DOI:
10.1039/C4NJ01293C
(Focus)
New J. Chem., 2015,
39, 31-37
O-Arylation with nitroarenes: metal-catalyzed and metal-free methodologies
Received
(in Montpellier, France)
4th August 2014
, Accepted 22nd September 2014
First published on 23rd September 2014
Abstract
In this article, we focus on the introduction of nitroarene as an alternative electrophile for Caryl–O cross-coupling chemistry. In polar aprotic solvents and in the presence of a base at elevated temperature nitroarene undergoes cross-coupling with arylboronic acids or phenols under metal or metal-free reaction conditions. Compared to the conventional aryl halides, nitroarene provides highly attractive and environmentally friendly options for the synthesis of diaryl ether derivatives.
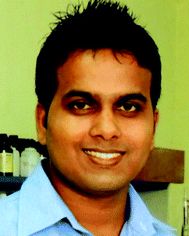
Manoj Mondal
| Manoj Mondal was born in Arunachal Pradesh, India, in 1986. He received his BSc from Digboi College (2007) and his MSc in organic chemistry from Dibrugarh University (2010). He is currently a PhD scholar in the group of Dr Utpal Bora, where he works on the development of transition-metal based catalytic systems and their applications in carbon–carbon and carbon–oxygen bond formation reactions. |
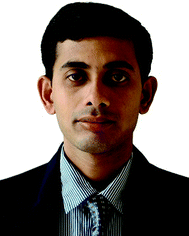
Saitanya K. Bharadwaj
| Saitanya K. Bharadwaj received his MSc from Gauhati University, Assam, in 2003 and PhD from Indian Institute of Technology, Guwahati, India, in 2009. After postdoctoral study at the University of Mississippi, USA, and Technical University of Munich, Germany, he joined Pragjyotish College as Assistant Professor. He was a recipient of the Eli Lilly and Company Asia outstanding Thesis award in 2010. His research interests include organometallic and green chemistry. |
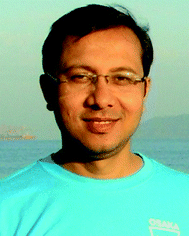
Utpal Bora
| Dr Utpal Bora received his PhD degree in 2005 under the guidance of Dr R. C. Boruah at CSIR-NEIST-Jorhat. He was awarded the JSPS postdoctoral fellowship in 2005 to work under the supervision of Professor Hironao Sajiki at Gifu Pharmaceutical University, Gifu, Japan. After completion of the JSPS postdoctoral tenure, he joined Syngene International Limited, Bangalore, and then moved to the Department of Chemistry, Dibrugarh University, Dibrugarh, as Assistant Professor in 2008. Recently he joined the Department of Chemical Sciences, Tezpur University, Tezpur, as Assistant Professor. His research interests include catalysis, new synthetic strategies, and green chemistry. |
1. Introduction
Transition metal-catalyzed cross-coupling reaction has become a significant methodology for the formation of carbon–heteroatoms and carbon–carbon bonds via the connection of electrophilic and nucleophilic fragments.1 In this domain, the development of Ullmann chemistry for the synthesis of diaryl ether has received extensive attention. Conventionally, when the term “O-arylation chemistry” is mentioned, the prime consideration is the use of aryl halides as electrophiles in the presence of stoichiometric or catalytic amounts of either the copper,2 the palladium3 or the iron4 based catalyst (Scheme 1, eqn (1)). However, aryl halides are generally environmental pollutants, and the by-product generated from their coupling often hampers the isolation and purification processes of the desired products. Additionally, irrespective of their reactivity (Cl ≪ Br < I), their availability (I ≪ Br < Cl) and cost limit widespread applications. Consequently, numerous novel electron-rich ligands and related metal complexes were developed as catalysts which have led to the successful coupling of even less reactive though abundant and inexpensive aryl chlorides under mild conditions.5 Although a plethora of electronically and structurally diverse aryl halides are available these days, some of the structurally targeted substrates required for multistep synthesis may not be easily accessible. Moreover, very few methods for O-arylation with activated aryl halides in the absence of metal are available in the literature6 (Scheme 1, eqn (2)). Thus, the exploration of efficient alternative electrophiles is getting considerable attention not only due to academic interest but to provide additional options when a particular application is under consideration.
