Solar ultraviolet radiation and ozone depletion-driven climate change: effects on terrestrial ecosystems
Received
20th October 2014
, Accepted 20th October 2014
First published on 1st December 2014
Abstract
In this assessment we summarise advances in our knowledge of how UV-B radiation (280–315 nm), together with other climate change factors, influence terrestrial organisms and ecosystems. We identify key uncertainties and knowledge gaps that limit our ability to fully evaluate the interactive effects of ozone depletion and climate change on these systems. We also evaluate the biological consequences of the way in which stratospheric ozone depletion has contributed to climate change in the Southern Hemisphere. Since the last assessment, several new findings or insights have emerged or been strengthened. These include: (1) the increasing recognition that UV-B radiation has specific regulatory roles in plant growth and development that in turn can have beneficial consequences for plant productivity via effects on plant hardiness, enhanced plant resistance to herbivores and pathogens, and improved quality of agricultural products with subsequent implications for food security; (2) UV-B radiation together with UV-A (315–400 nm) and visible (400–700 nm) radiation are significant drivers of decomposition of plant litter in globally important arid and semi-arid ecosystems, such as grasslands and deserts. This occurs through the process of photodegradation, which has implications for nutrient cycling and carbon storage, although considerable uncertainty exists in quantifying its regional and global biogeochemical significance; (3) UV radiation can contribute to climate change via its stimulation of volatile organic compounds from plants, plant litter and soils, although the magnitude, rates and spatial patterns of these emissions remain highly uncertain at present. UV-induced release of carbon from plant litter and soils may also contribute to global warming; and (4) depletion of ozone in the Southern Hemisphere modifies climate directly via effects on seasonal weather patterns (precipitation and wind) and these in turn have been linked to changes in the growth of plants across the Southern Hemisphere. Such research has broadened our understanding of the linkages that exist between the effects of ozone depletion, UV-B radiation and climate change on terrestrial ecosystems.
Introduction
We have focused mainly on recent work in order to highlight the progress made to date, and to attempt an analysis of the complexity of both independent and interacting factors on terrestrial ecosystems in terms of UV radiation and other environmental constraints, including emerging evidence of the role of stratospheric ozone trends in affecting climate.
Ozone depletion, changed exposure to ultraviolet-B (UV-B, 280–315 nm) radiation, and climate change exert both individual and interactive effects on biological systems, with intricate feedbacks.1,2 Some of the key factors interacting with UV radiation that affect organism response are water availability, temperature, and nutrient availability. UV radiation has also been implicated as a contributor to global warming through its stimulation of volatile organic compounds from plants, plant litter and soils. Emission of carbon dioxide (CO2) from plant litter and soils may also contribute to global warming.3,4
Ozone depletion modifies Southern Hemisphere summer weather through its effect on the Southern Annular Mode (SAM), with consequences for plant growth in South America, New Zealand, and Antarctica already reported.5–7 These impacts of ozone depletion on other climate factors (e.g. wind patterns, precipitation, and warming) may result in an increase in the interactive effects of UV radiation with drought and temperature. Other seasonal weather phenomena need to be taken into account to gain an accurate perspective of the different determinants of UV exposure on terrestrial organisms. These include La Niña and El Niño events, which change cloud cover, winds, sea surface temperatures, and atmospheric pressure at sea level. In addition, changes in land-use and vegetation cover, which also feed back to climate systems, have implications on the exposure, and thus response, of organisms to UV radiation.
During the course of research on the effects of UV radiation, much emphasis has been placed on the potential detrimental impacts on plants and ecosystems. However, the balance of recent evidence is shifting to show that while some detrimental effects do occur, UV radiation is also a key regulator of plant morphology and physiological, biochemical and genetic processes, and is important in animal and plant signalling. Following on from this line of investigation, it has also become apparent that UV radiation and climate variables can be usefully exploited for value-adding to, e.g., agricultural crops.8 The emerging concept, that agricultural2,9 plants can become more hardy through exposure to UV radiation, represents a marked shift in perspective.8,9 In addition, certain plants produce more medicinal compounds with exposure to UV radiation.10 The overall objective is to boost the quality and/or quantity of the yield, usually selectively, e.g. by making plants less prone to attack by pests and diseases. Concepts such as that of “eustress” are also relevant. Eustress is analogous to “priming” where a stress is imposed on plants to acclimate them and develop tolerance, which facilitates better growth when exposed to a more severe stress.9,11
Exposure to ecologically-relevant levels of UV radiation is generally not deleterious as long as plants are able to acclimatise, although this depends on the environmental conditions, including climate variables, latitudinal location12 and plant type (e.g. whether plants are herbaceous or woody). Consequently, the direct negative effects of exposure to UV-B radiation on plant growth, photosynthesis, and productivity are generally minor, or not detectable (summarised in meta-analyses by Searles et al.13 and Newsham and Robinson14). However, indirect effects of exposure to UV radiation are often more pronounced than direct effects and need to be addressed to obtain a holistic perspective of the role of UV radiation as a regulator and modifier of ecosystem and organism response.
In this current assessment, we focus on the way in which UV radiation, stratospheric ozone trends, climate and other phenomena affect the biosphere, in order to better understand the current interactive effects from different stresses and to identify possible new interactions and their implications. This will allow for an evaluation of the capability of terrestrial ecosystems to adapt to a changing environment in which UV radiation plays an integral part in the response. Additionally, an assessment of these interactive processes on organisms and ecosystems recognises that the effects of UV radiation often represent a balance of both positive and negative influences.2 Although the role of UV-B radiation is a major consideration in this paper, other relevant and often interacting factors, such as stratospheric ozone trends and climate change cannot be meaningfully separated.
Multiple plant stresses and their implications in the response to UV radiation
Evaluation of the effect of different levels of exposure to UV-B radiation, whether beneficial or not, is complicated by the dependency on many other variables, including the sensitivity of diverse ecosystems and species. This differential sensitivity, which results in distinctive response patterns, reflects the complexity of the biosphere as our understanding increases.
UV radiation, temperature and drought
The volume of research concentrating on the impacts of UV radiation and drought is indicative of the increasing awareness of a changing climate coupled with other interlinking factors. However, there are still several knowledge gaps, e.g. at the mechanistic level around drought and UV-B signalling interactions and the consequences at the molecular level leading to plant response. Areas such as the Middle East, North Africa, certain regions of Australia and the Mediterranean are particularly vulnerable to climate change.15 Research on plants originating from drought-prone regions (e.g. the Mediterranean area), provides insight into current and potential long-term constraints for growth of agricultural plants and ecosystems by increased temperature, scarcity of water and enhanced exposure to UV radiation.16 Predicted decreased cloudiness in the Mediterranean area is likely to enhance exposure to UV-B and UV-A radiation (315–400 nm).17 Other co-occurring factors modifying UV radiation at the Earth's surface include air pollution and aerosol load.18
High solar radiation, high temperatures, and drought conditions can lead to an array of responses including oxidative stress and physiological and metabolic acclimation.19 The frequently observed, although not universal, cross-tolerance to drought stress and UV radiation is in contrast to the increased sensitivity to UV radiation that can occur in adequately watered plants (Caldwell et al.20 and references therein and others21–23). For example, in many woody Mediterranean plants, several physiological and biochemical traits (e.g. plant growth, net rate of assimilation of CO2, and photochemistry) show little response to changes in UV radiation against a background of high temperatures and drought periods.23
UV radiation and other stress factors can increase allocation of newly assimilated carbon to polyphenols, and in particular, flavonoid compounds, indicative of an energy shift in order to acclimate to stress conditions (Ballaré et al.1 and references therein and Guidi et al.19). The lack of a substantial response to UV radiation in many of the parameters measured in Mediterranean plants may also be explained by biochemical acclimation induced by the visible part of the solar spectrum, thus leading to acclimation to UV, and ultimately adaptation.19 This involves effective scavenging of reactive oxygen species and other protective mechanisms such as morphological, physiological, and biochemical changes16 that contribute to drought and high temperature tolerance, as well as positive plant–insect interactions.24 Decreased plant productivity and changes in crop quality may, however, occur with long-term and multiple stress exposure.
Significance of sequential stress
In the context of interactive stresses, recent work has continued to address the importance of the sequence in which plants are exposed to the different stresses (reviewed in Bandurska et al.25), although further supporting research is needed in this area. Field studies are also lacking, which may be a reflection of the difficulties encountered in trying to evaluate sequential stresses under more natural conditions. A growth chamber study by Bandurska and Cieslak26 showed that exposure of plants to UV-B radiation and drought, whether in combination or individually applied, affected metabolic processes differently at different locations within the plant. With separate applications of enhanced UV-B radiation and simulated drought conditions, plant growth is often retarded and oxidative stress increases. In general, exposure to both UV-B radiation and drought can elicit a number of physiological and biochemical responses that are common to both abiotic stresses. These involve increases in oxidative stress through production of reactive oxygen species, including hydrogen peroxide and nitric oxide, growth inhibition and, in some cases, the induction of phenolic compounds (Bandurska et al.26 and references therein). UV-induced oxidative damage, such as lipid peroxidation, can be reduced by pre-exposing plants to mild drought conditions.
Sequential or simultaneous exposure to stress factors can also be genotype-specific. For example, as illustrated in a growth-chamber study, drought-susceptible genotypes were more adversely affected by simultaneous application of enhanced UV-B radiation and drought conditions than the drought-tolerant genotype.27 However, the response may differ under natural environments. When either drought or enhanced UV-B radiation is first supplied singly, the negative effects may be lessened when followed by the other stress.27 Similarly, pre-exposure of plants to enhanced levels of UV-B radiation before exposure to high levels of visible light and high temperatures results in an increase in photosynthesis and relative growth.8 Pre-exposure to relatively high temperatures can also induce UV tolerance.28
These studies are of relevance particularly for horticultural practices, where the vitality of plants can be increased through exposure to the relevant sequential stress prior to transfer from a greenhouse/nursery to open field conditions.
Fertiliser application and nitrogen deposition
The degree of complexity of plant and ecosystem responses to multiple environmental and climatic factors is becoming increasingly evident as research moves from single-stress experiments to more natural environmental conditions. This is exemplified by a number of recent field studies where application of fertiliser to plants exposed to enhanced UV radiation induced varied responses. Fertiliser can modify the effects of elevated temperature and UV-B radiation. For example, changes in some secondary metabolites, including certain phenolic compounds and alkaloids, occur, as was shown in Norway spruce (Picea abies L.).29 Furthermore, increased sensitivity towards enhanced UV-B radiation may result where fertiliser is applied in excess of recommended levels.30 This was manifested in a shift in biomass towards the shoots of field-grown radish plants, causing a loss in yield compared to growth with recommended levels of nitrogen (N), phosphorus (P), and potassium (K).30 In contrast, additional but not excessive N may alleviate the negative effects of UV-B radiation stress.31,32
Volatile organic compounds
Several environmental stresses, both biotic and abiotic, stimulate the emission of volatile organic compounds (VOCs) from plant tissues, partly as a protective mechanism.33,34 While a large diversity of VOCs are produced from terrestrial ecosystems, only a few of these compounds contribute substantially to the total emissions.35 So far, only a few studies have focused on co-occurring stresses33,36 to elucidate the potential interactive effects on VOC emissions. These stressors include UV radiation, drought, soil moisture, temperature, tropospheric ozone, and herbivore attack, presenting a challenge in terms of quantifying their individual and combined contributions to emissions of VOCs at a local, regional and global level. This complexity is further overlaid by the variable nature of emissions according to the type of plant. VOCs such as isoprene are potential contributors to increasing greenhouse gases37 and thus may modify atmospheric composition, including extending the lifetime of methane.38 For example, one of the major sources of tropospheric carbon monoxide (CO) is oxidation of isoprene, and the scavenging of OH radicals by CO reduces the oxidation rate of methane, increasing its lifetime.
Isoprene, the most environmentally important VOC emitted from terrestrial plants, contributes to ca. 50% of the total global biogenic VOCs.35 While UV-B radiation and temperature can have interactive effects on VOC emissions, temperature is the more significant driver of increasing emissions. Those factors that stimulate VOC emissions, including UV radiation,39 result in an energy-cost to plants with a potential loss in productivity as a result of allocation of energy and carbon to, for example, the synthesis of isoprene.33,40 The extent to which UV radiation plays a stimulatory role in VOC emissions is currently unknown.
VOCs from a range of terrestrial and certain marine systems have been widely measured, including in extreme environments such as the Arctic regions and deserts. The effects of enhanced UV-B radiation on emissions of VOCs from sub-Arctic peatlands are highly variable, even when averaged over a growing season, ranging from no detectable change to 60% greater compared to ambient UV-B treatments.41–43 Thus, emissions of VOCs from plants and peatlands reflect the prevailing environmental conditions, including temperature, availability of water and UV radiation,39,44,45 and can lead to further interactions and feedbacks within the biosphere.
