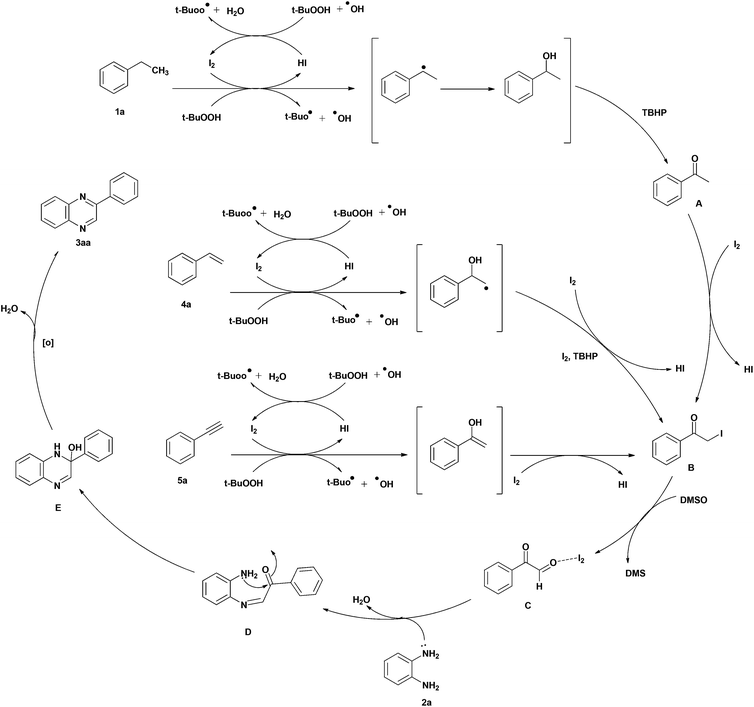 |
| Scheme 3 Plausible reaction mechanism. | |
Experimental section
General information
Chemical reagents were obtained from commercial companies. All reactions were performed in a sealed tube and monitored by TLC performed on aluminum plates (0.25 mm, E. Merck) precoated with silica gel Merck 60 F-254. Developed TLC plates were visualized under a short-wavelength UV lamp. Yields refer to spectroscopically (1H, 13C NMR) homogeneous material obtained after column chromatography performed on silica gel (100
:
200 mesh size) supplied by S. D. Fine Chemicals Limited, India. 1H and 13C NMR spectra were recorded in CDCl3 or DMSO-d6 solution with Brüker 400, Agilent 500 and 600 MHz spectrometers. Coupling constants (J) were measured in Hertz. Chemical shifts (δ) are quoted in ppm, relative to SiMe4 (δ = 0) as an internal standard. The number of protons (n) for a given resonance is indicated by nH. High resolution mass spectra (HRMS) were obtained by using positive electrospray ionization (ESI) by Time of Flight (TOF) method. Melting points were recorded on a standard melting point apparatus from Sunder Industrial Product, Mumbai and uncorrected.
General experimental procedure for synthesis of quinoxalines
A sealed tube equipped with magnetic stirring bar was charged with ethylarene (1) or ethylenearene (4) or ethynearene (5) (1.0 mmol), I2 (0.2 mmol) and tert-butyl hydroperoxide (TBHP, 3.0 mmol, 70% aq. solution) at room temperature. The resulting mixture was heated to 120 °C and maintained at 120 °C for 1.0 h. After disappearance of the reactant (monitored by TLC), substituted o-phenylenediamine 2 (1.0 mmol) in DMSO (3.0 mL) was added and heating continued at 120 °C for 2.0 h. After completion of the reaction, 20 mL of water was added to the reaction mixture and it was extracted with ethyl acetate (2 × 20 mL). The organic layer was washed with 10% Na2S2O3 solution (20 mL) and brine solution (20 mL) successively. The organic layer was dried over anhydrous Na2SO4 and concentrated under reduced pressure. The residue was purified by column chromatography on 100
:
200 mesh silica gel using n-hexane
:
ethyl acetate (8
:
2) as eluent to obtain the corresponding quinoxaline 3.
Product characterization data
2-Phenylquinoxaline (3aa). White solid; MP 76–78 °C; 1H NMR (600 MHz, CDCl3): δ = 7.53 (t, J = 7.8, 7.2 Hz, 1H), 7.58 (t, J = 7.8, 7.2 Hz, 2H), 7.76 (t, J = 7.8, 6.6 Hz, 1H), 7.80 (t, J = 7.2 Hz, 1H), 8.13 (d, J = 7.8 Hz, 1H), 8.17 (d, J = 8.4 Hz, 1H), 8.20 (d, J = 7.2 Hz, 2H), 9.34 (s, 1H); 13C NMR (150 MHz, CDCl3): δ = 127.53, 129.08, 129.14, 129.54, 129.59, 130.17, 130.29, 136.74, 141.52, 142.28, 143.33, 151.85; HRMS (ESI-MS): m/z [M + H]+ calcd for C14H11N2: 207.0923; found: 207.0925.