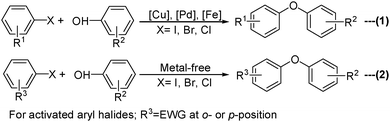 |
| Scheme 1 Conventional metal catalyzed and metal free O-arylation. | |
In addition to the use of aryl halides, nitroarenes are another attractive alternative for the Caryl–O bond formation reaction. Activated nitroarenes contain an ortho- or a para-substituted electron withdrawing group which facilitates the nucleophilic aromatic substitution (SNAr) to produce diaryl or aryl–alkyl ether in the presence of strong nucleophiles. Although it was a significant method developed for the synthesis of ethers, the use of highly reactive nucleophiles competes with many functional groups resulting in limited application. Recent developments in this area have stimulated a number of researchers to study the controlled substitution pattern of activated nitroarenes under metal catalyzed mild reaction conditions.
Apart from being a significant precursor of a variety of substituents in the organic system, the nitro group, which is not usually lost in aliphatic systems, is a particularly good leaving group in SNAr reactions when activated by an ortho- or a para-substituted electron-withdrawing group. The extent of activation is so high, as examined in many studies, that the rate of displacement of an activated nitro group increases relatively higher than that of the chlorine or the bromine group.7a The presence of an ortho- or a para-substituted electron-withdrawing group increases the polarity of the carbon–nitrogen bond, and acts as a highly activating group in SNAr reactions by stabilizing the reaction intermediates through resonance. This disparity facilitates the nucleophilic ipso-substitution yielding synthetically important structural motifs e.g., diaryl or aryl–alkyl ethers (and thioethers).7 Thus, activated nitroarenes like nitro-(pentafluorosulfanyl)benzenes,7c nitro-pyridine-4-carboxylate8a,b and 2-cyano-3-nitroimidazo[1,2-a]pyridine8c have been used in various SNAr reactions (Scheme 2). Despite the usefulness of these methodologies,9 the harsh reaction conditions and high sensitivity of the nucleophile towards the adjacent functional group generate unwanted side products thereby limiting extensive applications. This drawback has made nitroarene less familiar in the cross-coupling domain until the development of transition metal based catalytic systems.
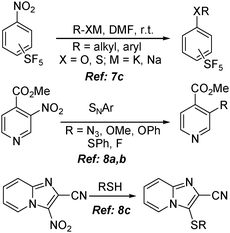 |
| Scheme 2 SNAr reactions of some activated nitroarenes. | |
Wu and co-workers serendipitously discovered that 4-phenoxybenzaldehyde 3a was formed as a secondary product during the palladium- or copper-catalyzed synthesis of (4-nitrophenyl)(phenyl)methanone 3b and (4-nitrophenyl)(phenyl)-methanol 3c from the coupling of phenylboronic acid 1 with 4-nitrobenzaldehyde 2 (Scheme 3).10a
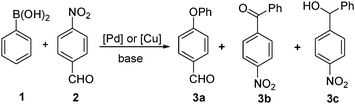 |
| Scheme 3 Pd or Cu catalyzed C–O cross-coupling of nitroarenes. | |
This finding has opened up the door for the introduction of nitroarene reagents as alternative electrophiles for Caryl–O cross-coupling chemistry. Encouraged by this observation, Wu and co-workers have developed a rhodium(I)-based catalytic protocol for the synthesis of diaryl ethers using activated nitroarenes and arylboronic acid.10a Thereafter, Wu,10b,c Chang11 and Phan12 have developed various copper- and palladium-based catalytic protocols for the synthesis of diaryl ethers using activated nitroarene and arylboronic acid or phenol. These novel approaches, reported recently, have gained significant popularity as they provide excellent yields and utilize easily available substrates. In addition, the reaction conditions tolerate all fluoro, chloro and bromo moieties in arylboronic acids (and in nitroarene13), which are commonly used in various cross-coupling reactions, and thus have many further synthetic utilities. Herein, we focus on the recent developments made in the Ullmann cross-coupling of activated nitroarenes with O-nucleophiles, such as arylboronic acid and phenol. These reactions are preferentially metal catalyzed; however, under microwave assisted conditions and at high temperature they proceed without metals.13
2.