Methane, the second most important greenhouse gas after carbon dioxide, is produced by both microbial and non-microbial mechanisms as well as geological processes. Methane is emitted from peatlands and wetlands (Fig. 1) as well as from other vegetation types,45 soils, surface waters, animals, and fungi (Wang et al.46 and references therein). Microbially-produced methane has been well documented (Wang et al.46 and references therein), while methane production from oxygen-rich environments (non-microbial production) has only recently been investigated and its implications discussed. Recent estimates of emission of methane from plants indicate that this may be very low, although these estimates have varied substantially (e.g. contributing <0.2% to 40% of all methane released to the atmosphere).47–50 The source of methane emitted from vegetation is still under investigation, with reports suggesting emission is from an internal, plant structural cell wall component, pectin,51 or from surface waxes of leaves, the production of which may be stimulated by UV radiation.52 Additional factors leading to the stimulation of methane emissions include production of reactive oxygen species induced by environmental stress and injury to plants.53–55
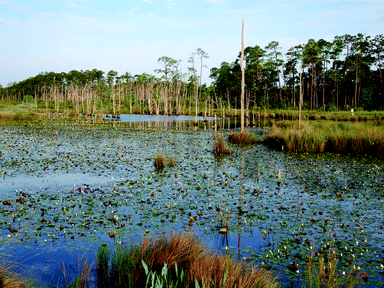 |
| Fig. 1 Wetland ecosystems, such as in south-eastern Louisiana, USA, are important sources of methane and have been the subject of a number of studies examining the effects of UV radiation on methane emissions (photograph: P. Barnes). | |
As is the case for many of the non-methane VOCs, the magnitude of methane emissions is variable and dependent on location and ambient conditions including UV radiation. A long-term study over 6 years showed that enhanced UV-B radiation in a boreal peatland increased methane emissions.45 A complementary study on the emission of methane from trees growing in natural wetlands showed that emissions from trees exceeded those of the peatland on which they were growing. These studies found that wetland trees mediated emission of methane from the peatland.56 In contrast, a study of a wetland (fen) in Northern Finland over three growing seasons did not show UV-B induced changes in net emission of methane, although enhanced UV-B radiation contributed to a slight increase in the organic acid precursors for methanogenesis.57 In field experiments specifically studying the response of rice plants and paddy fields to enhanced UV-B radiation, UV-B supplementation using lamps significantly increased the emission of methane from the paddy, especially between the tillering and heading stages of the rice. At the same time, decreased tiller number and biomass occurred as a response to the enhanced UV-B radiation levels.58
The important roles played by VOCs in ecosystem functioning – from inter- and intra-plant communications to plant chemical defence that decreases damage by herbivores – need to be analysed within a dynamic environment of interacting stresses from rapid and frequent climate events and changes in exposure to UV radiation. These factors should be considered when addressing the roles of different VOCs in mediating the diverse range of interactions occurring in ecosystems. In general, further investigations under natural environmental conditions are required to clarify the overall significance of UV radiation as a contributing factor to VOC emissions from plants and peatlands.
The ozone ‘hole’ as a driver for Southern Hemisphere climate and ecosystem change
The role of ozone depletion in Southern Hemisphere climate processes59 has been largely overlooked in the biological literature and this section highlights some of the ways ozone depletion, independent of UV radiation, has affected ecosystems through climatic change. While these climate perturbations are likely to have had a significant impact over the past few decades they have only recently started to be considered at the ecological level.
The ozone ‘hole’ moderates Southern Hemisphere climate
Ozone depletion and our response to it have had major implications for the Earth's climate. In the Southern Hemisphere, the ozone ‘hole’ has been a dominant driver5–7 of atmospheric circulation changes since the 1970s and has shielded Antarctica from much of the effects of global warming.6,60–63
The Southern Hemisphere Annular Mode (SAM), or Antarctic Oscillation, refers to the difference in atmospheric pressure between the mid- and high-latitudes of the Southern Hemisphere and thus the position of the polar jet. When the SAM index is positive the polar jet is located towards the South Pole and when it is negative it moves northwards. Since the late 1970s, the atmospheric polar jet has shifted south by 1–2° of latitude and increased in strength by 15–20%.64,65 The observed trend in the SAM has been largest during the austral summer and this is believed to be driven primarily by the development of the Antarctic ozone ‘hole’ (Thompson et al.5 and references therein). From records of ice cores it appears that intensification of the westerlies started over a century ago, driven by increases in greenhouse gases, but in recent decades the intensification associated with ozone depletion has been more pronounced.66 The SAM index is now at its highest level for at least 1000 years.67
Climate-related effects of ozone depletion on Southern Hemisphere ecosystems
The changes to circumpolar westerly winds attributed to ozone depletion are likely to have far-reaching consequences for biological ecosystems, particularly due to the influence of wind on the availability of water. The availability of liquid water to organisms broadly depends on the balance between annual precipitation and losses by evaporation, sublimation, and freezing. Increased wind causes evaporation, and in polar and alpine environments, sublimation and scouring of snow.68 In Antarctica, increased wind speeds have been linked to decreased growth rates of plants69 and changes in biodiversity in lakes70 in East Antarctica (Fig. 2). These changes are correlated with declining availability of water in East Antarctic coastal sites associated with increasing wind speeds and, in the case of moss growth rates, ozone depletion (Fig. 2). This research thus links ozone depletion to negative biodiversity outcomes through factors other than increasing UV-B radiation (reviewed in Robinson and Erickson7).
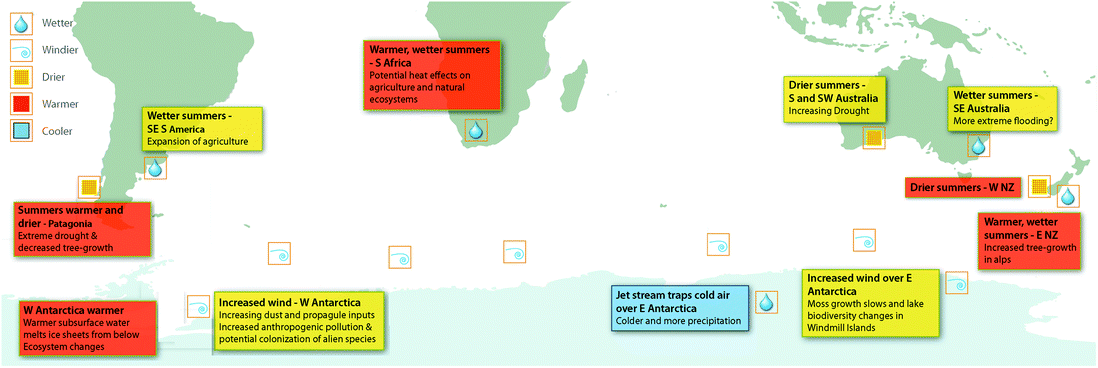 |
| Fig. 2 The Southern Hemisphere showing the impacts of the positive phase of the Southern Annular Mode (SAM) on atmospheric circulation, wind patterns and precipitation, as well as oceanic currents and temperatures. The associated effects of these climate changes on terrestrial ecosystems are shown where available. Over the past century, increasing greenhouse gases and then ozone depletion over Antarctica have both pushed the SAM towards a more positive phase and the SAM index is now at its highest level for at least 1000 years.67 During the summer months the positive phase of the SAM is strongly associated with ozone depletion. NZ, New Zealand. The figure, produced by Andrew Netherwood, has been modified from Robinson et al.7 | |
Winds also transport dust (including nutrients, seeds, spores, and other reproductive structures) from lower latitudes into the Southern Ocean and central West Antarctica (Fig. 2). Changes to either the location or strength of winds can affect the sources of dust and the quantities transported, with widespread implications including changes in productivity of the ocean. For example, increased wind-blown iron deposited into the ocean leads to phytoplankton blooms, indicative of increased productivity.71,72
These biological impacts are not restricted to the Antarctic. Significant changes to tree growth across the Southern Hemisphere in the last 50 years, relative to the previous 250 years, correlate with ozone ‘hole’-influenced changes to the SAM73 (Fig. 2). This is illustrated by the ca. 50% decline in growth rates of three species of trees (Austrocedrus chilensis, Araucaria araucana and Nothofagus betuloides) in southernmost South America since the 1950s, associated with SAM-induced decreased precipitation in the Andes. Similarly, changes to circulation patterns have increased precipitation over sub-alpine areas of New Zealand resulting in greater than average rates of growth in another tree species (Halocarpus biformis). In this case, a third of the growth increase was attributed to changes in the SAM.73
Recent modelling studies suggest that stratospheric ozone losses since the 1970s have also increased the frequency and intensity of extreme precipitation in austral summer.74,75 This has resulted in drying at the southern tip of South America and SW Australia, wetter summers in SE Australia, E New Zealand and SE South America and increased precipitation and freshening (increase in freshwater input) in the Southern Ocean76 (Fig. 2). Given the importance of water availability for all life on Earth, the vital role it plays in human and ecosystem health and for food security, these findings suggest that ozone depletion has far greater ecosystem impacts than previously anticipated. In particular, extremes of precipitation (droughts and floods) can be economically and socially devastating (cf. IPCC77).
Changes to the Southern Hemisphere circulation processes can have wider modifying effects than simply on wind speeds and associated water availability. It has been suggested that the shift to warmer summers in Southern Africa strongly correlates with the large ozone ‘hole’ era (1993–2010).78 Manatsa et al.78 re-analysed satellite data, focusing on October–December, to separate the effect of greenhouse gases on the SAM index from effects attributed to the ozone ‘hole’ (Fig. 2). While this analysis does not specifically link to biological impacts, it illustrates the potential effects of the ozone ‘hole’ in terms of human health, natural ecosystems, and agriculture. It also illustrates the need to investigate the role of the ozone ‘hole’ in ecological processes and systems other than those directly related to changes in UV radiation. Since ozone depletion is driving multiple stressors across the Southern hemisphere (e.g. increased UV-B radiation combined with either increased drought or increased precipitation), the interactive effects described above need to be considered.
Recovery of the ozone ‘hole’ over the next century will have widespread and complex effects on Southern Hemisphere climate processes with counter forcing from greenhouse gas emissions predicted to play a pivotal role.79 Contrary to expectations, some consequences of ozone depletion have been positive, such as maintaining Antarctica's cold temperatures, and their reversal may have negative effects for life on Earth. A more holistic picture of the true ecological consequences of ozone depletion on terrestrial, aquatic and marine ecosystems in the Southern Hemisphere is required in order to better project future changes and ways of mitigating risk.7
UV-B radiation and litter decomposition
Decomposition of organic material is a crucial component of global biogeochemical cycles that affects soil fertility, the fate and residence times of carbon and nutrients in organic matter pools, and ultimately plant community composition and production.43
Although the activity and make-up of the decomposing microbial community (bacteria and fungi) are key determinants of decomposition, solar radiation, including the UV-B component, plays a significant role. Recent studies, including several meta-analyses,80,81 have increased our understanding of the ecological significance of UV-B radiation on decomposition, including the fundamental mechanisms by which UV-B (and UV-A) radiation modifies the decomposition process, and the potential linkages between changes in UV radiation and other climate change factors. These analyses indicate that UV-B radiation, at ambient or enhanced levels, has complex effects on decomposition of litter and can either retard or accelerate rates of decomposition depending on UV-B exposure, climatic factors (e.g. temperature and precipitation), and litter chemistry and structure. The conditions and ecosystems where UV-B radiation is expected to play a significant role in litter decomposition are described below.
UV exposure of litter and wavelength sensitivity of decomposition processes
Ultimately, the effectiveness of incident solar UV radiation on the decomposition of litter is determined largely by exposure to UV and the sensitivity of the underlying decomposition processes to the wavelength (Fig. 3). At present, little is known of the precise nature of the dose–response relationships for the various mechanisms of UV-driven decomposition and what action spectra best describe the sensitivity of these processes. This makes it difficult to quantitatively assess the importance of the effects of ozone depletion and associated changes in UV radiation on the decomposition of litter, storage of carbon by ecosystems and the emissions of CO2 and other trace gases from decomposing plant litter. Recent studies have highlighted the importance of factors such as cloud cover,1,82 vegetation structure,82 litter depth,83 litter orientation,84 and soil coverage85 in altering the exposure of litter to UV radiation (Fig. 3 and 4). Also, while several recent studies have shown that photodegradation can be driven by UV-A and visible radiation (photosynthetically active radiation; PAR, 400–700 nm) in addition to the UV-B component,86,87 little progress has been made in developing action spectra for specific biotic (microbial) and abiotic (photodegradation) decomposition processes (Barnes et al.82 and references therein; but see Gao and Garcia-Pichel88).
