DOI:
10.1039/C5LC00741K
(Paper)
Lab Chip, 2015,
15, 4322-4330
One-step in-mould modification of PDMS surfaces and its application in the fabrication of self-driven microfluidic channels†
Received
27th June 2015
, Accepted 8th September 2015
First published on 9th September 2015
Abstract
Poly(dimethylsiloxane) (PDMS) has become the material of choice for fabricating microfluidic channels for lab-on-a-chip applications. Key challenges that limit the use of PDMS in microfluidic applications are its hydrophobic nature, and the difficulty in obtaining stable surface modifications. Although a number of approaches exist to render PDMS hydrophilic, they suffer from reversion to hydrophobicity and, frequently, surface cracking or roughening. In this study, we describe a one-step in-mould method for the chemical modification of PDMS surfaces, and its use to assess the ability of different surfactants to render PDMS surfaces hydrophilic. Thin films of ionic and non-ionic surfactants were patterned into an array format, transferred onto silicone pre-polymer, and subsequently immobilized onto the PDMS surface during vulcanization. The hydrophilicity of the resulting surfaces was assessed by contact angle measurements. The wettability was observed to be dependent on the chemical structure of the surfactants, their concentration and interactions with PDMS. The morphology of modified PDMS surfaces and their change after wetting and drying cycles were visualized using atomic force microscopy. Our results show that while all surfactants tested can render PDMS surfaces hydrophilic through the in-mould modification, only those modified with PEG–PDMS–PEG copolymer surfactants were stable over wetting/dying cycles and heat treatments. Finally, the in-mould functionalization approach was used to fabricate self-driven microfluidic devices that exhibited steady flow rates, which could be tuned by the device geometry. It is anticipated that the in-mould method can be applied to a range of surface modifications for applications in analytical separations, biosensing, cell isolation and small molecule discovery.
Introduction
Advances in the microfabrication of microfluidic and biochip devices have made PDMS the material of choice for biomedical, analytical and biotechnological applications. The inherent properties of PDMS such as optical transparency, biocompatibility, gas permeability, widespread availability, low cost, and ease of fabrication have made it particularly attractive in lab-on-a-chip applications for biomedical diagnostics.1–4 The biggest drawback in the use of PDMS for these applications is its hydrophobic nature, which prevents aqueous solutions from filling micron and nanometer-sized channels through capillary action and promotes device fouling through the non-specific adsorption of proteins and small molecules.5 Significant efforts have been made to introduce reactive chemical functionalities onto PDMS surfaces to render them hydrophilic. Such strategies include the use of high-energy treatments in the form of ion plasma,6,7 UV-O38,9 and corona discharge10 to introduce hydroxyl groups on the surface of PDMS, coating of PDMS surfaces with polar functionalities using chemical vapour deposition,11 silanization,12,13 phospholipid bilayer14,15 or polyelectrolyte multilayer16,17 modifications, and more recently grafting of hydrophilic polymers to or from the surface of PDMS via ultraviolet/atom transfer radical polymerization,18–21 hydrosilylation22 and click chemistry.23 While these interventions have proved successful, they can be limited in applications, have limited chemical stability, are hard to direct to specific sites within microfluidic channels, or involve multiple steps that make the process cumbersome or difficult to achieve within microdevices. More recently, a strategy was reported to make permanently wettable silicone elastomers, but the chemical steps necessary preclude its utilization in microfluidic channels.24 Additional issues reported with some of these modifications include surface cracking and increased roughness, increased opacity, loss of elasticity and reversion to hydrophobicity over time.25,26 These issues limit the usefulness of modified PDMS surfaces and can prevent the subsequent immobilization of biomolecules or cause issues of biocompatibility. To address some of the challenges and provide a simple solution to stable PDMS surface modifications, we have turned to silicone-based molecules as direct functionalization agents that preserve the inherent attributes of silicone elastomers.