2-(2-Bromophenyl)quinoxaline (3ba). White solid; MP 116–118 °C; 1H NMR (400 MHz, CDCl3): δ = 7.38 (t, J = 7.2 Hz, 1H), 7.51 (t, J = 7.2 Hz, 1H), 7.68 (d, J = 7.2 Hz, 1H), 7.75 (d, J = 7.2 Hz, 1H), 7.81–7.84 (q, J = 3.2 Hz, 2H), 8.17 (d, J = 3.2 Hz, 2H), 9.19 (s, 1H); 13C NMR (100 MHz, CDCl3): δ = 122.05, 128.10, 129.32, 129.67, 130.24, 130.39, 130.99, 131.95, 133.51, 138.57, 141.41, 142.16, 146.21, 153.69; HRMS (ESI-MS): m/z [M + H]+ calcd for C14H10BrN2: 285.0028; found: 285.0030.
2-(4-Fluorophenyl)quinoxaline (3ca). White solid; MP 120–122 °C; 1H NMR (400 MHz, CDCl3): δ = 7.22–7.28 (m, 2H), 7.74–7.82 (q, J = 9.6, 8.0, 6.8 Hz, 2H), 8.13 (t, J = 8.0, 6.8 Hz, 2H), 8.20–8.23 (distorted q, J = 6.4, 5.6, 2.0 Hz, 2H), 9.30 (s, 1H); 13C NMR (100 MHz, CDCl3): δ = 116.15, 116.37, 129.18, 129.50, 129.58, 129.62, 130.43, 132.99, 133.02, 141.55, 142.25, 142.97, 150.81, 163.05, 165.54; HRMS (ESI-MS): m/z [M + H]+ calcd for C14H10FN2: 225.0829; found: 225.0833.
2-(2,4-Dichlorophenyl)quinoxaline (3da). White solid; MP 144–146 °C; 1H NMR (400 MHz, CDCl3): δ = 7.46–7.83 (m, 5H), 8.17 (s, 2H), 9.20 (s, 1H); 13C NMR (100 MHz, CDCl3): δ = 128.00, 129.35, 129.66, 130.16, 130.45, 130.52, 132.96, 133.40, 135.11, 136.37, 141.49, 142.33, 145.90, 151.35; HRMS (ESI-MS): m/z [M + H]+ calcd for C14H9Cl2N2: 275.0144; found: 275.0146.
2-(o-Tolyl)quinoxaline (3ea). White solid; MP 88–90 °C; 1H NMR (400 MHz, CDCl3): δ = 2.47 (s, 3H), 7.37 (s, 3H), 7.55 (d, J = 6.8 Hz, 1H), 7.80 (distorted t, J = 3.6, 3.2 Hz, 2H), 8.16 (d, J = 6.8 Hz, 2H), 9.02 (s, 1H); 13C NMR (100 MHz, CDCl3): δ = 20.43, 126.42, 129.22, 129.51, 129.63, 129.84, 130.08, 130.35, 131.30, 136.66, 137.20, 141.08, 142.11, 145.98, 155.09; HRMS (ESI-MS): m/z [M + H]+ calcd for C15H13N2: 221.1079; found: 221.1083.
2-(p-Tolyl)quinoxaline (3fa). White solid; MP 92–94 °C; 1H NMR (600 MHz, CDCl3): δ = 2.46 (s, 3H), 7.38 (d, J = 7.8 Hz, 2H), 7.74 (t, J = 7.8, 7.2 Hz, 1H), 7.78 (t, J = 7.8, 7.2 Hz, 1H), 8.10–8.12 (m, 3H), 8.15 (d, J = 8.4 Hz, 1H), 9.32 (s, 1H); 13C NMR (125 MHz, CDCl3): δ = 21.32, 127.37, 129.03, 129.48, 129.54, 129.77, 129.98, 133.96, 140.47, 141.42, 142.31, 143.35, 151.81; HRMS (ESI-MS): m/z [M + H]+ calcd for C15H13N2: 221.1079; found: 221.1081.