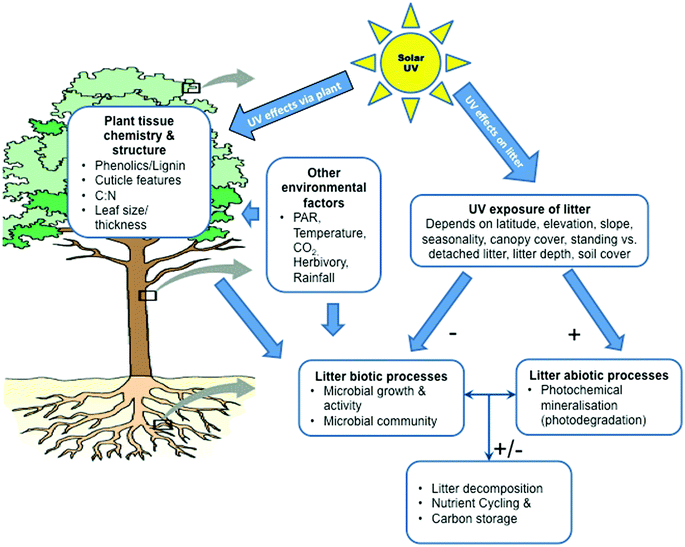 |
| Fig. 3 Conceptual model of the direct and indirect effects of solar UV radiation (290–400 nm) on the decomposition of terrestrial leaf litter, including potential interactions with other environmental factors. The total exposure of litter to UV radiation will depend on a combination of climatic, landscape/vegetation and species-specific factors. UV radiation generally reduces rates of biotic decomposition, whereas abiotic processes tend to enhance decomposition. Both processes exhibit distinct wavelength sensitivities (as seen from action spectra) depending on the underlying chromophores and mechanisms involved. Indirect effects of UV radiation are primarily mediated by those on leaf chemistry and structure, leading, e.g., to decreasing attractiveness of the litter for decomposing microbes. The role played by UV radiation on decomposition can depend also on other environmental factors interacting with biotic and abiotic processes and leaf chemistry and structure. The net effect of solar UV radiation on rates of decomposition, nutrient cycling and carbon storage will depend on the combined effects of biotic and abiotic processes and may be positive, negative or neutral. PAR, photosynthetically active radiation (400–700 nm). | |
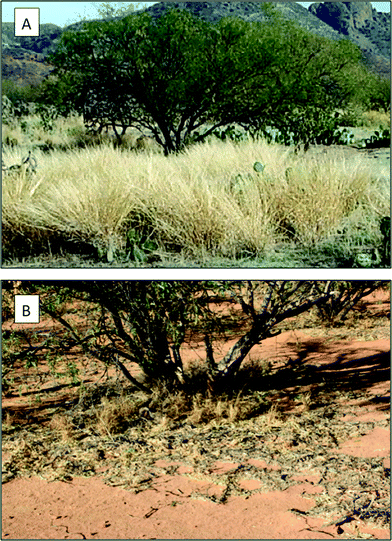 |
| Fig. 4 Temporal and spatial aspects of litter production and distribution in a semi-desert savanna in the Sonoran Desert, southern Arizona, USA. A. End-of-growing season standing litter of the C4 grass, Heteropogon contortus with the winter-deciduous shrub, Prosopis velutina before leaf drop. At this time of year there is significant potential for photodegradation of the standing litter of the grass. B. Spatial variation in bare ground, surface litter accumulation and light conditions under and near a P. velutina canopy after leaf drop and prior to the onset of the growing season. Once litter reaches the ground it can be redistributed across the landscape and mixed with soil such that microbial-driven decomposition increases while photodegradation decreases (photograph: S. Archer). | |
Effects of UV radiation on decomposer microorganisms
Solar UV-B radiation affects litter decomposition in terrestrial ecosystems via several mechanisms including direct effects on microbes and abiotic photochemistry (photodegradation), as well as through indirect effects mediated through alterations in leaf chemistry (Fig. 3; Barnes et al.82) These mechanisms can interact with one another and multiple pathways of decomposition can occur within a given process (see below). These processes are difficult to differentiate under field conditions. Hence, the quantitative importance of individual effects is largely unknown. In general, UV-B radiation tends to have negative effects on the growth, survival, and reproduction of microbes, which then retards rates of decomposition (Fig. 3; see also ref. 1, 82, 89). However, microbial species vary in their sensitivity to UV-B radiation, as evidenced by studies demonstrating insensitivity of certain bacterial and fungal species to UV-B radiation in extreme environments.90,91 Consequently, the overall effect of solar UV radiation (UV-B + UV-A) on the community composition of microbial decomposers is complex92,93 and requires a multi-criteria approach.
UV radiation and photodegradation of litter
The abiotic process of photodegradation occurs via photochemical mineralisation of photo-reactive compounds, such as lignin, and/or the transformation of compounds as a result of UV-induced formation of reactive oxygen species and other intermediates.80,86 In addition, apparent photodegradation is enhanced in the presence of oxygen but can also occur under anoxic conditions,4 indicative of the involvement of multiple chemical pathways.80 Generally, rates of photodegradation tend to increase with increasing moisture content of litter87,94 and air temperature.4
The effects of UV radiation on biotic (microbial) and abiotic (photodegradation) processes are not entirely independent and there is evidence that photodegradation can modify or partially degrade compounds in ways that enhance or retard subsequent microbial decomposition of litter (i.e. photopriming95,96) Thus, even when direct photodegradation has a minor effect on the loss of mass of litter, subsequent biological turnover can be positively correlated with the length of prior exposure to radiation.95 Photopriming may be of particular importance in the “conditioning” of litter prior to its detachment from living vegetation (i.e. “standing litter”; Fig. 4) and incorporation into the soil.86 However, photopriming can enhance carbon mineralisation from organic matter at the soil surface.97 In addition, susceptibility to photopriming will vary among plant species. Future photopriming experiments with multiple species in field situations are needed to assess whether this is a frequent or important facet of the photodegradation processes.
Effects of UV radiation on decomposition mediated by plants
Solar UV-B radiation can alter the chemistry and structure of living plant tissue, which then makes subsequent litter less suitable for the growth of decomposing microbes (Fig. 3; see also ref. 82 and 89). These UV-induced changes in plant tissue chemistry generally involve stimulation of the production of phenolic compounds that function in the protection of plants against UV radiation,13,98 although concentrations of other chemical constituents (e.g. C, N, P, K, lignin, C
:
N) can change as well.89 UV-B-induced changes in leaf chemistry need further evaluation for their potential role in photodegradation.
Interactive effects of UV radiation, climate and vegetation on decomposition
Photodegradation results in the efflux of a number of gases, including CO2,4,99 CH4,49,51 carbon monoxide (CO),4,87 and nitrous oxide (N2O).95 Analyses suggest that photodegradation of surface litter can have measurable effects on landscape-level rates of the flux of CO2, and ultimately carbon storage.99,100 The potential therefore exists for significant involvement of UV-driven photodegradation in influencing atmospheric CO2 levels and carbon sequestration, at least in certain ecosystems. The magnitude of these effects, however, is at present uncertain.
Under field conditions, the effects of UV-B radiation on microbial decomposition and photodegradation usually occur simultaneously, such that the net impact of UV-B radiation will reflect the balance of the biotic and abiotic mechanisms82,94 (Fig. 3). Consequently, UV-B radiation can have a positive, negative or neutral effect on decomposition, depending on environmental conditions and quality of the litter. Under dry conditions, exposure to UV-B radiation tends to increase decomposition via photodegradation. In contrast, where litter is exposed to UV-B radiation under moist conditions, rates of decomposition of litter generally decrease. This could reflect the inhibition of microbial activity by the UV-B radiation. Results from a recent meta-analysis of 93 field and laboratory studies conducted across six biomes (natural environmental communities), revealed the complex role of UV-B radiation in the decomposition of litter.81 Investigators reported that the direct effects of elevated and ambient UV-B radiation tended to increase the rate of decomposition (7 to 23%). However, the indirect effects (i.e. plant-mediated effects) were variable in magnitude and direction (−7 to 12%) depending on exposure to UV radiation. None of these positive and negative changes were statistically significant, however. Overall, the effects of UV-B radiation on litter decomposition are influenced by exposure to UV-B radiation, climatic factors (temperature and precipitation), and litter chemistry. While these findings are generally consistent with our understanding of the role of UV-B radiation on decomposition rates, they do indicate that detecting a statistically significant effect of UV-B radiation on the decomposition of litter may often be difficult within the limits of field experiments.
In arid and semi-arid climates (referred to as dryland ecosystems), UV-B radiation has significant and measurable impacts on decomposition (Fig. 4). These ecosystems typically receive large solar UV radiation fluxes at ground level, high temperatures, and low and highly variable precipitation-conditions that tend to shift the balance of effects of UV-B radiation on decomposition in favour of abiotic photodegradation processes. Photodegradation is now being considered as an important process affecting carbon cycling and storage in these systems. However, most studies in drylands have not explicitly considered factors that routinely alter the exposure of litter to UV radiation (e.g., litter depth, soil-litter mixing and litter movement across the landscape). While such studies may reasonably determine the decomposition of attached standing plant litter, their extrapolation to decomposition of detached plant litter on soil surfaces fails to account for these factors that can strongly mediate or even negate the abiotic effects. Thus, use of more realistic field conditions in experiments would serve to increase understanding and allow a more rigorous quantification of the role of solar UV-B and UV-A radiation on the decomposition of litter and biogeochemistry. In general, these findings highlight the linkages between changes in UV-B radiation, climate, and land-cover and suggest that these may alter the magnitude and even the direction of the effect of UV-B radiation on decomposition in terrestrial ecosystems.
It is evident that the degree of photodegradation varies with plant species and chemical composition of the litter.4,101 A meta-analysis of data from 50 field studies largely from dryland ecosystems80 showed that loss of mass due to photodegradation alone is, on average, 23%, but considerable variation occurs in rates among and within study sites. The variation in photodegradation was related to structural and chemical attributes of litter (area
:
mass and C
:
N ratios but not lignin content), precipitation, and exposure to solar radiation. This suggests that photodegradation may be an important but historically overlooked mechanism of decomposition in these systems and may account for the discrepancies between measured rates of decomposition and those predicted from ecosystem models based largely on climatic factors (temperature and precipitation) and initial litter quality.80,102–104 However, the role of the UV-B radiation component may be less effective than that of short wavelength visible radiation, as suggested by the meta-analysis of King et al.,80 although more evidence is needed to confirm this.
Elevated UV-B radiation and deposition of N also affect decomposition of plant litter, an important aspect of nutrient cycling.43 A field experiment in China showed that a combination of UV radiation and N deposition markedly increased the rate of litter decomposition under bamboo stands.105 Also, loss of carbon and phosphorus, and lignin degradation were promoted. Nitrogen deposition changes the availability of N in soil as well as the enzymatic activity of soil microbes such that increasing N can stimulate decomposition of litter. In contrast, enhanced UV-B radiation may decrease release of N from plant litter, probably through a direct effect on the microbial decomposers. Opposite outcomes of the effects of UV-B radiation and deposition of N have also been documented where the type of litter and ambient environmental conditions differ.105
UV radiation, soil organisms and belowground processes
Previous assessments have reported that although UV radiation does not penetrate soil to any great extent, a variety of soil-dwelling organisms and processes below ground can be modified indirectly via the effects of UV radiation on plants and plant litter. This occurs through UV-B-induced alterations in the chemistry of the leaf, which in turn can affect the physiology and composition of species of decomposer microorganisms (bacteria and fungi) in soil. There is considerable variation in these responses, depending on species, ecosystem type and developmental stages of the associated plants.1,106,107
While certain compounds in roots, such as flavonoids, can change in response to aboveground exposure of plants to UV-B radiation,108 a number of interactions in the soil immediately around root systems (rhizosphere) are mediated by flavonoids present in the exudates of roots.109 These include signalling between the root and Rhizobium (N-fixing bacteria), mycorrhizal infection, and plant–plant allelochemistry (release of biochemicals by plants that may be harmful or beneficial, i.e. allelopathy).110 Solar UV radiation has been implicated in playing a role in altering competitive interactions between invasive and native plant species by increasing the negative allelopathic effects of exotic species on native species.111 In field studies,112 exclusion of solar UV radiation significantly increased root growth, root nodulation, nitrogenase activity, and the leghaemoglobin and hemechrome contents in root nodules, suggesting a negative effect on the fixation of nitrogen in certain species under ambient levels of solar radiation. The potential exists for significant modifications in soil invertebrate communities in Antarctic and Arctic ecosystems currently experiencing appreciable ozone depletion and climate change. Reduction in herbivory by insects under solar UV-B radiation would be expected to be driven largely by differences arising from plant biochemistry and composition of vegetation rather than by direct effects of elevated UV-B radiation on these organisms.113 Although additional studies are clearly needed, these findings suggest a role for UV-B radiation in several belowground processes, which would have important consequences for mineral-nutrition of plants, storage of carbon in soil, biogeochemical cycles, and composition of plant species.