O-Arylation of arylboronic acids with nitroarenes
2.1 By using a rhodium(I) catalyst
In 2011, Wu and co-workers reported for the first time that the cross-coupling of activated nitroarenes 4a–h and arylboronic acids 5a–k in the presence of the rhodium(I) complex produces unsymmetrical diaryl ethers 6aa–6ha in excellent yields (Scheme 4).10a The reaction is compatible with dipolar aprotic solvents such as DMF, DMSO and NMP, delivering best results in the presence of Cs2CO3. Initial investigation of the model reaction in the presence of a catalytic amount of Pd(OAc)2 and PdCl2 under various reaction conditions provides only a trace amount of the desired product. The choice of ligands and metal complexes also plays a significant role, as a phosphine ligated complex like RhCl(PPh3)3 exhibits excellent catalytic activity (up to 81%) compared to complexes having a higher or a neutral oxidation state. Although nitroarene bearing o- or p-formyl, acetyl, sulpho and cyano functional groups are well tolerated under these reaction conditions, trace or no product was observed with substrates like (4-nitrophenyl)(phenyl)-methanone 4d, methyl 4-nitrobenzoate 4f and m-formyl nitrobenzene 4h. Coupling of nitro-pyridine, which is also activated in nature, was not described.
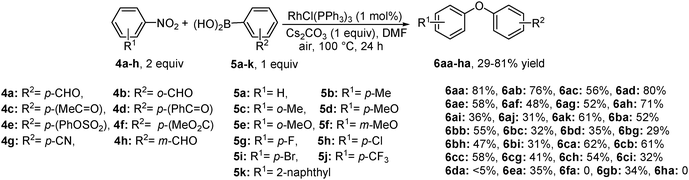 |
| Scheme 4 Rhodium-catalyzed cross-coupling of arylboronic acids with nitroarenes. | |
The chemistry behind this reaction, as it includes an organic electrophile, an organometallic nucleophile and a base, seems to be mechanistically identical to the general transition metal catalyzed cross-coupling reactions. The observations like (i) no reaction under metal-free conditions, and (ii) formation of an aryloxy anion intermediate (by isotope labeling experiment)10a,b suggest that the reaction proceeds via oxidative addition of nitroarene with metal followed by the transmetallation of the organometallic intermediate. Moreover, the advantage of using cesium over other alkali-metal bases had been previously revealed in the contexts of Williamson- and Buchwald-ether synthesis.14 Cesium ions, possessing large cationic charge, low charge density and high electronic polarizability,15 exhibit the lowest degree of solvation compared to analogous alkali metal ions. Hence, in polar aprotic solvents, having a high dielectric constant, better performance is endorsed to the higher solubility and stability of in situ formed cesium aryloxides, which deliver superior nucleophilic reactivity.16 Nevertheless, in contrast to Cs2CO3, K2CO3 displays almost identical basicity although less solubility17 and could be a preferred base when the choice of solvent is DMF or DMSO. However, the use of other strong nucleophilic bases, such as tBuOK, LiF, and CsF in the above reaction, replaces the nitro group in lieu of the aryloxy anion intermediate and lowers the yield of diaryl ether.
2.2 By using a nanoCuO catalyst
Wu et al. reported a great acceleration of C–O cross-coupling catalyzed by copper–oxide nanoparticles and the addition of one equivalent of oxone (potassium peroxymonosulfate, 2KHSO5·KHSO4·K2SO4), an efficient oxidant in DMF (Scheme 5).10b The electronic properties of the substituents on the phenyl ring of arylboronic acids showed a negligible effect on the reaction and no side reactions like homo-coupling and protodeboronation were observed. Moreover, heterocyclic nitroarenes and organoborons, such as 2,4,6-triphenylboroxin and 2-phenyl-4,4,5,5-tetramethyl-1,3,2-dioxaborolane, deliver excellent yields. When controlled experiments were performed under standard conditions (Scheme 6), the reaction between 4-nitrobenzaldehyde and phenol gave isolated yield of diaryl ether (86%), whereas no product was noticed when phenyl boronic acid and 4-hydroxy-benzaldehyde were treated. These provide conclusive evidence that the reaction proceeds via oxidative addition and transmetallation of the aryloxy anion intermediate.