In this manuscript, we present a one-step in-mould PDMS functionalization approach, where patterned moulds define the surface sites to be modified and the surface functionalization occurs during the PDMS vulcanization step. Using this method, arrays were prepared where ionic and non-ionic surfactants were applied to the elastomer surface during the curing process, leading to the spatial tethering of uncharged and charged alkyl/polymer chains on PDMS. This method allowed us to directly compare small molecule and block co-polymer surfactant modifications of PDMS surfaces. We investigated the effect of surfactant chemical structure, surfactant–PDMS interaction, drop-casting concentration, and morphology of the modified surface on the wetting properties of the modified surfaces. Through these experiments, we have identified treatments that render the PDMS surface hydrophilic even after repeated wetting/drying cycles and thermal treatment, and further show that the one-step in-mould surface modification approach can be used to produce hydrophilic microfluidic channels that can be filled through capillary action. We anticipate that similar surface modification strategies can be used to spatially pattern channels with functional groups for lab-on-a-chip devices used in analytical separations, biosensing, cell targeting and isolation, or small molecule discovery.
Experimental section
Materials
Seven ionic and non-ionic surfactants were used to modify PDMS surfaces: sodium dodecyl sulphate (SDS), cetyl trimethylammonium bromide (CTAB) and Tween 20 were from Sigma-Aldrich (Oakville, ON), Silsurf A008-UP was from Siltech Corp (Toronto, ON), poly(ethyleneglycol)-silicone-poly(ethyleneglycol) (PEG-PDMS-PEG) non-ionic triblock copolymers with alkyl (o-Wet) and siloxane terminal functionalities (n-Wet and a-Wet, where a-Wet has a more highly branched siloxane) were provided by EnRoute Interfaces, Inc. (Hamilton, ON).
Fabrication of surface functionalized PDMS arrays
In this study, the fabrication approach used soft lithography with masks made by xurography. Self-adhesive Teflon masks (Bytac® surface protection laminate, Sigma-Aldrich) defining 5 mm diameter, 220 μm depth sample wells were designed by computer aided design (CAD) software and patterned using a blade cutter (Graphtec ROBOPro CE5000, Irvine, CA). The Teflon masks were lifted off and adhered to clean polystyrene dishes. Thin surfactant films were formed inside the sample wells by drop casting either 8 μl of ionic (2, 5 and 10% wt/v in 7
:
3 IPA/water) or non-ionic (2, 5, and 10% wt/v in IPA) surfactant solutions into each well and allowing them to dry overnight. Silicone pre-polymer (10
:
1 wt% elastomer
:
hardener, Sylgard 184, Dow corning) was applied with a syringe around the Teflon mask and allowed to flow over the sample wells. This was left to cure at room temperature (RT) for 48 h on a level surface. The modified PDMS arrays were gently peeled off the Teflon mask and further cured at 60 °C for 4 h. The arrays were then rinsed with isopropyl alcohol (IPA) to remove excess surfactant and returned to the oven to dry at 60 °C for an additional 4 h. Finally, the modified surfaces were soaked in water for 20 h at RT and dried under a nitrogen stream.
Characterization of functionalized PDMS arrays
The wettability of the functionalized PDMS surfaces was assessed through static contact angle measured by the sessile drop method using an OCA 20 Future Digital Scientific system (Garden City, NY). Briefly, 1 μl of 18.2 MΩ cm−1 water (A10-Merck-Millipore system, Darmstadt, Germany) was dropped onto the modified PDMS surface and digital images were acquired. The average of measurements taken from 40 replicate surfaces is reported as the mean contact angle for the functionalized PDMS.
The morphology of the functionalized surfaces was visualized through Atomic Force Microscopy (AFM) using an Asylum Research MFP-3D Classic™ Scanning Probe Microscope (Santa Barbara, CA). The images were taken in tapping mode with aluminium reflex coated silicon cantilevers (Veeco AC240TS-R3, Olympus) with nominal spring constant of 2 N m−1 and resonant frequency of 70 kHz.