2-(4-Methoxyphenyl)quinoxaline (3ga). White solid; MP 98–100 °C; 1H NMR (400 MHz, CDCl3): δ = 3.91 (s, 3H), 7.09 (d, J = 8.8 Hz, 2H), 7.70–7.78 (m, 2H), 8.11 (t, J = 8.8 Hz, 2H), 8.18 (d, J = 8.8 Hz, 2H), 9.30 (s, 1H); 13C NMR (100 MHz, CDCl3): δ = 55.51, 114.66, 129.04, 129.13, 129.45, 130.25, 141.27, 142.38, 143.15, 151.51, 161.52; HRMS (ESI-MS): m/z [M + H]+ calcd for C15H13N2O: 237.1029; found: 237.1033.
2-(4-Nitrophenyl)quinoxaline (3ha). Yellow solid; MP 188–190 °C; 1H NMR (400 MHz, CDCl3): δ = 7.83 (t, J = 3.6, 3.2 Hz, 2H), 8.17 (t, J = 7.6 Hz, 2H), 8.40 (s, 4H), 9.38 (s, 1H); 13C NMR (100 MHz, CDCl3): δ = 124.38, 128.42, 129.35, 129.92, 130.78, 130.97, 142.17, 142.27, 142.62, 142.88, 148.87, 149.30; HRMS (ESI-MS): m/z [M + H]+ calcd for C14H10N3O2: 252.0774; found: 252.0778.
2-(Naphthalen-1-yl)quinoxaline (3ia). Off white solid; MP 134–136 °C; 1H NMR (400 MHz, CDCl3): δ = 7.51–7.58 (q, J = 6.8, 6.4 Hz, 2H), 7.64 (t, J = 7.6 Hz, 1H), 7.78 (d, J = 7.2 Hz, 1H), 7.81–7.86 (distorted q, J = 6.8, 4.0 Hz, 2H), 7.96 (d, J = 7.6 Hz, 1H), 8.01 (d, J = 8.0 Hz, 1H), 8.17 (d, J = 8.0 Hz, 1H), 8.20–8.24 (m, 2H), 9.17 (s, 1H); 13C NMR (100 MHz, CDCl3): δ = 125.08, 125.41, 126.35, 127.18, 128.54, 128.64, 129.31, 129.69, 129.91, 130.13, 130.39, 131.18, 134.05, 135.15, 141.40, 142.24, 146.63, 154.28; HRMS (ESI-MS): m/z [M + H]+ calcd for C18H13N2: 257.1079; found: 257.1081.
2-(Naphthalen-2-yl)quinoxaline (3ja). Yellow solid; MP 138–140 °C; 1H NMR (400 MHz, CDCl3): δ = 7.57 (s, 2H), 7.76–7.83 (distorted q, J = 8.8, 7.6 Hz, 2H), 7.92 (s, 1H), 8.03 (distorted d, J = 8.4 Hz, 2H), 8.14–8.22 (dd, J = 7.6 Hz, 2H), 8.37 (d, J = 7.6 Hz, 1H), 8.66 (s, 1H), 9.49 (s, 1H); 13C NMR (100 MHz, CDCl3): δ = 124.57, 126.79, 127.40, 127.59, 127.90, 128.99, 129.14, 129.24, 129.68, 129.71, 130.45, 134.22, 141.64, 143.61, 151.81; HRMS (ESI-MS): m/z [M + H]+ calcd for C18H13N2: 257.1079; found: 257.1083.
2-(Furan-2-yl)quinoxaline (3ka). Light brown solid; MP 98–100 °C; 1H NMR (400 MHz, CDCl3): δ = 6.63 (s, 1H), 7.32 (d, J = 2.8 Hz, 1H), 7.68–7.77 (m, 3H), 8.05–8.10 (q, J = 8.4, 4.4 Hz, 2H), 9.24 (s, 1H); 13C NMR (100 MHz, CDCl3): δ = 111.91, 112.58, 129.28, 129.42, 130.57, 141.35, 142.11, 142.17, 143.92, 145.19, 151.64; HRMS (ESI-MS): m/z [M + H]+ calcd for C12H9N2O: 197.0716; found: 197.0720.
2-(Thiophen-2-yl)quinoxaline (3la). Pale yellow solid; MP 120–122 °C; 1H NMR (400 MHz, CDCl3): δ = 7.20 (t, J = 4.4 Hz, 1H), 7.55 (d, J = 4.8 Hz, 1H), 7.69–7.76 (q, J = 7.6, 6.8 Hz, 2H), 7.86 (d, J = 3.2 Hz, 1H), 8.05–8.08 (distorted q, J = 3.6, 2.4 Hz, 2H), 9.24 (s, 1H); 13C NMR (100 MHz, CDCl3): δ = 127.05, 128.55, 129.19, 129.28, 129.91, 130.51, 141.40, 142.12, 142.20, 142.30, 147.45; HRMS (ESI-MS): m/z [M + H]+ calcd for C12H9N2S: 213.0487; found: 213.0491.