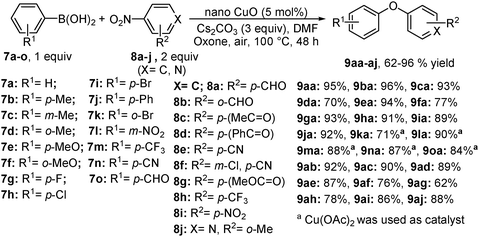 |
| Scheme 5 Copper-catalyzed O-arylation of arylboronic acids with nitroarenes. | |
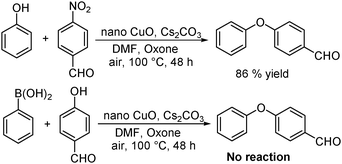 |
| Scheme 6 Control experiments. | |
2.3 By using a 2,2-bipyridine-palladacycle catalyst
Meanwhile, chemists have started to investigate other possible metal catalysts for attaining this catalytic conversion effectively. The first example for palladium-catalyzed denitrated cross-coupling was achieved in co-solvent dioxane–DMSO systems (Scheme 7).11a Chang et al. used this strategy employing a highly electron rich cyclopalladated ferrocenylimine complex A (Scheme 7). They used a combination of both Pd and Fe metals as the catalytic species and Cs2CO3 as a base at 100 °C. Although the method tolerates many heterocyclic boronic acids, ortho-substituted arylboronic acids are sensitive to the steric effect and resulted in much lower yields. Moreover, under present reaction conditions, palladium promotes homo-coupling and protodeboronation of boronic acid (side reactions) hampering isolation and purification processes. Controlled experiments with 4-nitrobenzaldehyde and phenol gave nearly quantitative yield of the desired product.
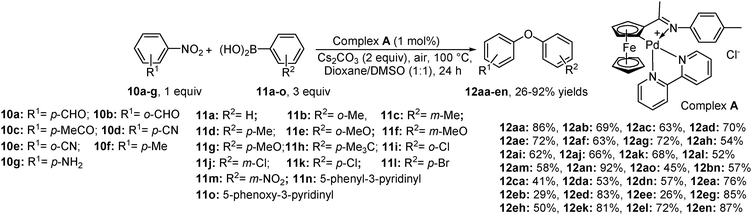 |
| Scheme 7 Pd-catalyzed coupling of nitroarenes with arylboronic acid. | |
3.
O-Arylation of phenols with nitroarenes
3.1 By using a cyclopalladated ferrocenylimine catalyst
In another case, when an equimolar amount of nitroarene and phenol was treated in the presence of complex B (Scheme 8), Cs2CO3 and DMF, excellent yield of diaryl ether was obtained within 2 h (Scheme 8).11b In these above-mentioned reactions, it is observed that the reactivity of the activated nitroarene for the coupling reaction is opposite to that observed for general aromatic nucleophilic substitution SNAr, as it relies on the use of a metal catalyst. The conclusion is that probably the metal is involved in the activation of the nucleophile and may to a certain extent interact with nitroarene. Considering the standard Pd-catalyzed cross-coupling reactions, it can be assumed that these reactions also proceed with the pre-activation of palladacycle (complex A and B) forming Pd(0). Moreover, it appears that the oxidative addition of nitroarene forms arylpalladium species and the presence of base transforms boronic acid or phenol into the aryloxy anion intermediate. The arylpalladium species provides diaryl ether via transmetallation with the aryloxy anion intermediate followed by reductive elimination (Scheme 9a). Whereas, in the case of copper,18 it is believed that actual catalytic Cu(I) is first activated by the nucleophile (to give CuNu or CuOPh) followed by oxidative addition of ArNO2 (via a Cu (III)); finally the product is obtained by reductive elimination (Scheme 9b). Another probable mechanistic pathway may include the involvement of the single electron transfer (SET), as it is known that the copper compounds are capable of electron transfer19 and promote radical nucleophilic substitution reactions in aromatic systems.20
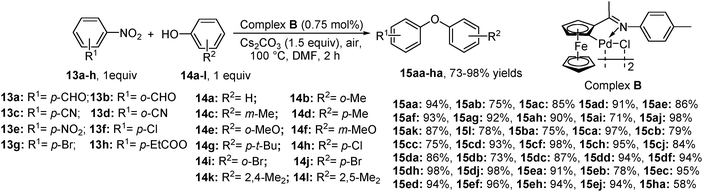 |
| Scheme 8 Palladium-catalyzed O-arylation of nitroarenes with phenols. | |
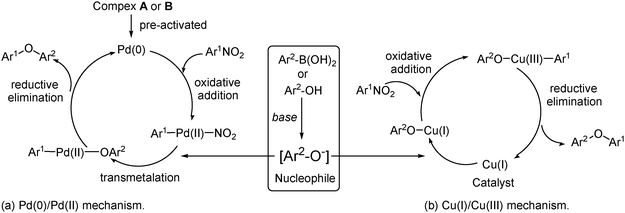 |
| Scheme 9 Probable reaction pathways. | |
3.2 By using a copper acetate catalyst
In another attempt, Wu et al. have used copper catalysts for the C–O denitrated coupling of nitroarene with phenol.10c In the presence of copper acetate under a N2 atmosphere the reaction completes within 4 h and tolerates almost every electronically diverse substrate with negligible steric and electronic intervention (Scheme 10).