Implications of UV-induced changes in plant defence systems
Exposure of plants to solar UV-B radiation leads to an increase in several secondary metabolites that play a key role in the interactions of plants with other organisms, including herbivorous insects and microbial pathogens (Paul et al.,93 reviewed in Ballaré et al.114). Reduction in herbivory by insects under solar UV-B radiation is well documented in field-grown plants1 with the majority (>80%) of such studies reporting increases in plant damage or insect growth when solar UV-B radiation is experimentally reduced.115,116 Direct avoidance of UV radiation by many insects partly accounts for the reduction in herbivory.117,118 There are also some indications that, under ambient levels of UV-B radiation, infection of plants by pathogens is reduced.119,120 Pretreatment of plants with UV-B radiation before inoculation with a pathogen can also increase resistance to infection.120
Some of the increased production of secondary metabolites by UV-B radiation that boost plant defenses against pests includes leaf phenolics,120–122 conjugated polyamines,122 diterpenes123 and, in some cases, defense-related proteins such as proteinase inhibitors.122,124,125 On the other hand, phenolic compounds induced by UV-A radiation may modulate the responses to UV-B radiation via complex interactions between the UV-B photoreceptor, UVR8126,127 and UV-A/blue light signalling pathways.128 The functional relationships between UV-B radiation and the resistance of plants to pests (involving salicylic acid (SA) and jasmonic acid (JA) pathways) are not yet well characterised (for a review, see Ballaré et al.114), although there is increasing evidence that some of the effects of UV-B radiation on the resistance of plants to herbivory are mediated by increased JA signalling.122,123 In addition, UV-B radiation can affect plant defense against herbivores and pathogens via mechanisms that are not mediated by JA. For example, UV-B radiation, acting through UVR8, increases resistance of certain plants to the necrotrophic fungus Botrytis cinerea, and this effect is conserved in JA-insensitive genotypes.120
Protection and acclimation
Apart from plant defense mechanisms that protect against pests and disease, other UV-acclimative processes involving the accumulation of UV-absorbing compounds result in changes in plant optical properties (primarily epidermal transmittance, see Ballaré et al.1 and references therein). This acclimative response varies according to a wide range of prevailing conditions, including geographical and temporal, as well as with plant morphology (e.g. Ruhland et al.129) and increases understanding of the capacity of plants to respond to changing levels of UV radiation. Examples of dynamic acclimation include that of mature shade leaves of Populus tremuloides and Vicia faba where epidermal transmittance of UV radiation decreases when plants are transferred to sunny environments. Sun leaves, on the other hand, do not immediately respond by decreasing UV-absorbing pigments upon transfer to shady environments.130 This ability to increase but not decrease transmittance of UV radiation rapidly may be associated with the location of the UV-absorbing compounds. Compounds bound to cell walls are less likely to be re-mobilised than those inside the cell.131,132
Plants may also rapidly adjust their mechanism of UV-protection in response to daily changes in UV irradiances. Protection against UV radiation increases from dawn to midday and then decreases towards sunset.133 How plants achieve these rapid changes and what the significance is for function beyond protection from UV radiation is not yet known. Increased allocation of carbon to UV-absorbing compounds may divert carbon from growth and photosynthetic functions,134 such that reducing UV protection during times of the day when levels of UV radiation are low could enhance the daily gain of carbon. In comparison to plants that maintain high UV protection throughout the day, plants that exhibit diurnal changes in epidermal UV transmittance experience increased UV radiation exposure to the underlying mesophyll both early and late in the day, but not at midday. It is possible that increased penetration of UV radiation at these times may protect leaves from photoinhibition that can occur at midday.8 Since UV-A radiation can also drive photosynthesis,135 the increased penetration of UV-A radiation may enhance photosynthesis at times of the day when leaves are light-limited. Added to this, several of the compounds induced by UV radiation (e.g. quercetin, kaempferol) inhibit the plant growth regulator, auxin, and its transport within the plant.136 Thus, maintaining high concentrations of flavonoids could interfere with plant growth during night-time periods. Although the processes of acclimation of plants to UV radiation are complex, it is now clear that UV-absorbing acclimation involving UV-absorbers is much more flexible and dynamic than previously thought and has implications for the evaluation of plant hardiness under different environmental conditions.
Revisiting the potential for reconstructing past variations in stratospheric ozone and UV-B radiation
The relationships between ozone and climate change are complex59 and further investigation of the past nature of these relationships will lead to a better understanding of the physical interactions among solar activity, ozone and climate as well as more clarity on how ecosystems respond to changing UV-B radiation.137 The near ubiquitous response of plants to produce chemically stable UV-absorbing compounds, and thus achieve protection from UV-B radiation, means that plant tissues preserved in herbaria and in sedimentary and ice-core archives can be used to reconstruct past variations (prior to the instrumental period) in stratospheric ozone and UV-B radiation.138–140 In particular, UV-absorbing compounds bound to cell walls of plant tissues are extremely stable69 and may even persist in fossils.138,139 In addition to tracking past UV-B radiation, plant material is increasingly being assessed for its usefulness as a biological proxy for historical availability of water,141 temperature, and concentration of CO2 in the atmosphere (reviewed in Jordan142). Spores and pollen are common in the fossil record, and thus this type of proxy enables reconstruction of past UV-B radiation for providing spatial and temporal fidelity.140
UV-absorbing compounds (e.g. p-coumaric acid) in cell walls vary with latitude (and estimated exposure to UV-B radiation) in both spores of club mosses and in pine pollen. Greater variation is observed in samples collected from polar regions, where UV-B radiation has varied in recent decades due to ozone depletion, than in those collected from the tropics, where UV radiation levels have remained relatively stable.138,143 Similarly, UV-absorbing compounds in these same tissues show good temporal correlations with independent instrumental records and model results, allowing reconstruction of ozone concentration and flux of UV-B radiation over decades138 to millennia.143 However, further validation of proxies using UV-absorbing compounds requires development of UV radiation dose–response curves, as well as confirmation of the long-term chemical stability of the UV-absorbing compounds within paleobiological samples.139
Methodological advances, especially in microspectroscopy,138,140,143 allow analysis of very small samples (e.g. 50 grains of pine pollen). Such micro-scale analysis would also permit UV-absorbing compounds to be tracked down the length of shoots of slow growing individual plants such as polar mosses.69,141 Proxies that provide concurrent information on past climate and UV-B radiation, over centuries to millennia, would be especially valuable for greater understanding of past polar environments.
Implications of exposure to UV radiation and climate interactions for food production and food quality
Given the way in which solar UV radiation, stratospheric ozone, and climate variables have multiple and often interdependent effects on terrestrial and agricultural systems, it is logical that these effects will modify the development, production and crop quality of agricultural crops.9,144–146 However, there are currently few studies focusing on the interplay of climatic variables on crop quality.9,146 In contrast, many studies have reported both negative and positive effects of UV radiation and other climate variables on crop production. The type of response in the crop is largely dependent upon species and cultivar, geographical location, genetic differences, and the co-occurring environmental conditions (cf. ref. 1, 9, 147). Where UV-B radiation has had a negative effect on crop production, this has been manifested mainly as small decreases in biomass, and reduced leaf area (Ballaré et al.1 and references therein). As noted in previous sections, there are also indirect effects of UV-B radiation on plant growth such as decreased herbivory, due to increased secondary metabolites such as phenols, which is a positive plant effect.24,93,148
Many investigations have documented changes in biochemical and regulatory pathways in plants under ambient and enhanced UV-B radiation, although few studies have explicitly related these to follow-on effects on the quality of food crops.9,146 The UV-stimulated biochemical changes also contribute to differences in taste and aroma in, e.g., herbs such as mints.149 Several field studies have shown that ambient UV-B radiation increases concentrations of chemicals, e.g. flavonols, esters and fatty acids, which can enhance the aroma and flavour of wine.150–152 Terpene emission from grapes also increases with enhanced UV-B radiation and may be another modifier of quality.153
In general, a number of stress factors, including UV radiation, tend to increase the concentrations of proteins and antioxidants and reduce those of starch and lipids.144,154 While these biochemical changes often reflect the acclimative response of plants to enhanced levels of UV radiation and other environmental stresses, they may have either positive or negative consequences for the quality of crops in terms of nutrition and commercial use for specific products.
The degree to which plants can modulate acclimative response to often rapid changes in UV radiation is also of interest for agricultural and horticulture practices. A lag in this response has implications for UV susceptibility of crops that are propagated in low UV radiation environments (e.g. greenhouses) and are then subsequently transplanted to the field. This is illustrated by growth chamber studies with lettuce (Lactuca sativa), where new developing leaves require at least 6–8 days to fully acclimate to UV-B radiation and high visible light (PAR, photosynthetically active radiation, 400–700 nm).8,155
In terms of the beneficial effects of UV radiation on food crops, increased exposure to UV radiation through, for example, land-use changes and climate change, as well as manipulations of controlled growth conditions can be exploited to enhance food quality and nutritional value through induced changes in the secondary metabolism of plants.
Visual sensitivity to and damage from UV radiation in terrestrial animals
The range of wavelengths an animal perceives depends on the spectrum available in the environment, the degree to which this is transmitted through the ocular media and the visual pigments found in the retina. Visible light represents the spectrum perceived by humans but other animals often see a different range of “colours” due to visual pigments absorbing elsewhere in the spectrum. UV-vision is used extensively by a wide range of invertebrates and vertebrates for critical life processes including mate selection and location of food resources in birds, fish, insects, spiders, and other taxa. Some invertebrates are specifically able to detect and respond to UV-B radiation under natural conditions.118 The recent discovery that UV-vision (>300 nm) in mammals may be more widespread than previously recognised, suggests the need for more research into the ecological significance of this finding (Douglas and Jeffery,156 and references therein).
As with humans, animals can develop UV-related diseases, although research on these topics tends to be concentrated on economically important animals such as cattle. The occurrence of ocular squamous cell carcinoma (OSCC) or “cancer eye” has been reported in cattle worldwide, with 10–20% of animals in some Australian herds diagnosed with this disease. OSCC is the most common malignant tumour affecting cattle in North America and is responsible for significant economic losses (estimated at $20 million per annum in the United States alone; reviewed in Tsujita and Plummer157). In 2002, OSCC was the third-leading cause of carcass condemnation at slaughterhouses inspected by the US Department of Agriculture. European breeds of cattle, particularly those with light coloured skin such as Herefords and white-faced Holstein breeds, commonly develop OSCC, particularly when they are raised in regions with high natural levels of UV-B radiation (e.g. Australia, and the southwestern USA); see also Ballaré et al.1 While a proportion of the disease might be related to increasing UV radiation in areas affected by ozone depletion, the bulk appears to be caused by agricultural practices (e.g. lack of shade) and the movement of animals traditionally bred in low UV-B radiation environments to latitudes with higher UV-B radiation.
Progress in technical and experimental issues
Methodological issues in UV radiation supplementation studies, where filtered fluorescent UV lamps do not have a spectral output that perfectly matches the solar spectrum, were discussed previously.1 Briefly, Biological Spectral Weighting Functions (BSWF), dimensionless factors that represent the relative effectiveness of the different wavelengths of UV radiation in influencing a particular biological response, are used to calculate “biologically effective” UV radiation.59 This has been the only way to compare artificial UV radiation levels in experiments with solar UV radiation.
A new filter, termed the urate anion liquid filter, has been developed that permits fluorescent UV lamps to approximate sunlight much more closely.158 This new filter removes more of the shortwave UV-B radiation (λ ≤ 305 nm) than traditional cellulose diacetate filters and transmits more longwave UV-B (λ ≥ 310 nm). While this filter was developed for laboratory use in algal studies, there is potential for it to be adapted to other systems.
Accurate measurement of UV radiation is an essential aspect of experimental work on the effects of UV radiation.59 Ideally these measurements are done with a spectroradiometer, where radiation is quantified at discrete wavelengths. Usually, double-grating spectroradiometers are used for these measurements, where two gratings are linked in tandem to refine the signal sent to the detector. This double-grating system reduces “stray light” in the instrument. For example, a small amount of stray visible radiation could swamp radiation of a UV-B wavelength being measured. An instrumentation innovation that is rapidly supplementing the mechanical double-grating spectroradiometer is the diode-array spectroradiometer. This instrument has no moving parts and can produce a spectral irradiance measurement almost instantaneously. In contrast, typical mechanical double-grating units require several minutes to complete a scan, during which irradiation conditions can change.
Since diode-array spectroradiometers are much less costly than double-grating units, they may often replace broadband UV detectors in experimental studies. These spectral irradiance data will be much more valuable than the broadband measurements. The unit's small size and portability is also an asset. However, they have limitations that users need to take into account. Stray light is a considerable issue as these are basically single-grating spectroradiometers. Some features of the instrument can help minimise this problem, but measurements in full sunlight may still not be accurate. These issues are discussed at length by Aphalo et al.159 and references therein.