3-(Quinoxalin-2-yl)-2H-chromen-2-one (3ma). Yellow solid; MP 198–200 °C; 1H NMR (400 MHz, DMSO-d6): δ = 7.47 (t, J = 7.6, 7.2 Hz, 1H), 7.55 (d, J = 8.0 Hz, 1H), 7.75 (t, J = 7.6, 7.2 Hz, 1H), 7.90–7.96 (m, 2H), 8.03 (d, J = 7.6 Hz, 1H), 8.16–8.21 (distorted q, J = 7.2, 2.4 Hz, 2H), 8.94 (s, 1H), 9.60 (s, 1H); 13C NMR (100 MHz, DMSO-d6): δ = 116.14, 119.10, 123.62, 125.00, 128.86, 129.07, 129.76, 130.65, 130.77, 133.26, 141.24, 141.42, 144.59, 145.38, 147.84, 153.83, 159.61; HRMS (ESI-MS): m/z [M + H]+ calcd for C17H11N2O2: 275.0821; found: 275.0823.
6-Chloro-2-phenylquinoxaline (3ab). White solid; MP 146–148 °C; 1H NMR (400 MHz, CDCl3): δ = 7.52–7.59 (m, 3H), 7.71–7.74 (dd, J = 2.4, 2.0 Hz, 1H), 8.09 (d, J = 9.2 Hz, 1H), 8.11 (d, J = 2.0 Hz, 1H), 8.19 (d, J = 6.8 Hz, 2H), 9.33 (s, 1H); 13C NMR (100 MHz, CDCl3): δ = 127.54, 128.11, 129.24, 130.45, 130.86, 131.34, 135.28, 136.41, 140.88, 141.86, 144.17, 151.98; HRMS (ESI-MS): m/z [M + H]+ calcd for C14H10ClN2: 241.0533; found: 241.0535.
7-Chloro-2-phenylquinoxaline (3ac). White solid; MP 126–128 °C; 1H NMR (400 MHz, CDCl3): δ = 7.52–7.60 (m, 3H), 7.67–7.69 (dd, J = 2.0 Hz, 1H), 8.05 (d, J = 8.8 Hz, 1H), 8.15 (d, J = 2.4 Hz, 1H), 8.18–8.20 (dd, J = 1.2 Hz, 2H), 9.31 (s, 1H); 13C NMR (100 MHz, CDCl3): δ = 127.62, 128.51, 129.22, 130.37, 130.51, 130.56, 136.11, 136.33, 140.12, 142.67, 143.43, 152.56; HRMS (ESI-MS): m/z [M + H]+ calcd for C14H10ClN2: 241.0533; found: 241.0537.
6-Methyl-2-phenylquinoxaline (3ad) and 7-methyl-2-phenylquinoxaline (3ae) 3ad
:
3ae = 1
:
4. Pale yellow solid; 1H NMR (500 MHz, CDCl3): δ = 2.61 (s, 3H), 7.46–7.62 (m, 4H), 7.89 and 7.94 (s, 1H), 8.01 and 8.05 (d, J = 8.5 Hz, 1H), 8.18 (d, J = 7.0 Hz, 2H), 9.26 and 9.28 (s, 1H); 13C NMR (125 MHz, CDCl3): δ = 151.78, 151.08, 143.28, 143.13, 142.49, 142.37, 141.58, 140.76, 140.13, 136.93, 132.71, 132.50, 130.06, 129.80, 129.21, 129.16, 128.61, 128.50, 127.99, 127.42, 127.39; HRMS (ESI-MS): m/z [M + H]+ calcd for C15H13N2: 221.1079; found: 221.1083.
6-Methoxy-2-phenylquinoxaline (3af). Pale yellow solid; MP 94–96 °C; 1H NMR (500 MHz, CDCl3): δ = 3.99 (s, 3H), 7.38–7.40 (dd, J = 9.0, 2.5 Hz, 1H), 7.44 (d, J = 2.0 Hz, 1H), 7.51 (t, J = 7.5, 7.0 Hz, 1H), 7.56 (t, J = 8.0, 7.0 Hz, 2H), 7.99 (d, J = 9.0 Hz, 1H), 8.16 (d, J = 7.5 Hz, 2H), 9.16 (s, 1H); 13C NMR (125 MHz, CDCl3): δ = 55.90, 106.99, 122.99, 127.51, 128.97, 129.21, 130.07, 136.99, 137.76, 140.82, 143.95, 151.93, 161.05; HRMS (ESI-MS): m/z [M + H]+ calcd for C15H13N2O: 237.1029; found: 237.1031.