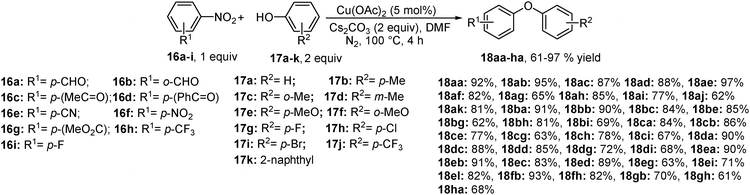 |
| Scheme 10 Synthesis of diaryl ether starting with phenol derivatives. | |
3.3 By using a recyclable Cu2(BDC)2(DABCO) catalyst
Phan et al. have also contributed to the denitrated coupling reaction catalyzed by copper complexes supported on an organic framework.12 They found that a highly crystalline and porous Cu2(BDC)2(DABCO) complex (H2BDC = 1,4-benzenedicarboxylic acid, DABCO = 1,4-diazabicyclo[2.2.2]octane) is very active (>99% conversion) for the coupling of nitroarene with phenol at 100 °C. It was evident from the results of optimization that the reaction relies on high temperature and catalyst concentration, and no conversion took place at 30 °C or in the absence of the Cu-catalyst after 2 h. The main advantages of this methodology were the use of less expensive K2CO3 as a base and the catalyst reusability for six consecutive runs without any noticeable decrease in activity. The substrate scope and reactivity are consistent with above-mentioned methods but the role of the bulky Cu-complex in the reaction was not discussed (Scheme 11). Moreover, under both above-stated copper catalyzed conditions 2 equiv. of phenol is necessary to provide optimal yield.
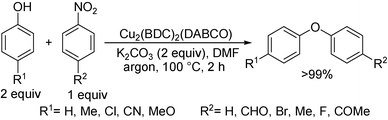 |
| Scheme 11 Recyclable copper based catalyst for coupling of phenol and nitroarene derivatives. | |
4. Microwave assisted metal-free arylation of phenols with nitroarenes
Until now, it has been considered that the formation of Caryl–O bonds between nitroarenes and O-nucleophile reaction depends on the SNAr or metal catalyzed conditions. However, the use of a metal catalyst and activated nitroarene is not always necessary, as recently Shinde et al. have achieved the denitrated cross-coupling of non-activated nitroarenes with phenols under high temperature microwave-irradiation conditions without a metal catalyst.13 Optimum yields of the product were obtained at reflux within 8–11 min (70–92% conversion) in the presence of K2CO3 and DMSO (Scheme 12). A similar report on the arylation of O- and N-nucleophiles with activated haloarenes, with or without added metal, was recently made by the Taillefer group.6c
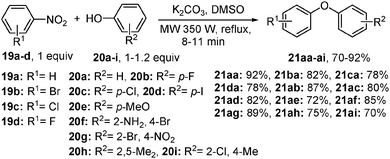 |
| Scheme 12 Microwave-assisted denitrated coupling of nitroarenes. | |
These findings have opened up a new avenue of opportunity in the domain of nitroarene-based Caryl–O bond formation chemistry as, until now, it was believed that a transition metal or a strong nucleophile is essential to break the strong sp2 aryl C–N bond of nitroarene. This metal-free protocol provides:
(i) efficient denitrated coupling with even non-activated nitrobenzene,
(ii) selective nitro substitution; C–X (–Br, –Cl, –F) and C–NH2 bonds showed no reactivity, and
(iii) coupling under reduced reaction times.