Sensitivity of instruments to temperature is also an issue, especially when conducting measurements in the field. A 15% change in the sensitivity of a double-grating spectroradiometer over the range of 11.5 to 33.5 °C can occur.160 Temperature also affects diode-array units as wavelength accuracy, dark current offset, and spectral responsivity are all influenced by temperature.161 It is possible in some cases to develop correction algorithms for particular units (e.g. Baczynska et al.160), although one must consider whether differential heating of the unit in direct intense sunlight can be adequately simulated in a test chamber. Ideally spectroradiometers used in measuring sunlight should be temperature stabilised.
Some ecological studies require measurements of time-integrated biologically effective UV radiation in environments where there is considerable spatial variability in UV irradiance (e.g. within plant canopies, soil surfaces under plant canopies and others). These applications require deployment of a number of inexpensive devices to measure the UV radiation. UV-absorbing polymers that were originally developed as human UV dosimeters59,162 have been used to characterise the UV radiation environment of individual leaves in canopies,163 as well as to quantify fine-scale differences in exposure of plants to UV radiation in heterogeneous habitats.164 While these devices are no substitute for spectroradiometers, they can provide useful and inexpensive estimates of exposure to UV radiation for certain applications.165 Current polymers have wavelength sensitivities similar to common plant biological weighting functions166,167 and have been developed with the inclusion of neutral density filters allowing estimates of exposure to UV radiation over periods of days to weeks.168 Provided these dosimeters are calibrated against spectroradiometers at the field locations where they are to be deployed, they may have particular utility for decomposition studies and in areas under the ozone ‘hole’ where long-term continuous measurement of the incident UV radiation on standing and ground level plant litter is needed.
Increased attention needs to be given to developing techniques and approaches that allow for the determination of realistic exposures to UV radiation of individual plants, natural ecosystems, and agricultural environments. The spectral sensitivities and exposures to UV radiation are needed in models that evaluate the combined effects of changes in UV radiation, climate, and vegetation on terrestrial ecosystems. The numerous technical issues involved in conducting experiments with UV radiation can, however, be intimidating to researchers starting work in this field. A comprehensive guide to all aspects of experimental design, implementation, analysis and instrumentation by Aphalo et al.159 will help standardize protocols and ensure reliable results that can be meaningfully assessed.
Gaps in knowledge
Since the last assessment,1 evidence of the coupling of ozone depletion effects and those of climate change2 has been strengthened, and has revealed the complexity of the interactions that are occurring. The success of the Montreal Protocol has been two-fold, viz., the phasing out of ozone depleting substances (ODS) and the contribution to decreasing some of the load of greenhouse gases, since many of the phased out ODS are themselves greenhouse gases. It should also be recognised that exposure to changing levels of UV radiation is not only ozone-dependent, but also reflects changes in land-use and climate-related phenomena, such as projected changes in rainfall, decreased or increased cloud cover in some regions, and snow and ice melting. These events are likely to affect ecosystem functioning and food production, all of which calls for a holistic approach to research that encompasses the role of UV radiation within a rapidly changing environment. From the research to date, evidence is accumulating that these interlinking factors of UV radiation, changes in ozone, climate, and environment are modifying responses of plants and ecosystems. Further evaluation of where the potential tipping points or beneficial effects are occurring will increase our understanding and ability to project potential future effects from the interactions between exposure to UV radiation and other simultaneously occurring environmental stresses. Currently, our knowledge of the consequences for the biosphere is far from comprehensive. What has become clear is that an integrative research approach under realistic conditions is essential for future projections of the response of ecosystems. For many of the processes discussed in this present paper, more empirical evidence is needed to determine the ecological significance of the role played by UV-B radiation in the presence of other environmental stresses.
Apart from the focus on UV radiation over more than 25 years of the Montreal Protocol, ozone depletion has been implicated in changes to the climate of the Southern Hemisphere, highlighting the need to assess the impact of such changes on the ecosystems of this region.7 One of the key uncertainties here is the extent to which climate is affected by ozone depletion versus greenhouse gas forcing and particularly the seasonality of these effects. Resolving this will improve understanding of how ozone recovery will feed back on these climate processes and is vital to our ability to model future ecosystems across half of the globe.
Acknowledgements
JFB acknowledges support from Curtin University, Western Australia; PWB was supported by the U.S. National Science Foundation (DEB 0815897) and Loyola University J.H. Mullahy Endowment for Environmental Biology; SAR was supported by the Australian Government, Department of Environment and the University of Wollongong. CLB was funded by grants from CONICET (Consejo Nacional de Investigaciones Científicas y Técnicas), ANPCyT (Agencia Nacional de Promoción Científica y Tecnológica) and UBACyT (Universidad de Buenos Aires Ciencia y Técnica).
References
- C. L. Ballaré, M. M. Caldwell, S. D. Flint, S. A. Robinson and J. F. Bornman, Effects of solar ultraviolet radiation on terrestrial ecosystems. Patterns, mechanisms, and interactions with climate change, Photochem. Photobiol. Sci., 2011, 10, 226–241 Search PubMed.
- C. E. Williamson, R. G. Zepp, R. M. Lucas, S. Madronich, A. T. Austin, C. L. Ballaré, M. Norval, B. Sulzberger, A. F. Bais, R. L. McKenzie, S. A. Robinson, D.-P. Häder, N. D. Paul and J. F. Bornman, Solar ultraviolet radiation in a changing climate, Nat. Clim. Change, 2014, 4, 434–441 CrossRef.
- R. M. Cory, B. C. Crump, J. A. Dobkowski and G. W. Kling, Surface exposure to sunlight stimulates CO2 release from permafrost soil carbon in the Arctic, Proc. Natl. Acad. Sci. U. S. A., 2013, 110, 3429–3434 CrossRef CAS PubMed.
- H. Lee, T. Rahn and H. L. Throop, An accounting of C-based trace gas release during abiotic plant litter degradation, Global Change Biol., 2012, 18, 1185–1195 CrossRef PubMed.
- D. W. J. Thompson, S. Solomon, P. J. Kushner, M. H. England, K. M. Grise and D. J. Karoly, Signatures of the Antarctic ozone hole in Southern Hemisphere surface climate change, Nat. Geosci., 2011, 4, 741–749 CrossRef CAS.
- J. Turner, N. E. Barrand, T. J. Bracegirdle, P. Convey, D. A. Hodgson, M. Jarvis, A. Jenkins, G. Marshall, M. P. Meredith, H. Roscoe, J. Shanklin, J. French, H. Goosse, M. Guglielmin, J. Gutt, S. Jacobs, M. C. Kennicutt Ii, V. Masson-Delmotte, P. Mayewski, F. Navarro, S. Robinson, T. Scambos, M. Sparrow, C. Summerhayes, K. Speer and A. Klepikov, Antarctic climate change and the environment: An update, Polar Rec., 2014, 50, 237–259 CrossRef.
- S. A. Robinson and D. J. Erickson III, Not just about sunburn–the ozone hole's profound effect on climate has significant implications for Southern Hemisphere ecosystems, Global Change Biol., 2014 DOI:10.1111/gcb.12739.
- J. J. Wargent, E. M. Elfadly, J. P. Moore and N. D. Paul, Increased exposure to UV-B radiation during early development leads to enhanced photoprotection and improved long-term performance in Lactuca sativa, Plant, Cell Environ., 2011, 34, 1401–1413 CrossRef CAS PubMed.
- J. J. Wargent and B. R. Jordan, From ozone depletion to agriculture: understanding the role of UV radiation in sustainable crop production, New Phytol., 2013, 197, 1058–1076 CrossRef CAS PubMed.
- W. J. Zhang and L. O. Björn, The effect of ultraviolet radiation on the accumulation of medicinal compounds in plants, Fitoterapia, 2009, 80, 207–218 CrossRef CAS PubMed.
- B. Mauch-Mani and F. Mauch, The role of abscisic acid in plant-pathogen interactions, Curr. Opin. Plant Biol., 2005, 8, 409–414 CrossRef CAS PubMed.
- D. Comont, J. Martinez Abaigar, A. Albert, P. Aphalo, D. R. Causton, F. L. Figueroa, A. Gaberscik, L. Llorens, M.-T. Hauser, M. A. K. Jansen, M. Kardefelt, P. de la Coba Luque, S. Neubert, E. Núñez-Olivera, J. Olsen, M. Robson, M. Schreiner, R. Sommaruga, Å. Strid, S. Torre, M. Turunen, S. Veljovic-Jovanovic, D. Verdaguer, M. Vidovic, J. Wagner, J. B. Winkler, G. Zipoli and D. Gwynn-Jones, UV responses of Lolium perenne raised along a latitudinal gradient across Europe: a filtration study, Physiol. Plant., 2012, 145, 604–618 CrossRef CAS PubMed.
- P. S. Searles, S. D. Flint and M. M. Caldwell, A meta-analysis of plant field studies simulating stratospheric ozone depletion, Oecologia, 2001, 127, 1–10 CrossRef.
- K. K. Newsham and S. A. Robinson, Responses of plants in polar regions to UVB exposure: a meta-analysis, Global Change Biol., 2009, 15, 2574–2589 CrossRef PubMed.
-
T. F. Stocker, D. Qin, G.-K. Plattner, M. Tignor, S. K. Allen, J. Boschung, A. Nauels, Y. Xia, V. Bex and P. M. Midgley, IPCC: Summary for Policymakers, in Climate Change 2013: The Physical Science Basis. Contribution of Working Group I to the Fifth Assessment Report of the Intergovernmental Panel on Climate Change, Cambridge University Press, Cambridge, UK and New York, NY, 2013 Search PubMed.
- F. Bussotti, F. Ferrini, M. Pollastrini and A. Fini, The challenge of Mediterranean sclerophyllous vegetation under climate change: From acclimation to adaptation, Environ. Exp. Bot., 2014, 103, 80–98 CrossRef PubMed.
- WMO, Scientific Assessment of Ozone Depletion: 2010, Global Ozone Research and Monitoring Project-Report No. 52, Report No., Geneva, Switzerland, 2011, p. 516.
- S. Madronich, M. Shao, S. R. Wilson, K. R. Solomon, J. Longstrethe and X. Tang, Changes in air quality and tropospheric composition due to depletion of stratospheric ozone and interactions with changing climate: Implications for human and environmental health, Photochem. Photobiol. Sci., 2015, 14 Search PubMed , this issue.
- L. Guidi, E. Degl'Innocenti, D. Remorini, S. Biricolti, A. Fini, F. Ferrini, F. P. Nicese and M. Tattini, The impact of UV-radiation on the physiology and biochemistry of Ligustrum vulgare exposed to different visible-light irradiance, Environ. Exp. Bot., 2011, 70, 88–95 CrossRef CAS PubMed.
- M. M. Caldwell, L. O. Björn, J. F. Bornman, S. D. Flint, G. Kulandaivelu, A. H. Teramura and M. Tevini, Effects of increased solar ultraviolet radiation on terrestrial ecosystems, J. Photochem. Photobiol., B, 1998, 46, 40–52 CrossRef CAS.
- J. D. Turnbull, S. J. Leslie and S. A. Robinson, Desiccation protects two Antarctic mosses from ultraviolet-B induced DNA damage, Funct. Plant Biol., 2009, 36, 214–221 CrossRef CAS.
- D. Verdaguer, L. Llorens, M. Bernal and J. Badosa, Photomorphogenic effects of UVB and UVA radiation on leaves of six Mediterranean sclerophyllous woody species subjected to two different watering regimes at the seedling stage, Environ. Exp. Bot., 2012, 79, 66–75 CrossRef PubMed.
- M. Bernal, L. Llorens, J. Badosa and D. Verdaguer, Interactive effects of UV radiation and water availability on seedlings of six woody Mediterranean species, Physiol. Plant., 2013, 147, 234–247 CrossRef CAS PubMed.
- C. A. Mazza, P. I. Gimenez, A. G. Kantolic and C. L. Ballaré, Beneficial effects of solar UV-B radiation on soybean yield mediated by reduced insect herbivory under field conditions, Physiol. Plant., 2013, 147, 307–315 CrossRef CAS PubMed.
- H. Bandurska, J. Niedziela and T. Chadzinikolau, Separate and combined responses to water deficit and UV-B radiation, Plant Sci., 2013, 213, 98–105 CrossRef CAS PubMed.
- H. Bandurska and M. Cieslak, The interactive effect of water deficit and UV-B radiation on salicylic acid accumulation in barley roots and leaves, Environ. Exp. Bot., 2013, 94, 9–18 CrossRef CAS PubMed.
- L. He, X. Jia, Z. Gao and R. Li, Genotype-dependent responses of wheat (Triticum aestivum L.) seedlings to drought, UV-B radiation and their combined stresses, Afr. J. Biotechnol., 2011, 10, 4046–4056 CAS.
- E. A. Kravets, L. B. Zelena, E. P. Zabara and Y. B. Blume, Adaptation strategy of barley plants to UV-B radiation, Emirates J. Food Agric., 2012, 24, 632–645 CrossRef.