7-Methoxy-2-phenylquinoxaline (3ag). Pale yellow solid; MP 92–94 °C; 1H NMR (500 MHz, CDCl3): δ = 3.99 (s, 3H), 7.40 (d, J = 2.0 Hz, 1H), 7.42–7.45 (dd, J = 9.5, 2.5 Hz, 1H), 7.49 (t, J = 7.5, 7.0 Hz, 1H), 7.55 (t, J = 7.5 Hz, 2H), 8.03 (d, J = 9.5 Hz, 1H), 8.15 (d, J = 7.5 Hz, 2H), 9.24 (s, 1H); 13C NMR (125 MHz, CDCl3): δ = 55.88, 106.41, 123.41, 127.20, 129.19, 129.80, 130.50, 136.99, 138.40, 143.10, 143.26, 149.62, 160.54; HRMS (ESI-MS): m/z [M + H]+ calcd for C15H13N2O: 237.1029; found: 237.1033.
6-Nitro-2-phenylquinoxaline (3ah). Yellow solid; MP 210–212 °C; 1H NMR (500 MHz, CDCl3): δ = 7.60–7.63 (m, 3H), 8.25–8.29 (m, 3H), 8.54–8.56 (dd, J = 9.0, 2.5 Hz, 1H), 9.02 (d, J = 2.5 Hz, 1H), 9.49 (s, 1H); 13C NMR (125 MHz, CDCl3): δ = 123.82, 125.72, 127.92, 129.27, 129.51, 131.14, 135.60, 140.31, 144.89, 145.40, 147.43, 154.29; HRMS (ESI-MS): m/z [M + H]+ calcd for C14H10N3O2: 252.0774; found: 252.0776.
7-Nitro-2-phenylquinoxaline (3ai). Yellow solid; MP 208–210 °C; 1H NMR (500 MHz, CDCl3): δ = 7.60–7.63 (m, 3H), 8.24–8.29 (m, 3H), 8.49–8.51 (dd, J = 9.5, 2.5 Hz, 1H), 9.05 (d, J = 2.0 Hz, 1H), 9.47 (s, 1H); 13C NMR (125 MHz, CDCl3): δ = 122.83, 125.81, 127.71, 129.26, 129.49, 130.82, 135.52, 141.32, 143.88, 146.05, 148.26, 153.69; HRMS (ESI-MS): m/z [M + H]+ calcd for C14H10N3O2: 252.0774; found: 252.0778.
2-(3-Chlorophenyl)quinoxaline (3na). Yellow solid; MP 130–132 °C; 1H NMR (400 MHz, CDCl3): δ = 7.49 (d, J = 4.4 Hz, 2H), 7.75–7.82 (q, J = 7.6, 6.8 Hz, 2H), 8.06 (s, 1H), 8.14 (t, J = 8.8 Hz, 2H), 8.22 (s, 1H), 9.29 (s, 1H); 13C NMR (100 MHz, CDCl3): δ = 125.59, 127.74, 129.24, 129.74, 130.06, 130.27, 130.44, 130.62, 135.42, 138.58, 141.87, 142.26, 143.01, 150.40; HRMS (ESI-MS): m/z [M + H]+ calcd for C14H10ClN2: 241.0533; found: 241.0535.
2-(4-Bromophenyl)quinoxaline (3oa). Yellow solid; MP 128–130 °C; 1H NMR (400 MHz, CDCl3): δ = 7.69–7.82 (m, 4H), 8.08–8.16 (m, 4H), 9.31 (s, 1H); 13C NMR (100 MHz, CDCl3): δ = 125.01, 129.03, 129.20, 129.64, 129.83, 130.50, 132.38, 135.68, 141.73, 142.27, 142.83, 150.69; HRMS (ESI-MS): m/z [M + H]+ calcd for C14H10BrN2: 285.0028; found: 285.0032.
2-(2,6-Dimethoxyphenyl)quinoxaline (3pa). Yellow solid; MP 68–70 °C; 1H NMR (400 MHz, CDCl3): δ = 3.86 (s, 3H), 3.87 (s, 3H), 7.01 (s, 2H), 7.48 (s, 1H), 7.75 (t, J = 3.6 Hz, 2H), 8.11–8.16 (q, J = 6.8, 6.4 Hz, 2H), 9.35 (s, 1H); 13C NMR (100 MHz, CDCl3): δ = 55.98, 56.37, 113.09, 116.11, 117.30, 127.08, 129.09, 129.52, 129.57, 129.86, 141.11, 142.67, 147.28, 151.79, 152.01, 154.30; HRMS (ESI-MS): m/z [M + H]+ calcd for C16H15N2O2: 267.1134; found: 267.1136.