Although a microwave provides focused and rapid superheating, it may be too early to consider it as the only driving factor for the reaction and to exclude the previous observations related to metal catalysis. Moreover, one can also speculate on the possible generation of the aryl or the aryloxy radical under high temperature conditions. Clearly, further information like frontier orbital theory and electrochemical measurements of the reacting partners is needed to understand the mechanistic basis of the coupling. Determination of trace metal impurities (using ICP-AES analysis) in the reaction system may show the presence of other possible catalytic species.21,22
5. Conclusions and perspective
Replacement of aryl halides from Ullmann chemistry with nitroarenes will undoubtedly provide a sustainable chemical process for the synthesis of diaryl ethers. Although the catalysis under thermal heating conditions is limited to activated nitroarenes, the microwave assisted method proceeds efficiently with both activated and deactivated substrates. Thus, a proper understanding using Density functional theory (DFT) calculations of the active catalytic intermediate along with electronic charge distribution of coupling partners will be a major step forward to overcome the substrate limitations.
While significant progress has been made, a series of control experiments, such as thermal coupling, radical scavenging experiments (with traps like 2,2,6,6-tetramethylpiperidine N-oxide (TEMPO), CBrCl3, Bu3SnH, galvinoxyl, electron-deficient olefins, diaryl diselenide) and kinetic isotope effects on the reaction components, are still necessary to model the exact pathway of the reaction. Moreover, exploration of the unconventional aqueous system will undoubtedly provide significant outcomes, as the plausible mechanism/isotopic experiments (in the case of metal-based studies) confirm the generation of the aryloxy anion in the presence of ambient water.
Thus, these findings need to be confirmed with further studies performed under different experimental conditions. We expect further progress in this area of research, which will lead to a mild and efficient reaction protocol that will tolerate a wide array of substrates, providing better possibilities for cross-coupling chemistry.
Acknowledgements
We gratefully thank UGC, New Delhi (India), for support No. 41-254/2012 (SR). M.M. thanks UGC, New Delhi, for the UGC-BSR (RFSMS) fellowship.
Notes and references
- For a review on metal catalyzed cross-coupling reaction, see:
(a) K. C. Nicolaou, P. G. Bulger and D. Sarlah, Angew. Chem., Int. Ed., 2005, 44, 4442 CrossRef CAS PubMed;
(b) S. H. Cho, J. Y. Kim, J. Kwak and S. Chang, Chem. Soc. Rev., 2011, 40, 5068 RSC;
(c) G. C. Fortman and S. P. Nolan, Chem. Soc. Rev., 2011, 40, 5151 RSC;
(d) I. P. Beletskaya and A. V. Cheprakov, Organometallics, 2012, 31, 7753 CrossRef CAS.
- For a review on copper catalyzed cross-coupling, see:
(a) J. S. Sawyer, Tetrahedron, 2000, 56, 5045 CrossRef;
(b) S. V. Ley and A. W. Thomas, Angew. Chem., Int. Ed., 2003, 42, 5400 CrossRef CAS PubMed;
(c) A. Correa, O. G. Mancheno and C. Bolm, Chem. Soc. Rev., 2008, 37, 1108 RSC;
(d) F. Monnier and M. Taillefer, Angew. Chem., Int. Ed., 2009, 48, 6954 CrossRef CAS PubMed.
- For examples of palladium-catalyzed synthesis of diaryl ethers, see:
(a) G. Mann, C. Incarvito, A. L. Rheingold and J. F. Hartwig, J. Am. Chem. Soc., 1999, 121, 3224 CrossRef CAS;
(b) Q. Shelby, N. Kataoka, G. Mann and J. Hartwig, J. Am. Chem. Soc., 2000, 122, 10718 CrossRef CAS;
(c) C. H. Burgos, T. E. Barder, X. Huang and S. L. Buchwald, Angew. Chem., Int. Ed., 2006, 45, 4321 CrossRef CAS PubMed;
(d) Bandna, N. R. Guha, A. K. Shil, D. Sharma and P. Das, Tetrahedron Lett., 2012, 53, 5318 CrossRef CAS.