- V. Virjamo, S. Sutinen and R. Julkunen-Tiitto, Combined effect of elevated UVB, elevated temperature and fertilization on growth, needle structure and phytochemistry of young Norway spruce (Picea abies) seedlings, Global Change Biol., 2014, 20, 2252–2260 CrossRef PubMed.
- S. Singh, R. Kumari, M. Agrawal and S. B. Agrawal, Modification in growth, biomass and yield of radish under supplemental UV-B at different NPK levels, Ecotoxicol. Environ. Saf., 2011, 74, 897–903 CrossRef CAS PubMed.
- T. S. L. Lau, E. Eno, G. Goldstein, C. Smith and D. A. Christopher, Ambient levels of UV-B in Hawaii combined with nutrient deficiency decrease photosynthesis in near-isogenic maize lines varying in leaf flavonoids: Flavonoids decrease photoinhibition in plants exposed to UV-B, Photosynthetica, 2006, 44, 394–403 CrossRef CAS PubMed.
- X.-R. Guo, B.-W. Chang, Y.-G. Zu and Z.-H. Tang, The impacts of increased nitrate supply on Catharanthus roseus growth and alkaloid accumulations under ultraviolet-B stress, J. Plant Interact., 2014, 9, 640–646 CrossRef.
- J. K. Holopainen and J. Gershenzon, Multiple stress factors and the emission of plant VOCs, Trends Plant Sci., 2010, 15, 176–184 CrossRef CAS PubMed.
-
M. Possell and F. Loreto, The role of volatile organic compounds in plant resistance to abiotic stresses: responses and mechanisms, in Biology, controls and models of tree volatile organic compound emissions, ed. Ü. Niinemets and R. K. Monson, Springer, Berlin, 2013, pp. 209–235 Search PubMed.
-
A. Guenther, Upscaling biogenic volatile compound emissions from leaves to landscapes, in Biology, controls and models of tree volatile organic compound emissions, ed. Ü. Niinemets and R. K. Monson, Springer, Berlin, 2013, pp. 391–414 Search PubMed.
- J. Peñuelas and M. Staudt, BVOCs and global change, Trends Plant Sci., 2010, 15, 133–144 CrossRef PubMed.
- M. J. Potosnak, B. M. Baker, L. LeStourgeon, S. M. Disher, K. L. Griffin, M. S. Bret-Harte and G. Starr, Isoprene emissions from a tundra ecosystem, Biogeosciences, 2013, 10, 871–889 Search PubMed.
- A. T. Archibald, J. G. Levine, N. L. Abraham, M. C. Cooke, P. M. Edwards, D. E. Heard, M. E. Jenkin, A. Karunaharan, R. C. Pike, P. S. Monks, D. E. Shallcross, P. J. Telford, L. K. Whalley and J. A. Pyle, Impacts of HOx regeneration and recycling in the oxidation of isoprene: Consequences for the composition of past, present and future atmospheres, Geophys. Res. Lett., 2011, 38, GL046520 CrossRef.
- J. Llusia, L. Llorens, M. Bernal, D. Verdaguer and J. Penuelas, Effects of UV radiation and water limitation on the volatile terpene emission rates, photosynthesis rates, and stomatal conductance in four Mediterranean species, Acta Physiol. Plant., 2012, 34, 757–769 CrossRef CAS.
- T. D. Sharkey, Is it useful to ask why plants emit isoprene?, Plant, Cell Environ., 2013, 36, 517–520 CrossRef CAS PubMed.
- P. Tiiva, R. Rinnan, P. Faubert, J. Räsänen, T. Holopainen, E. Kyro and J. K. Holopainen, Isoprene emission from a subarctic peatland under enhanced UV-B radiation, New Phytol., 2007, 176, 346–355 CrossRef CAS PubMed.
- P. Faubert, P. Tiiva, A. Rinnan, J. Räsänen, J. K. Holopainen, T. Holopainen, E. Kyrö and R. Rinnan, Non-methane biogenic volatile organic compound emissions from a subarctic peatland under enhanced UV-B radiation, Ecosystems, 2010, 13, 860–873 CrossRef CAS.
- D. J. Erickson III, B. Sulzberger, R. Zepp, A. T. Austin and N. Paul, Effects of stratospheric ozone depletion, solar UV radiation, and climate change on biogeochemical cycling: Interactions and feedbacks, Photochem. Photobiol. Sci., 2015, 14 Search PubMed , this issue.
- F. Loreto and J. P. Schnitzler, Abiotic stresses and induced BVOCs, Trends Plant Sci., 2010, 15, 154–166 CrossRef CAS PubMed.
- R. Rinnan, S. Saarnio, J. K. Haapala, S. K. Mörsky, P. J. Martikainen, J. Silvola and T. Holopainen, Boreal peatland ecosystems under enhanced UV-B radiation and elevated tropospheric ozone concentration, Environ. Exp. Bot., 2013, 90, 43–52 CrossRef CAS PubMed.
- Z. P. Wang, S. X. Chang, H. Chen and X. G. Han, Widespread non-microbial methane
production by organic compounds and the impact of environmental stresses, Earth-Sci. Rev., 2013, 127, 193–202 CrossRef CAS PubMed.
- F. Keppler, J. T. G. Hamilton, M. Brass and T. Rockmann, Methane emissions from terrestrial plants under aerobic conditions, Nature, 2006, 439, 187–191 CrossRef CAS PubMed.
-
A. McLeod and F. Keppler, Vegetation, in Methane and Climate Change, ed. D. S. Reay, P. Smith and A. van Amstel, Earthscan, London and Washington, D.C., 2010, pp. 74–96 Search PubMed.
- A. A. Bloom, J. Lee-Taylor, S. Madronich, D. J. Messenger, P. I. Palmer, D. S. Reay and A. R. McLeod, Global methane emission estimates from ultraviolet irradiation of terrestrial plant foliage, New Phytol., 2010, 187, 417–425 CrossRef CAS PubMed.
- R. G. Zepp, D. J. Erickson, N. D. Paul and B. Sulzberger, Effects of solar UV radiation and climate change on biogeochemical cycling: interactions and feedbacks, Photochem. Photobiol. Sci., 2011, 10, 261–279 CAS.
- A. R. McLeod, S. C. Fry, G. J. Loake, D. J. Messenger, D. S. Reay, K. A. Smith and B.-W. Yun, Ultraviolet radiation drives methane emissions from terrestrial plant pectins, New Phytol., 2008, 180, 124–132 CrossRef CAS PubMed.
- D. Bruhn, T. N. Mikkelsen, M. M. M. Rolsted, H. Egsgaard and P. Ambus, Leaf surface wax is a source of plant methane formation under UV radiation and in the presence of oxygen, Plant Biol., 2014, 16, 512–516 CrossRef CAS PubMed.
- D. Bruhn, I. M. Møller, T. N. Mikkelsen and P. Ambus, Terrestrial plant methane production and emission, Physiol. Plant., 2012, 144, 201–209 CrossRef CAS PubMed.
- F. Althoff, A. Jugold and F. Keppler, Methane formation by oxidation of ascorbic acid using iron minerals and hydrogen peroxide, Chemosphere, 2010, 80, 286–292 CrossRef CAS PubMed.
- D. J. Messenger, A. R. McLeod and S. C. Fry, The role of ultraviolet radiation, photosensitizers, reactive oxygen species and ester groups in mechanisms of methane formation from pectin, Plant, Cell Environ., 2009, 32, 1–9 CrossRef CAS PubMed.
- S. R. Pangala, S. Moore, E. R. C. Hornibrook and V. Gauci, Trees are major conduits for methane egress from tropical forested wetlands, New Phytol., 2013, 197, 524–531 CrossRef PubMed.
- S. K. Mörsky, J. K. Haapala, R. Rinnan, S. Saarnio, H. Suokanerva, K. Latola, E. Kyrö, J. Silvola, T. Holopainen and P. J. Martikainen, Minor long-term effects of ultraviolet-B radiation on methane dynamics of a subarctic fen in Northern Finland, Biogeochemistry, 2012, 108, 233–243 CrossRef.
- Y. Lou, W. Zhou and L. Ren, Elevated UV-B radiation increased CH4 emission in transgenic rice from a paddy soil, Agric., Ecosyst. Environ., 2012, 151, 16–20 CrossRef CAS PubMed.
- A. F. Bais, R. L. McKenzie, P. J. Aucamp, M. Ilyas, S. Madronich, G. Bernhard and K. Tourpali, Ozone depletion and climate change: Impacts on UV radiation, Photochem. Photobiol. Sci., 2015, 14 Search PubMed , this issue.
- Y. Wu, L. M. Polvani and R. Seager, The Importance of the Montreal Protocol in Protecting Earth's Hydroclimate, J. Clim., 2013, 26, 4049–4068 CrossRef.
-
J. Turner, R. A. Bindschadler, P. Convey, G. Di Prisco, E. Fahrbach, J. Gutt, D. A. Hodgson, P. A. Mayewski and C. P. Summerhayes, Antarctic Climate Change and the Environment, SCAR, Cambridge, 2009, http://acce.scar.org/wiki/Antarctic_Climate_Change_and_the_Environment Search PubMed, accessed 1/11/2014.
- E. R. Thomas, T. J. Bracegirdle, J. Turner and E. W. Wolff, A 308 year record of climate variability in West Antarctica, Geophys. Res. Lett., 2013, 40, 5492–5496 CrossRef.
- P. Convey, R. Bindschadler, G. di Prisco, E. Fahrbach, J. Gutt, D. A. Hodgson, P. A. Mayewski, C. P. Summerhayes and J. Turner, Antarctic climate change and the environment, Antarct. Sci., 2009, 21, 541–563 CrossRef.
- H. Korhonen, K. S. Carslaw, P. M. Forster, S. Mikkonen, N. D. Gordon and H. Kokkola, Aerosol climate feedback due to decadal increases in Southern Hemisphere wind speeds, Geophys. Res. Lett., 2010, 37, GL041320 CrossRef.
-
J. Turner and G. J. Marshall, Climate Change in the Polar Regions, Cambridge University Press, Cambridge, 2011 Search PubMed.
- D. A. Dixon, P. A. Mayewski, I. D. Goodwin, G. J. Marshall, R. Freeman, K. A. Maasch and S. B. Sneed, An ice-core proxy for northerly air mass incursions into West Antarctica, Int. J. Climatol., 2012, 32, 1455–1465 CrossRef.
- N. J. Abram, R. Mulvaney, F. Vimeux, S. J. Phipps, J. Turner and M. H. England, Evolution of the Southern Annular Mode during the past millennium, Nat. Clim. Change, 2014, 4, 564–569 CrossRef CAS.
- P. Convey, S. L. Chown, A. Clarke, D. K. A. Barnes, S. Bokhorst, V. Cummings, H. W. Ducklow, F. Frati, T. G. A. Green, S. Gordon, H. J. Griffiths, C. Howard-Williams, A. H. L. Huiskes, J. Laybourn-Parry, W. B. Lyons, A. McMinn, S. A. Morley, L. S. Peck, A. Quesada, S. A. Robinson, S. Schiaparelli and D. H. Wall, The spatial structure of Antarctic biodiversity, Ecol. Monogr., 2014, 84, 203–244 CrossRef PubMed.
- L. J. Clarke, S. A. Robinson, Q. Hua, D. J. Ayre and D. Fink, Radiocarbon bomb spike reveals biological effects of Antarctic climate change, Global Change Biol., 2012, 18, 301–310 CrossRef PubMed.
- D. A. Hodgson, D. Roberts, A. McMinn, E. Verleyen, B. Terry, C. Corbett and W. Vyverman, Recent rapid salinity rise in three East Antarctic lakes, J. Paleolimnol., 2006, 36, 385–406 CrossRef PubMed.
- M. Cataldo, H. Evangelista, J. C. Simoes, R. H. M. Godoi, I. Simmonds, M. H. Hollanda, I. Wainer, F. Aquino and R. Van Grieken, Mineral dust variability in central West Antarctica associated with ozone depletion, Atmos. Chem. Phys., 2013, 13, 2165–2175 CrossRef.
- J. R. McConnell, A. J. Aristarain, J. R. Banta, P. R. Edwards and J. C. Simões, 20th-Century doubling in dust archived in an Antarctic Peninsula ice core parallels climate change and desertification in South America, Proc. Natl. Acad. Sci. U. S. A., 2007, 104, 5743–5748 CrossRef CAS PubMed.
- R. Villalba, A. Lara, M. H. Masiokas, R. Urrutia, B. H. Luckman, G. J. Marshall, I. A. Mundo, D. A. Christie, E. R. Cook, R. Neukom, K. Allen, P. Fenwick, J. A. Boninsegna, A. M. Srur, M. S. Morales, D. Araneo, J. G. Palmer, E. Cuq, J. C. Aravena, A. Holz and C. LeQuesne, Unusual Southern Hemisphere tree growth patterns induced by changes in the Southern Annular Mode, Nat. Geosci., 2012, 5, 793–798 CrossRef CAS.