Fabrication of surface-modified microfluidic devices
Self-adhesive vinyl film (80 μm thickness, FDC-4300, FDC graphic films, South Bend, IN) was used in the fabrication of microfluidic channel moulds. Vinyl films were patterned into both linear and serpentine channel templates (0.1, 0.5, 1 mm width × 50 mm length) using a blade cutter (Graphtec ROBOPro CE5000). These templates were lifted off with the aid of transfer tape and adhered to a clean polystyrene dish. Vinyl masks 0.1 mm wider than the microfluidic templates were also patterned and adhered around each template, leaving a space between the mask and the template. Each of the vinyl templates was coated with a thin film of a-Wet by drop casting 30 μl of 10% solution (wt/v in IPA) over the template and allowing it to dry overnight at RT. The vinyl masks were then carefully removed. 1.2 cm Masterflex® Silicone tubing (1/16′′ × 0.189′′, Cole-Parmer Canada Inc.) was placed over each reservoir on the vinyl template to define inlet and outlet ports. Silicone prepolymer (10
:
1 wt% elastomer base
:
curing agent, Sylgard 184) was applied with a syringe around the coated vinyl and allowed to flow slowly over the template. After curing at RT for 24 h, the surface-modified microfluidic channel was cut using a scalpel, rinsed with IPA to remove excess surfactant, dried under nitrogen and adhered to a 75 mm × 25 mm microscope slide by applying even pressure.
Results and discussion
In-mould modification of PDMS surfaces
A one-step in-mould modification process for the controlled spatial tethering of surfactant molecules at the PDMS surface was developed as a simple and cost effective alternative to conventional surface grafting and modification approaches (Fig. 1). The key concept behind the one-step modification is that exposure of the pre-polymer to surfactants during the vulcanization process can entrap the surfactant molecules through adsorption, hydrophobic binding, and/or entanglement at the PDMS interface and confer to it the surfactant's functionality (Fig. 1, inset A). Furthermore, we hypothesized that by choosing the right chemical structure of the surfactant molecules (i.e., copolymers containing a PDMS block), they would be irreversibly entrapped by forming an interpenetrating network with the crosslinking polymer, yielding stable functional PDMS surfaces (Fig. 1, inset B). The one-step in-mould functionalization process was used to produce arrays of PDMS surfaces modified with a range of surfactants. These arrays allowed us to compare the ability of the immobilized surfactants to convey a stable hydrophilic character to the PDMS surface.
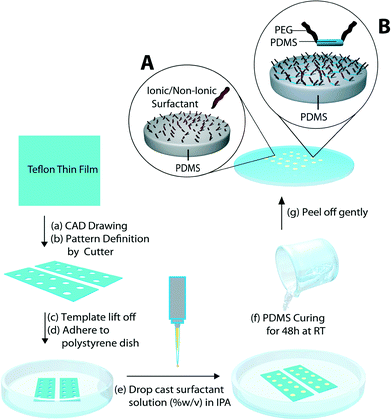 |
| Fig. 1 Hydrophilic PDMS arrays: a schematic of the fabrication technique for immobilizing surfactant molecules onto PDMS surface. Insets: Schematics of proposed molecular conformation of adsorbed and/trapped surfactant molecules at the PDMS–air interface. | |
Seven sets of ionic and non-ionic surfactants were spatially patterned onto PDMS as illustrated in Fig. 1. First, xurography was used to pattern individual wells into Teflon adhesive sheets. Then, the surfactant solutions were drop cast into each well and allowed to dry into thin films, after which the PDMS pre-polymer solution was applied and cured. The dry thin films made from non-ionic surfactant solutions appeared optically clear (Fig. S1†). Such morphology was expected, as the non-ionic surfactants have been reported to form ordered reverse micelle multilayers upon drying.27 In particular, non-ionic PEG-PDMS-PEG block co-polymer surfactants were expected to form multilayers, where the PEG moiety would reside at the core and the hydrophobic PDMS would be exposed at the air interface, which would allow them to become entrapped in the interpenetrating network of the cured PDMS material. On the other hand, films deposited from ionic surfactant solutions were opaque (Fig. S1†), since the ionic surfactants crystallized as the solvent was removed. The observed thin film morphology was transferred to the PDMS surface during the curing/moulding process and was visible under the optical microscope after the PDMS surfaces were peeled off from the Teflon moulds (Fig. S2†). Extensive rinsing of the modified surfaces with water produced optically clear areas for all surfactant treatments (Fig. S3†). This indicated that immersion in water or rinsing could change the surface properties and/or topography.