- For examples of iron-catalyzed synthesis of diaryl ethers, see:
(a) N. Xia and M. Taillefer, Chem. – Eur. J., 2008, 14, 6037 CrossRef CAS PubMed;
(b) X. Liu and S. Zhang, Synlett, 2011, 268 CAS;
(c) R. Arundhathi, D. Damodara, P. R. Likhar, M. L. Kantam, P. Saravanan, T. Magdaleno and S. H. Kwon, Adv. Synth. Catal., 2011, 353, 1591 CrossRef CAS;
(d) R. Zhang, J. Liu, S. Wang, J. Niu, C. Xia and W. Sun, ChemCatChem, 2011, 3, 146 CrossRef CAS.
-
(a) G. Evano, N. Blanchard and M. Toumi, Chem. Rev., 2008, 108, 3054 CrossRef CAS PubMed;
(b) M. Carril, R. SanMartin and E. Domınguez, Chem. Soc. Rev., 2008, 37, 639 RSC;
(c) D. A. Alonso and C. Najera, Chem. Soc. Rev., 2010, 39, 2891 RSC;
(d) C. Deraedt and D. Astruc, Acc. Chem. Res., 2014, 47, 494 CrossRef CAS PubMed.
- For SNAr reactions of activated aryl halides, see:
(a) J. S. Sawyer, E. A. Schmittling, J. A. Palkowitz and W. J. Smith, III, J. Org. Chem., 1998, 63, 6338 CrossRef CAS PubMed;
(b) A. B. Naidu, E. A. Jaseer and G. Sekar, J. Org. Chem., 2009, 74, 3675 CrossRef CAS PubMed;
(c) M. P. Drapeau, T. Ollevier and M. Taillefer, Chem. – Eur. J., 2014, 20, 5231 CrossRef PubMed.
-
(a) J. R. Beck, Tetrahedron, 1978, 34, 2057 CrossRef CAS;
(b)
N. Ono, The Nitro Group in Organic Synthesis, Wiley & Sons, New York, 2001, pp. 302–324 CrossRef;
(c) P. Beier, T. Pastyrıkova, N. Vida and G. Iakobson, Org. Lett., 2011, 13, 1466 CrossRef CAS PubMed.
-
(a) J. Holt, F. Tjosaas, J. M. Bakke and A. Fiksdahl, J. Heterocycl. Chem., 2004, 41, 987 CrossRef CAS;
(b) F. Tjosaas and A. Fiksdahl, Molecules, 2006, 11, 130 CrossRef CAS PubMed;
(c) L. Arias, H. Salgado-Zamora, H. Cervantes, E. Campos, A. Reyes and E. C. Taylor, J. Heterocycl. Chem., 2006, 43, 565 CrossRef CAS.
- E. N. Pitsinos, V. P. Vidali and E. A. Couladouros, Eur. J. Org. Chem., 2011, 1207 CrossRef CAS.
-
(a) X. Zheng, J. Ding, J. Chen, W. Gao, M. Liu and H. Wu, Org. Lett., 2011, 13, 1726 CrossRef CAS PubMed and references cited therein;
(b) J. Zhang, J. Chen, M. Liu, X. Zheng, J. Ding and H. Wu, Green Chem., 2012, 14, 912 RSC;
(c) J. Chen, X. Wang, X. Zheng, J. Ding, M. Liu and H. Wu, Tetrahedron, 2012, 68, 8905 CrossRef CAS.
-
(a) D. Peng, A. Yu, H. Wang, Y. Wu and J. Chang, Tetrahedron, 2013, 69, 6884 CrossRef CAS;
(b) H. Wang, A. Yu, A. Cao, J. Chang and Y. Wu, Appl. Organomet. Chem., 2013, 27, 611 CAS.
- N. T. S. Phan, T. T. Nguyen, V. T. Nguyen and K. D. Nguyen, ChemCatChem, 2013, 5, 2374 CrossRef CAS.
- A. P. Sarkate, S. S. Bahekar, V. M. Wadhai, G. N. Ghandge, P. S. Wakte and D. B. Shinde, Synlett, 2013, 1513 CAS.