- C. Böning, A. Dispert, M. Visbeck, R. SR and F. Schwarzkopf, The response of the Antarctic Circumpolar Current to recent climate change, Nat. Geosci., 2008, 1, 864–869 CrossRef.
- A. Purich and S. W. Son, Impact of Antarctic ozone depletion and recovery on Southern Hemisphere precipitation, evaporation, and extreme changes, J. Clim., 2012, 25, 3145–3154 CrossRef.
- S. M. Kang, L. M. Polvani, J. C. Fyfe, S. W. Son, M. Sigmond and G. J. P. Correa, Modeling evidence that ozone depletion has impacted extreme precipitation in the austral summer, Geophys. Res. Lett., 2013, 40, 4054–4059 CrossRef.
- IPCC, Climate Change 2014: Impacts, Adaptation, and Vulnerability. Contribution of Working Group II to the Fifth Assessment Report of the Intergovernmental Panel on Climate Change. WGII AR5 Summary for Policymakers., Report No., IPCC, Geneva, Switzerland, 2014, p. 44.
- D. Manatsa, Y. Morioka, S. K. Behera, T. Yamagata and C. H. Matarira, Link between Antarctic ozone depletion and summer warming over southern Africa, Nat. Geosci., 2013, 6, 934–939 CrossRef CAS.
- J. Perlwitz, ATMOSPHERIC SCIENCE Tug of war on the jet stream, Nat. Clim. Change, 2011, 1, 29–31 CrossRef.
- J. Y. King, L. A. Brandt and E. C. Adair, Shedding light on plant litter decomposition: advances, implications and new directions in understanding the role of photodegradation, Biogeochemistry, 2012, 111, 57–81 CrossRef.
- X. Song, C. Peng, H. Jiang, Q. Zhu and W. Wang, Direct and indirect effects of UV-B exposure on litter decomposition: a meta-analysis, PLoS One, 2013, 8, e68858 CAS.
- P. W. Barnes, H. L. Throop, S. R. Archer, D. D. Breshears, R. L. McCulley and M. A. Tobler, Sunlight and soil-litter mixing: Drivers of litter decomposition in drylands, Prog. Bot., 2015, 76, 273–302 CrossRef.
- H. A. L. Henry, K. Brizgys and C. B. Field, Litter decomposition in a California annual grassland: Interactions between photodegradation and litter layer thickness, Ecosystems, 2008, 11, 545–554 CrossRef CAS PubMed.
- Y. Lin and J. Y. King, Effects of UV exposure and litter position on decomposition in a California grassland, Ecosystems, 2014, 17, 158–168 CrossRef CAS.
- P. W. Barnes, H. L. Throop, D. B. Hewins, M. L. Abbene and S. R. Archer, Soil coverage reduces photodegradation and promotes the development of soil-microbial films on dryland leaf litter, Ecosystems, 2012, 15, 311–321 CrossRef CAS.
- A. T. Austin and C. L. Ballaré, Dual role of lignin in plant litter decomposition in terrestrial ecosystems, Proc. Natl. Acad. Sci. U. S. A., 2010, 107, 4618–4622 CrossRef CAS PubMed.
- G. W. Schade, R. M. Hofmann and P. J. Crutzen, CO emissions from degrading plant matter (I). Measurements, Tellus, Ser. B, 1999, 51, 889–908 Search PubMed.
- Q. Gao and F. Garcia-Pichel, Microbial ultraviolet sunscreens, Nat. Rev. Microbiol., 2011, 9, 791–802 CrossRef CAS PubMed.
- X. Song, H. L. Zhang, H. Jiang, L. A. Donaldson and H. L. Wang, Influence of elevated UV-B radiation on leaf litter chemistry and subsequent decomposition in humid subtropical China, J. Soils Sediments, 2013, 13, 846–853 CrossRef CAS.
- L. Selbmann, D. Isola, L. Zucconi and S. Onofri, Resistance to UV-B induced DNA damage in extreme-tolerant cryptoendolithic Antarctic fungi: detection by PCR assays, Fungal Biol., 2011, 115, 937–944 CrossRef CAS PubMed.
- S. A. Alharbi, How do bacteria survive UV irradiation in the stratosphere?, J. Food Agric. Environ., 2012, 10, 843–845 CAS.
- H. Kadivar and A. E. Stapleton, Ultraviolet radiation alters maize phyllosphere bacterial diversity, Microb. Ecol., 2003, 45, 353–361 CrossRef CAS PubMed.
- N. D. Paul, J. P. Moore, M. McPherson, C. Lambourne, P. Croft, J. C. Heaton and J. J. Wargent, Ecological responses to UV radiation: interactions between the biological effects of UV on plants and on associated organisms, Physiol. Plant., 2012, 145, 565–581 CrossRef CAS PubMed.
- W. K. Smith, W. Gao, H. Steltzer, M. D. Wallenstein and R. Tree, Moisture availability influences the effect of ultraviolet-B radiation on leaf litter decomposition, Global Change Biol., 2010, 16, 484–495 CrossRef PubMed.
- B. Foereid, J. Bellarby, W. Meier-Augenstein and H. Kemp, Does light exposure make plant litter more degradable?, Plant Soil, 2010, 333, 275–285 CrossRef CAS PubMed.
- S. Ma, D. Baldocchi, J. Hatala, J. M. Detto and J. Yuste, Are rain-induced ecosystem respiration pulses enhanced by legacies of antecedent photodegradation in semi-arid environments?, Agric. For. Meteorol., 2012, 154–155, 203–213 CrossRef PubMed.
- L. M. Mayer, K. R. Thornton, L. L. Schick, J. D. Jastrow and J. W. Harden, Photodissolution of soil organic matter, Geoderma, 2012, 170, 314–321 CrossRef CAS PubMed.
- G. Agati, C. Brunetti, M. Di Ferdinando, F. Ferrini, S. Pollastri and M. Tattini, Functional roles of flavonoids in photoprotection: New evidence, lessons from the past, Plant Physiol. Biochem., 2013, 72, 35–45 CrossRef CAS PubMed.
- L. A. Brandt, C. Bohnet and J. Y. King, Photochemically induced carbon dioxide production as a mechanism for carbon loss from plant litter in arid ecosystems, J. Geophys. Res.: Biogeosci., 2009, 114, G02004 Search PubMed.
- S. Rutledge, D. I. Campbell, D. Baldocchi and L. A. Schipper, Photodegradation leads to increased carbon dioxide losses from terrestrial organic matter, Global Change Biol., 2010, 16, 3065–3074 Search PubMed.
- S. M. Uselman, K. A. Snyder, R. R. Blank and T. J. Jones, UVB exposure does not accelerate rates of litter decomposition in a semi-arid riparian ecosystem, Soil Biol. Biochem., 2011, 43, 1254–1265 CrossRef CAS PubMed.
- W. Parton, W. L. Silver, I. C. Burke, L. Grassens, M. E. Harmon, W. S. Currie, J. Y. King, E. C. Adair, L. A. Brandt, S. C. Hart and B. Fasth, Global-scale similarities in nitrogen release patterns during long-term decomposition, Science, 2007, 315, 361–364 CrossRef CAS PubMed.
- E. C. Adair, W. J. Parton, S. J. Del Grosso, W. L. Silver, M. E. Harmon, S. A. Hall, I. C. Burke and S. C. Hart, Simple three-pool model accurately describes patterns of long-term litter decomposition in diverse climates, Global Change Biol., 2008, 14, 2636–2660 Search PubMed.
- A. T. Austin, Has water limited our imagination for aridland biogeochemistry?, Trends Ecol. Evol., 2011, 26, 229–235 CrossRef PubMed.
- X. Song, H. Jiang, Z. Zhang, G. Zhou, S. Zhang and C. Peng, Interactive effects of elevated UV-B radiation and N deposition on decomposition of Moso bamboo litter, Soil Biol. Biochem., 2014, 69, 11–16 CrossRef CAS PubMed.
- M. M. Caldwell, J. F. Bornman, C. L. Ballaré, S. D. Flint and G. Kulandaivelu, Terrestrial ecosystems, increased solar ultraviolet radiation, and interactions with other climate change factors, Photochem. Photobiol. Sci., 2007, 6, 252–266 CAS.
- X. Bao, Q. Li, J. Hua, T. Zhao and W. Liang, Interactive effects of elevated ozone and UV-B radiation on soil nematode diversity, Ecotoxicology, 2014, 23, 11–20 CrossRef CAS PubMed.
- B. Li, A. Krumbein, S. Neugart, L. Li and M. Schreiner, Mixed cropping with maize combined with moderate UV-B radiations lead to enhanced flavonoid production and root growth in faba bean, J. Plant Interact., 2012, 7, 333–340 CrossRef CAS.
- S. Cesco, G. Neumann, N. Tomasi, R. Pinton and L. Weisskopf, Release of plant-borne flavonoids into the rhizosphere and their role in plant nutrition, Plant Soil, 2010, 329, 1–25 CrossRef CAS.
- S. Hassan and U. Mathesius, The role of flavonoids in root-rhizosphere signalling: opportunities and challenges for improving plant-microbe interactions, J. Exp. Bot., 2012, 63, 3429–3444 CrossRef CAS PubMed.
- S. Chen, S. Xiao and R. M. Callaway, Light intensity alters the allelopathic effects of an exotic invader, Plant Ecol. Diversity, 2012, 5, 521–526 CrossRef.
- S. Sharma and K. N. Guruprasad, Enhancement of root growth and nitrogen fixation in Trigonella by UV-exclusion from solar radiation, Plant Physiol. Biochem., 2012, 61, 97–102 CrossRef CAS PubMed.
- U. N. Nielsen and D. H. Wall, The future of soil invertebrate communities in polar regions: different climate change responses in the Arctic and Antarctic?, Ecol. Lett., 2013, 16, 409–419 CrossRef PubMed.
- C. L. Ballaré, Light regulation of plant defense, Annu. Rev. Plant Biol., 2014, 65, 335–363 CrossRef PubMed.
- C. L. Ballaré, C. A. Mazza, A. T. Austin and R. Pierik, Canopy Light and Plant Health, Plant Physiol., 2012, 160, 145–155 CrossRef PubMed.
- F. Kuhlmann and C. Müller, Impacts of ultraviolet radiation on interactions between plants and herbivorous Insects: A chemo-ecological perspective, Prog. Bot., 2011, 72, 305–347 CAS.
- C. A. Mazza, M. M. Izaguirre, J. Zavala, A. L. Scopel and C. L. Ballaré, Insect perception of ambient ultraviolet-B radiation, Ecol. Lett., 2002, 5, 722–726 CrossRef.
- C. A. Mazza, M. M. Izaguirre, J. Curiale and C. L. Ballaré, A look into the invisible. Ultraviolet-B sensitivity in an insect (Caliothrips phaseoli) revealed through a behavioural action spectrum, Proc. R. Soc. London, Ser. B, 2010, 277, 367–373 CrossRef CAS PubMed.
- T. S. Gunasekera, N. D. Paul and P. G. Ayres, The effects of ultraviolet-B (UV-B: 290–320 nm) radiation on blister blight disease of tea (Camellia sinensis), Plant Pathol., 1997, 46, 179–185 Search PubMed.
- P. V. Demkura and C. L. Ballaré, UVR8 Mediates UV-B-Induced Arabidopsis Defense Responses against Botrytis cinerea by Controlling Sinapate Accumulation, Mol. Plant, 2012, 5, 642–652 CrossRef PubMed.
- M. C. Rousseaux, R. Julkunen-Tiitto, P. S. Searles, A. L. Scopel, P. J. Aphalo and C. L. Ballaré, Solar UV-B radiation affects leaf quality and insect herbivory in the southern beech tree Nothofagus antarctica, Oecologia, 2004, 138, 505–512 CrossRef PubMed.
- P. V. Demkura, G. Abdala, I. T. Baldwin and C. L. Ballaré, Jasmonate-dependent and -independent pathways mediate specific effects of solar ultraviolet-B radiation on leaf phenolics and antiherbivore defense, Plant Physiol., 2010, 152, 1084–1095 CrossRef CAS PubMed.
- S. T. Đinh, I. Gális and I. T. Baldwin, UVB radiation and 17-hydroxygeranyllinalool diterpene glycosides provide durable resistance against mirid (Tupiocoris notatus) attack in field-grown Nicotiana attenuata plants, Plant, Cell Environ., 2013, 36, 590–606 CrossRef PubMed.
- J. W. Stratmann, B. A. Stelmach, E. W. Weiler and C. A. Ryan, UVB/UVA radiation activates a 48 kDa myelin basic protein kinase and potentiates wound signaling in tomato leaves, Photochem. Photobiol., 2000, 71, 116–123 CrossRef CAS.
- M. M. Izaguirre, A. L. Scopel, I. T. Baldwin and C. L. Ballaré, Convergent responses to stress. Solar ultraviolet-B radiation and Manduca sexta herbivory elicit overlapping transcriptional responses in field-grown plants of Nicotiana longiflora, Plant Physiol., 2003, 132, 1755–1767 CrossRef CAS PubMed.