Wettability of modified PDMS surfaces
The efficiency and reproducibility of the one-step modification method in producing stable hydrophilic surfaces was assessed by measuring sessile water droplet contact angles (WCA) on the surfactant-modified surfaces and comparing them against unmodified PDMS. It was observed that all surfactant films were stable and able to withstand the drag force of the uncured prepolymer mixture during the PDMS casting step. After peeling from the Teflon template, the one-step modification produced surfaces that exhibited uniform droplet spreading and wetting behaviour over the modified areas. This suggested the homogeneous transfer as well as full coverage of surfactant molecules within each of the circular modified areas.
Fig. 2 depicts the wettability of PDMS surfaces functionalized by each of the surfactant molecules. All seven surfactants tested led to hydrophilic surfaces after the one-step in-mould modification, with contact angles significantly below that of unmodified PDMS (109°). Surfaces that were functionalized by trapping/adsorbing ionic surfactants SDS and CTAB yielded WCA of 57° and 38° respectively. Tween 20 (a non-ionic PEG(20)sorbitan monolaurate surfactant) and Silsurf A008-UP (a non-ionic, low molecular weight ethoxylated PDMS surfactant commercially available as a silicone super-wetter) also demonstrated a significant drop in the hydrophobicity of the treated PDMS surfaces, as they were hydrophilic with WCA of 39° and 20°, respectively. Finally, surfaces functionalized with PEG-PDMS-PEG triblock co-polymers o-Wet, n-Wet and a-Wet were all hydrophilic with WCA 43°, 47° and 22°, respectively. The contact angle measurements show that all the surfactant-functionalized surfaces were rendered hydrophilic through the one-step in-mould PDMS functionalization process.
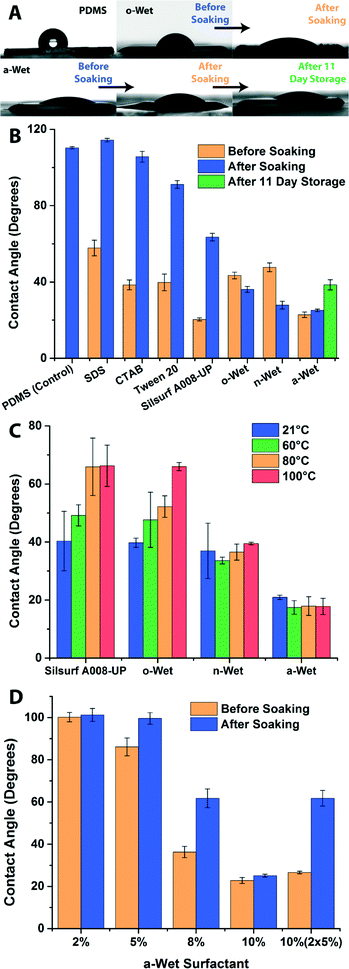 |
| Fig. 2 Water contact angle (WCA) measurements of surfactant functionalized PDMS surfaces. A) Representative images of WCA measurements performed on PDMS surfaces as prepared before soaking, after soaking and after 11 days of storage in air. B) Quantification of WCA measurements for all surfactant-modified PDMS surfaces. C) Quantification of WCA measurements for surfactant modified PDMS surfaces at different temperatures. D) WCA measurements of PDMS surfaces functionalized with thin films produced by a-Wet solutions cast at different concentrations. Error bars represent the standard error (n > 7). | |
The stability of the hydrophilicity of modified surfaces and the strength of PDMS–surfactant interactions were tested by soaking the treated PDMS surfaces in 18.2 MΩ water for 20 hours. Fig. 2B shows that in most cases, soaking the treated surfaces led to a drastic change in the contact angle. As expected, there were weak interactions between PDMS and the ionic surfactants, which were solvated as the modified surfaces were soaked in water. Thus, soaking the surfaces left behind bare PDMS surfaces that were hydrophobic in nature (WCA of 114° and 106° for SDS and CTAB, respectively). Similar scenarios were observed after soaking Tween 20 and Silsurf A008-UP modified surfaces, but with milder effects (they exhibited WCA of 91° and 63°). The incomplete reversion toward hydrophobicity can be explained as the result of an increased interaction of non-ionic surfactants with the hydrophobic PDMS surface. Nevertheless, prolonged exposure to an aqueous environment or fluid flow has been observed to lead to full reversion of surfaces treated with Tween 20 or the Silsurf A008-UP silicone super-wetter (data not shown). On the other hand, surfaces treated with the three PEG-PDMS-PEG block copolymers o-Wet, n-Wet and a-Wet remained hydrophilic after soaking (Fig. 2A and B). This suggests that the triblock copolymers were effectively entrapped in the silicone network at the PDMS interface during the polymerization step. Furthermore, it was observed that after soaking, o-Wet and n-Wet treated surfaces exhibited a drop in contact angle values to 36° and 27°, respectively. This observation suggests a rearrangement of the surfactant molecules at the surface mediated by hydration. The third triblock copolymer a-Wet showed highly stable hydrophilic behaviour, as it only exhibited a slight increase in contact angle from 22° to 25° after soaking and remained hydrophilic (WCA of 40°) even after 11 day storage in dry conditions on the bench top.