-
(a) W. H. Kruizinga and R. M. Kellogg, J. Am. Chem. Soc., 1981, 103, 5183 CrossRef CAS;
(b) J.-F. Marcoux, S. Doye and S. L. Buchwald, J. Am. Chem. Soc., 1997, 119, 10539 CrossRef CAS;
(c) J. P. Wolfe and S. L. Buchwald, J. Org. Chem., 2000, 65, 1144 CrossRef CAS PubMed.
- C. Galli, Org. Prep. Proced. Int., 1992, 24, 287 CrossRef.
-
(a) X. Xing, D. Padmanaban, L.-A. Yeh and G. D. Cuny, Tetrahedron, 2002, 58, 7903 CrossRef CAS;
(b) C. L. Liotta, E. E. Grisdale and H. P. Hopkins, Jr., Tetrahedron Lett., 1975, 4205 CrossRef CAS;
(c) F. Lehmann, Synlett, 2004, 2447 CrossRef CAS.
- J. A. Cella and S. W. Bacon, J. Org. Chem., 1984, 49, 1122 CrossRef CAS.
- For recent mechanistic studies on Cu-catalyzed C–O coupling, see:
(a) G. Lefèvre, A. Tlili, M. Taillefer, C. Adamo, I. Ciofini and A. Jutand, Dalton Trans., 2013, 42, 5348 RSC;
(b) C. Sambiagio, S. P. Marsden, A. J. Blacker and P. C. McGowan, Chem. Soc. Rev., 2014, 43, 3525 RSC.
-
(a) F. Bellesia, A. J. Clark, F. Felluga, A. Gennaro, A. A. Isse, F. Roncaglia and F. Ghelfi, Adv. Synth. Catal., 2013, 355, 1649 CrossRef CAS;
(b) W.-M. Liu, Z.-H. Liu, W.-W. Cheong, L.-Y. T. Priscilla, Y. Li and K. Narasaka, Bull. Korean Chem. Soc., 2010, 31, 563 CrossRef CAS;
(c) J. A. Bull, M. G. Hutchings and P. Quayle, Angew. Chem., Int. Ed., 2007, 46, 1869 CrossRef CAS PubMed;
(d) S.-F. Wang, C.-P. Chuang, J.-H. Lee and S.-T. Liu, Tetrahedron, 1999, 55, 2273 CrossRef CAS.
- For a review on the mechanism of the modified Ullmann reactions, see: E. Sperotto, G. P. M. van Klink, G. van Koten and J. G. de Vries, Dalton Trans., 2010, 39, 10338 RSC.
- For cross-coupling catalyzed by trace metal contaminants, see:
(a) P.-F. Larsson, A. Correa, M. Carril, P.-O. Norrby and C. Bolm, Angew. Chem., Int. Ed., 2009, 48, 5691 CrossRef CAS PubMed and references cited therein;
(b) S. L. Buchwald and C. Bolm, Angew. Chem., Int. Ed., 2009, 48, 5586 CrossRef CAS PubMed;
(c) R. A. D. Arancon, C. S. K. Lin, C. Vargas and R. Luque, Org. Biomol. Chem., 2014, 12, 10 RSC.
- Leadbeater discovered that the ultra-low level of palladium (20-50 ppb) contained in the commercially available sodium carbonate efficiently catalyzes the well-known Suzuki reaction under high temperature-microwave irradiation conditions in water, see:
(a) N. E. Leadbeater and M. Marco, Angew. Chem., Int. Ed., 2003, 42, 1407 CrossRef CAS PubMed;
(b) R. K. Arvela, N. E. Leadbeater, M. S. Sangi, V. A. Williams, P. Granados and R. D. Singer, J. Org. Chem., 2005, 70, 161 CrossRef CAS PubMed . For recent discussion and review on the role of trace metal impurities in the reaction, see: ;
(c) N. E. Leadbeater, Nat. Chem., 2010, 2, 1007 CrossRef CAS PubMed;
(d) I. Thomé, A. Nijs and C. Bolm, Chem. Soc. Rev., 2012, 41, 979 RSC.
|
This journal is © The Royal Society of Chemistry and the Centre National de la Recherche Scientifique 2015 |