2-(4-Chlorophenyl)quinoxaline (3qa). White solid; MP 136–138 °C; 1H NMR (400 MHz, CDCl3): δ = 7.53–7.59 (distorted q, J = 8.0, 7.2 Hz, 2H), 7.75–7.82 (q, J = 7.6, 6.4 Hz, 2H), 8.12–8.17 (m, 4H), 9.31 (s, 1H); 13C NMR (100 MHz, CDCl3): δ = 128.80, 129.18, 129.42, 129.63, 129.81, 130.50, 135.23, 136.62, 140.64, 142.89, 143.98, 150.64; HRMS (ESI-MS): m/z [M + H]+ calcd for C14H10ClN2: 241.0533; found: 241.0537.
2-(m-Tolyl)quinoxaline (3ra). White solid; MP 84–86 °C; 1H NMR (400 MHz, CDCl3): δ = 2.50 (s, 3H), 7.33–7.46 (distorted dd, J = 7.2, 6.0 Hz, 2H), 7.76 (t, J = 8.4, 8.0 Hz, 2H), 7.96 (d, J = 6.8 Hz, 1H), 8.02 (s, 1H), 8.11–8.17 (q, J = 8.4, 7.6 Hz, 2H), 9.31 (s, 1H); 13C NMR (100 MHz, CDCl3): δ = 21.65, 124.76, 128.28, 129.12, 129.19, 129.54, 129.65, 130.33, 131.08, 136.81, 139.03, 141.62, 142.36, 143.61, 152.14; HRMS (ESI-MS): m/z [M + H]+ calcd for C15H13N2: 221.1079; found: 221.1081.
Conclusion
In conclusion, we have developed a direct and efficient method to prepare quinoxaline derivatives from easily available multiform substrates. Use of simple unactivated ethylarenes, ethylenearenes and ethynearenes, tolerance to broad range of functional groups and use of inexpensive catalyst are some striking features of this protocol. We anticipate that this operationally simple tandem process will be widely adopted for construction of quinoxaline moieties needed for the synthesis of complex molecules.
Acknowledgements
This work was supported by CSIR-New Delhi (sanction no. 01(2427)/10/EMR-II dated 28/12/2010, pool scheme no. 8644-A). K.S.V. and H.P.K. thank UGC-SAP and CSIR-New Delhi respectively for award of Senior Research Fellowships. The authors thank Prof. Prakash M. Bhate for his generous support.
Notes and references
- N. D. Sonawane and D. W. Rangnekar, J. Heterocycl. Chem., 2002, 39, 303–308 CrossRef CAS.
- T. Hirayama, S. Yamasaki, H. Ameku, T. Ishii, T. Thiemann and S. Mataka, Dyes Pigm., 2005, 67, 105–110 CrossRef CAS PubMed.
- S. Dailey, W. J. Feast, R. J. Peace, I. C. Sage, S. Till and E. L. Wood, J. Mater. Chem., 2001, 11, 2238–2243 RSC.
- K. R. Justin Thomas, M. Velusamy, J. T. Lin, C.-H. Chuen and Y.-T. Tao, Chem. Mater., 2005, 17, 1860–1866 CrossRef.
- J. L. Sessler, H. Maeda, T. Mizuno, V. M. Lynch and H. Furuta, Chem. Commun., 2002, 862–863 RSC.
-
(a) W. He, M. R. Myers, B. Hanney, A. P. Spada, G. Bilder, H. Galzcinski, D. Amin, S. Needle, K. Page, Z. Jayyosi and M. H. Perrone, Bioorg. Med. Chem. Lett., 2003, 13, 3097–3100 CrossRef CAS;
(b) Y. B. Kim, Y. H. Kim, J. Y. Park and S. K. Kim, Bioorg. Med. Chem. Lett., 2004, 14, 541–544 CrossRef CAS PubMed.
- G. Sakata, K. Makino and Y. Kurasawa, Heterocycles, 1988, 27, 2481–2515 CrossRef CAS PubMed , and references cited therein.