- L. Rizzini, J. J. Favory, C. Cloix, D. Faggionato, A. O'Hara, E. Kaiserli, R. Baumeister, E. Schafer, F. Nagy, G. I. Jenkins and R. Ulm, Perception of UV-B by the Arabidopsis UVR8 Protein, Science, 2011, 332, 103–106 CrossRef CAS PubMed.
- G. I. Jenkins, The UV-B Photoreceptor UVR8: From Structure to Physiology, Plant Cell, 2014, 26, 21–37 CrossRef CAS PubMed.
- L. O. Morales, M. Brosché, J. Vainonen, G. I. Jenkins, J. J. Wargent, N. Sipari, A. Strid, A. V. Lindfors, R. Tegelberg and P. J. Aphalo, Multiple roles for UV RESISTANCE LOCUS8 in regulating gene expression and metabolite accumulation in Arabidopsis under solar ultraviolet radiation, Plant Physiol., 2013, 161, 744–759 CrossRef CAS PubMed.
- C. T. Ruhland, M. J. Dyslin and J. D. Krenz, Wyoming big sagebrush screens ultraviolet radiation more effectively at higher elevations, J. Arid. Environ., 2013, 96, 19–22 CrossRef PubMed.
- P. W. Barnes, A. R. Kersting, S. D. Flint, W. Beyschlag and R. J. Ryel, Adjustments in epidermal UV-transmittance of leaves in sun-shade transitions, Physiol. Plant., 2013, 149, 200–213 CrossRef CAS PubMed.
- L. J. Clarke and S. A. Robinson, Cell wall-bound ultraviolet-screening compounds explain the high ultraviolet tolerance of the Antarctic moss, Ceratodon purpureus, New Phytol., 2008, 179, 776–783 CrossRef PubMed.
- S. A. Robinson and M. J. Waterman, Sunsafe bryophytes: photoprotection from excess and damaging solar radiation, Adv. Photosynth. Respir., 2014, 37, 113–130 Search PubMed.
- P. W. Barnes, S. D. Flint, J. R. Slusser, W. Gao and R. J. Ryel, Diurnal changes in epidermal UV transmittance of plants in naturally high UV environments, Physiol. Plant., 2008, 133, 363–372 CrossRef CAS PubMed.
- S. Sumbele, M. N. Fotelli, D. Nikolopoulos, G. Tooulakou, V. Liakoura, G. Liakopoulos, P. Bresta, E. Dotsika, M. A. Adams and G. Karabourniotis, Photosynthetic capacity is negatively correlated with the concentration of leaf phenolic compounds across a range of different species, AoB PLANTS, 2012, 2012 DOI:10.1093/aobpla/pls025.
- T. L. Turnbull, A. Barlow and M. A. Adams, Photosynthetic benefits of ultraviolet-A to Pimelea ligustrina, a woody shrub of sub-alpine Australia, Oecologia, 2013, 173, 375–385 CrossRef PubMed.
- M. L. Falcone-Ferreyra, S. P. Rius and P. Casati, Flavonoids: biosynthesis, biological functions, and biotechnological applications, Front. Plant Sci., 2012, 3, 222 CAS.
- D. Magri, Past UV-B flux from fossil pollen: prospects for climate, environment and evolution, New Phytol., 2011, 192, 310–312 CrossRef PubMed.
- B. H. Lomax, W. T. Fraser, M. A. Sephton, T. V. Callaghan, S. Self, M. Harfoot, J. A. Pyle, C. H. Wellman and D. J. Beerling, Plant spore walls as a record of long-term changes in ultraviolet-B radiation, Nat. Geosci., 2008, 1, 592–596 CrossRef CAS.
- J. Rozema, P. Blokker, M. A. Mayoral Fuertes and R. Broekman, UV-B absorbing compounds in present-day and fossil pollen, spores, cuticles, seed coats and wood: evaluation of a proxy for solar UV radiation, Photochem. Photobiol. Sci., 2009, 8, 1233–1243 CAS.
- B. H. Lomax, W. T. Fraser, G. Harrington, S. Blackmore, M. A. Sephton and N. B. W. Harris, A novel palaeoaltimetry proxy based on spore and pollen wall chemistry, Earth Planet. Sci. Lett., 2012, 353–354, 22–28 CrossRef CAS PubMed.
- J. Royles, J. Ogée, L. Wingate, D. A. Hodgson, P. Convey and H. Griffiths, Carbon isotope evidence for recent climate-related enhancement of CO2 assimilation and peat accumulation rates in Antarctica, Global Change Biol., 2012, 18, 3112–3124 CrossRef PubMed.
- G. J. Jordan, A critical framework for the assessment of biological palaeoproxies: predicting past climate and levels of atmospheric CO2 from fossil leaves, New Phytol., 2011, 192, 29–44 CrossRef PubMed.
- K. J. Willis, A. Feurdean, H. J. B. Birks, A. E. Bjune, E. Breman, R. Broekman, J. A. Grytnes, M. New, J. S. Singarayer and J. Rozema, Quantification of UV-B flux through time using UV-B-absorbing compounds contained in fossil Pinus sporopollenin, New Phytol., 2011, 192, 553–560 CrossRef CAS PubMed.
- Y. X. Wang and M. Frei, Stressed food - The impact of abiotic environmental stresses on crop quality, Agric., Ecosyst. Environ., 2011, 141, 271–286 CrossRef PubMed.
- D. Gwynn-Jones, A. G. Jones, A. Waterhouse, A. Winters, D. Comont, J. Scullion, R. Gardias, B. J. Graae, J. A. Lee and T. V. Callaghan, Enhanced UV-B and Elevated CO2 Impacts Sub-Arctic Shrub Berry Abundance, Quality and Seed Germination, Ambio, 2012, 41(Suppl. 3), 256–268 CrossRef CAS PubMed.
- M. Schreiner, I. Mewis, S. Huyskens-Keil, M. A. K. Jansen, R. Zrenner, J. B. Winkler, N. O'Brien and A. Krumbein, UV-B-induced secondary plant metabolites - potential benefits for plant and human health, Crit. Rev. PlantSci., 2012, 31, 229–240 CrossRef CAS.
- B. Liu, X.-B. Liu, Y.-S. Li and S. J. Herbert, Effects of enhanced UV-B radiation on seed growth characteristics and yield components in soybean, Field Crops Res., 2013, 154, 158–163 CrossRef PubMed.
- J. A. Zavala, C. A. Mazza, F. M. Dillon, H. D. Chludil and C. L. Ballaré, Soybean resistance to stink bugs (Nezara viridula and Piezodorus guildinii) increases with exposure to solar UV-B radiation and correlates with isoflavonoid content in pods under field conditions, Plant, Cell Environ., 2014 DOI:10.1111/pce.12368.
- Y. Dolzhenko, C. M. Bertea, A. Occhipinti, S. Bossi and M. E. Maffei, UV-B modulates the interplay between terpenoids and flavonoids in peppermint (Mentha piperita L.), J. Photochem. Photobiol., B, 2010, 100, 67–75 CrossRef CAS PubMed.
- S. M. Gregan, J. J. Wargent, L. Liu, J. Shinkle, R. Hofmann, C. Winefield, M. Trought and B. Jordan, Effects of solar ultraviolet radiation and canopy manipulation on the biochemical composition of Sauvignon Blanc grapes, Aust. J. Grape Wine Res., 2012, 18, 227–238 CrossRef CAS PubMed.
- P. Carbonell-Bejerano, M. P. Diago, J. Martinez-Abaigar, J. M. Martinez-Zapater, J. Tardaguila and E. Nunez-Olivera, Solar ultraviolet radiation is necessary to enhance grapevine fruit ripening transcriptional and phenolic responses, BMC Plant Biol., 2014, 14, 183 CrossRef PubMed.
- K. Suklje, G. Antalick, Z. Coetzee, L. M. Schmidtke, H. B. Cesnik, J. Brandt, W. J. du Toit, K. Lisjak and A. Deloire, Effect of leaf removal and ultraviolet radiation on the composition and sensory perception of Vitis vinifera L. cv. Sauvignon Blanc wine, Aust. J. Grape Wine Res., 2014, 20, 223–233 CrossRef CAS PubMed.
- M. Gil, R. Bottini, F. Berli, M. Pontin, M. Fernanda Silva and P. Piccoli, Volatile organic compounds characterized from grapevine (Vitis vinifera L. cv. Malbec) berries increase at pre-harvest and in response to UV-B radiation, Phytochemistry, 2013, 96, 148–157 CrossRef CAS PubMed.
- F. J. Berli, R. Alonso, R. Bressan-Smith and R. Bottini, UV-B impairs growth and gas exchange in grapevines grown in high altitude, Physiol. Plant., 2013, 149, 127–140 CrossRef CAS PubMed.
- J. J. Wargent, B. C. W. Nelson, T. K. McGhie and P. W. Barnes, Acclimation to UV-B radiation and visible light in Lactuca sativa involves up-regulation of photosynthetic performance and orchestration of metabolome-wide responses, Plant, Cell Environ., 2014 DOI:10.1111/pce.12392.
- R. H. Douglas and G. Jeffery, The spectral transmission of ocular media suggests ultraviolet sensitivity is widespread among mammals, Proc. R. Soc. London, Ser. B, 2014, 281, 20132995 CrossRef CAS PubMed.
- H. Tsujita and C. E. Plummer, Bovine ocular squamous cell carcinoma, Vet. Clin. North Am. Food Anim. Pract., 2010, 26, 511–529 CrossRef PubMed.
- P. Sampath-Wiley and L. S. Jahnke, A new filter that accurately mimics the solar UV-B spectrum using standard UV lamps: the photochemical properties, stabilization and use of the urate anion liquid filter, Plant, Cell Environ., 2011, 34, 261–269 CrossRef CAS PubMed.
-
P. J. Aphalo, A. Albert, L. O. Björn, A. McLeod, T. M. Robson and E. Rosenqvist, Beyond the visible: A handbook of best practice in plant UV photobiology, in COST Action FA0906 UV4growth, University of Helsinki, Department of Biosciences, Division of Plant Biology, Helsinki, Finland, 2012, p. 176, https://helda.helsinki.fi/handle/10138/37558 Search PubMed.
- K. Baczynska, J. B. O'Hagan, A. J. Pearson and P. Eriksen, Temperature Correction of UV Spectral Solar Measurements for ICEPURE Project, Photochem. Photobiol., 2011, 87, 1464–1467 CrossRef CAS PubMed.
-
G. Seckmeyer, A. Bais, G. Bernhard, M. Blumthaler, S. Drüke, P. Kiedron, K. Lantz, R. McKenzie and S. Riechelmann, Instruments to Measure Solar Ultraviolet Radiation WMO/TD-No. 1538, Part 4: Array Spectroradiometers (vol. No. 191), World Meteorological Organization, Geneva, 2010 Search PubMed.
- R. M. Lucas, M. Norval, R. E. Neale, A. R. Young, F. R. de Gruijl, Y. Takizawa and J. C. van der Leun, The consequences for human health of stratospheric ozone depletion in association with other environmental factors, Photochem. Photobiol. Sci., 2015, 14 Search PubMed , this issue.
- A. V. Parisi, P. Schouten, N. J. Downs and J. Turner, Solar UV exposures measured simultaneously to all arbitrarily oriented leaves on a plant, J. Photochem. Photobiol., B, 2010, 99, 87–92 CrossRef CAS PubMed.
- A. V. Parisi, M. G. Kimlin, J. C. F. Wong and M. Wilson, Solar ultraviolet exposures at ground level in tree shade during summer in south east Queensland, Int. J. Environ. Health Res., 2001, 11, 117–127 CrossRef CAS PubMed.
- G. Seckmeyer, M. Klingebiel, S. Riechelmann, I. Lohse, R. L. McKenzie, J. Ben Liley, M. W. Allen, A.-M. Siani and G. R. Casale, A critical assessment of two types of personal UV dosimeters, Photochem. Photobiol., 2012, 88, 215–222 CrossRef CAS PubMed.
- J. Turner, A. V. Parisi and D. J. Turnbull, Dosimeter for the measurement of plant damaging solar UV exposures, Agric. For. Meteorol., 2009, 149, 1301–1306 CrossRef PubMed.
- M. M. Caldwell, Solar UV irradiation and the growth and development of higher plants, Photophysiology, 1971, 6, 131–177 CAS.
-
A. V. Parisi, P. Schouten and D. J. Turnbull, UV dosimeter based on Polyphenylene Oxide for the measurement of UV exposures to plants and humans over extended periods, in NIWA 2010 UV Workshop: UV radiation and its effects-an update 2010, Queenstown, New Zealand, 2010, pp. 25–26 Search PubMed.
|
This journal is © The Royal Society of Chemistry and Owner Societies 2015 |
Click here to see how this site uses Cookies. View our privacy policy here.