-
(a) A. Dell, D. H. Williams, H. R. Morris, G. A. Smith, J. Feeney and G. C. K. Roberts, J. Am. Chem. Soc., 1975, 97, 2497–2502 CrossRef CAS;
(b) C. Bailly, S. Echepare, F. Gago and M. Waring, Anti-Cancer Drug Des., 1999, 14, 291–303 CAS;
(c) K. Sato, O. Shiratori and K. Katagiri, J. Antibiot., 1967, 20, 270–276 CAS.
-
(a) A. Katoh, T. Yoshida and J. Ohkanda, Heterocycles, 2000, 52, 911–920 CrossRef CAS;
(b) J. L. Sessler, H. Maeda, T. Mizuno, V. M. Lynch and H. Furuta, J. Am. Chem. Soc., 2002, 124, 13474–13479 CrossRef CAS PubMed;
(c) M. J. Crossley and L. A. Johnston, Chem. Commun., 2002, 1122–1123 RSC;
(d) T. Yamaguchi, S. Matsumoto and K. Watanabe, Tetrahedron Lett., 1998, 39, 8311–8312 CrossRef CAS.
-
(a) Z. Zhao, D. D. Wisnoski, S. E. Wolkenberg, W. H. Leister, Y. Wang and C. W. Lindsley, Tetrahedron Lett., 2004, 45, 4873–4876 CrossRef CAS PubMed;
(b) S. Ajaikumar and A. Pandurangan, Appl. Catal., A, 2009, 357, 184–192 CrossRef CAS PubMed;
(c) M. M. Heravi, K. Bakhtiari, H. A. Oskooie and S. Taheri, Heteroat. Chem., 2008, 19, 218–220 CrossRef CAS;
(d) R. M. Adlington, J. E. Baldwin, D. Catterick and G. J. Pritchard, J. Chem. Soc., Perkin Trans. 1, 2001, 1, 668–679 RSC;
(e) B. C. Raju, N. D. Theja and J. A. Kumar, Synth. Commun., 2008, 39, 175–188 CrossRef;
(f) J.-T. Hou, Y.-H. Liu and Z.-H. Zhang, J. Heterocycl. Chem., 2010, 47, 703–710 CAS;
(g) S. Sadjadi, S. Sadjadi and R. Hekmatshoar, Ultrason. Sonochem., 2010, 17, 764–767 CrossRef CAS PubMed;
(h) M. L. H. Mantel, A. T. Lindhardt, D. Lupp and T. Skrydstrup, Chem.–Eur. J., 2010, 16, 5437–5442 CrossRef CAS PubMed;
(i) M. Lian, Q. Li, Y. Zhu, G. Yin and A. Wu, Tetrahedron, 2012, 68, 9598–9605 CrossRef CAS PubMed.
-
(a) S. Y. Kim, K. H. Park and Y. K. Chung, Chem. Commun., 2005, 1321–1323 RSC;
(b) V. Jeena and R. S. Robinson, Beilstein J. Org. Chem., 2009, 5, 24 Search PubMed;
(c) A. Shaabani and A. Maleki, Chem. Pharm. Bull., 2008, 56, 79–81 CrossRef CAS.
- A. Kumar, S. kumar, A. Saxena, A. De and S. Mozumdar, Catal. Commun., 2008, 9, 778–784 CrossRef CAS PubMed.
-
(a) L. Nagarapu, R. Mallepalli, G. Arava and L. Yeramanchi, Chem.–Eur. J., 2010, 1, 228–231 CrossRef CAS;
(b) A. Kumar, A. Verma, G. Chawla and Vaishali, Int. J. ChemTech Res., 2009, 1, 1177–1181 CAS.
-
(a) K. C. Nicolaou, T. Montagnon, T. Ulven, P. S. Baran, Y.-L. Zhong and F. Sarabia, J. Am. Chem. Soc., 2002, 124, 5718–5728 CrossRef CAS PubMed;
(b) P.-Y. Lin, R.-S. Hou, H.-M. Wang, I.-J. Kang and L.-C. Chen, J. Chin. Chem. Soc., 2009, 56, 683–687 CAS.
- M. M. Ali, M. M. F. Ismail, M. S. A. El-Gaby, M. A. Zahran and Y. A. Ammar, Molecules, 2000, 5, 864–873 CrossRef CAS PubMed.
- H. Thakuria and G. Das, J. Chem. Sci., 2006, 118, 425–428 CrossRef CAS.
- O. A. Attanasi, L. De Crescentini, P. Filippone, F. Mantellini and S. Santeusanio, Helv. Chim. Acta, 2001, 84, 2379–2386 CrossRef CAS.
- J. Barluenga, F. Aznar, R. Liz and M.-P. Cabal, Synthesis, 1985, 1985, 313–314 CrossRef PubMed.
-
(a) S. Chandrasekhar, N. K. Reddy and V. P. Kumar, Tetrahedron Lett., 2010, 51, 3623–3625 CrossRef CAS PubMed;
(b) W. Wang, Y. Shen, X. Meng, M. Zhao, Y. Chen and B. Chen, Org. Lett., 2011, 13, 4514–4517 CrossRef CAS PubMed.
- C. S. Cho and S. G. Oh, Tetrahedron Lett., 2006, 47, 5633–5636 CrossRef CAS PubMed.
- L. J. Martin, A. L. Marzinzik, S. V Ley and I. R. Baxendale, Org. Lett., 2011, 13, 320–323 CrossRef CAS PubMed.
- S. Sithambaram, Y. Ding, W. Li, X. Shen, F. Gaenzler and S. L. Suib, Green Chem., 2008, 10, 1029–1032 RSC.
- B. Das, K. Venkateswarlu, K. Suneel and A. Majhi, Tetrahedron Lett., 2007, 48, 5371–5374 CrossRef CAS PubMed.
- B. Madhav, S. Narayana Murthy, V. Prakash Reddy, K. Rama Rao and Y. V. D. Nageswar, Tetrahedron Lett., 2009, 50, 6025–6028 CrossRef CAS PubMed.
- J.-P. Wan, S.-F. Gan, J.-M. Wu and Y. Pan, Green Chem., 2009, 11, 1633–1637 RSC.
- H. M. Meshram, G. Santosh Kumar, P. Ramesh and B. Chennakesava Reddy, Tetrahedron Lett., 2010, 51, 2580–2585 CrossRef CAS PubMed.
-
(a) S. A. Raw, C. D. Wilfred and R. J. K. Taylor, Chem. Commun., 2003, 2286–2287 RSC;
(b) S. A. Raw, C. D. Wilfred and R. J. K. Taylor, Org. Biomol. Chem., 2004, 2, 788–796 RSC;
(c) R. S. Robinson and R. J. Taylor, Synlett, 2005, 1003–1005 CAS;
(d) C. S. Cho and S. G. Oh, J. Mol. Catal. A: Chem., 2007, 276, 205–210 CrossRef CAS PubMed.
- F. Pan, T.-M. Chen, J.-J. Cao, J.-P. Zou and W. Zhang, Tetrahedron Lett., 2012, 53, 2508–2510 CrossRef CAS PubMed.
- K. T. Venkateswara Rao, P. S. Sai Prasad and N. Lingaiah, J. Mol. Catal. A: Chem., 2009, 312, 65–69 CrossRef CAS PubMed.
- S. Paul and B. Basu, Tetrahedron Lett., 2011, 52, 6597–6602 CrossRef CAS PubMed.
- K. Padmavathy, G. Nagendrappa and K. V. Geetha, Tetrahedron Lett., 2011, 52, 544–547 CrossRef CAS PubMed.
-
(a) B. Pawar, V. Padalkar, K. Phatangare, S. Nirmalkar and A. Chaskar, Catal. Sci. Technol., 2011, 1, 1641–1644 RSC;
(b) S. Takale, S. Parab, K. Phatangare, R. Pisal and A. Chaskar, Catal. Sci. Technol., 2011, 1, 1128–1132 RSC;
(c) A. Chaskar, V. Padalkar, K. Phatangare, B. Langi and C. Shah, Synth. Commun., 2010, 40, 2336–2340 CrossRef CAS;
(d) K. Phatangare, V. Padalkar, D. Mhatre, K. Patil and A. Chaskar, Synth. Commun., 2009, 39, 4117–4121 CrossRef CAS;
(e) A. Chaskar, V. Padalkar, K. Phatangare, K. Patil, A. Bodkhe and B. Langi, Appl. Catal., A, 2009, 359, 84–87 CrossRef CAS PubMed;
(f) A. C. Chaskar, S. R. Bhandari, A. B. Patil, O. P. Sharma and S. Mayeker, Synth. Commun., 2008, 39, 366–370 CrossRef;
(g) K. S. Vadagaonkar, K. Murugan, A. C. Chaskar and P. M. Bhate, RSC Adv., 2014, 4, 34056–34064 RSC;
(h) H. P. Kalmode, K. S. Vadagaonkar and A. C. Chaskar, RSC Adv., 2014, 4, 60316–60326 RSC.
Footnotes |
† Electronic supplementary information (ESI) available. See DOI: 10.1039/c4ra08589b |
‡ The authors contributed equally to this work. |
|
This journal is © The Royal Society of Chemistry 2015 |
Click here to see how this site uses Cookies. View our privacy policy here.