The impact of temperature on the hydrophilicity of triblock copolymer and Silsurf-modified PDMS surfaces was also investigated. The treated surfaces were soaked for two hours, dried with nitrogen and incubated at different temperatures for one hour prior to measuring their WCA. Fig. 2C shows that surfactants Silsurf A008-UP and o-Wet exhibit an increase in contact angle as the temperature is increased, indicating a loss of the exposed surfactant or a collapse of the hydrophilic moieties in the surfactant as the modified surface is dried at higher temperatures. On the other hand, triblock copolymer surfactants n-Wet and a-Wet showed stable wettability under the temperature conditions tested. It must also be noted that at temperatures higher than 21 °C the o-Wet treated surfaces required a couple of minutes incubation time with the water droplet for the contact angle to stabilize to the values reported in Fig. 2C, indicating that rehydration of the dry surfactant on the surface is important for it to display its hydrophilic properties. These experimental results demonstrate that all surfactants tested can be used in the one-step in-mould modification protocol to produce hydrophilic PDMS surfaces, but that only the triblock copolymers that are entrapped at the interface show stability against wetting/drying cycles. The retention of hydrophilicity after washing/drying cycles, prolonged storage and temperature treatment shows that a-Wet modified surfaces do not readily undergo reversion to hydrophobicity, as many other PDMS surface modifications do, making it an excellent candidate for the permanent modification of silicone surfaces.
Effect of surfactant concentration on wettability
Since the surfactant a-Wet showed promising results for the production of stable hydrophilic PDMS surfaces, we further studied how the concentration of the surfactant impacted the wettability. To this end, a series of a-Wet solutions with 2–10% concentration were used and the WCA of the modified PDMS surfaces was assessed (Fig. 2D). It was observed that films produced with concentrations >2% readily increased the wettability of PDMS, with the most hydrophilic surfaces achieved by using 10% surfactant films. This suggests that a minimum amount of surfactant is needed to completely cover the 5 mm diameter sample wells cut into the mask used in the modification process. In addition, it was observed that some treatments (5 & 8%) exhibited significant increases in WCA after soaking. This can be attributed to insufficient surfactant immobilized in the well, which results in a fraction of the surfactant molecules that are not trapped within the interpenetrating network of PDMS, and can thus be washed away in the soaking step leading to increased WCA values. Nevertheless, it is important to note that surface coverage and total surfactant mass are not the only requirements for a successful and stable surface modification. This was demonstrated by casting a 5% solution twice, which resulted in a film with the same mass of surfactant as one produced from a 10% solution, and using the resulting film in the in-mould modification (Fig. 2D). While the hydrophilicity before soaking resembled that of the 10% modification (23°), the contact angle after soaking underwent a drastic increase to ~60°. This behaviour is attributed to the layer-by-layer deposition of the thin film, where the micellar arrangement of the first surfactant film was disrupted by the second surfactant application. Thus, to obtain a stable hydrophilic surface it was important to optimize the concentration of the surfactant solution used in the one-step in mould modification process.
Surface morphology of functionalized PDMS surfaces
To gain a better understanding of the surface chemistry of functionalized PDMS, AFM imaging was done on the surfactant-modified surfaces before and after soaking in water. This probed the dependency of the morphology of the modified surface on the chemical structure of the surfactant and its hydration state. All surfactant-modified surfaces were rougher than unmodified PDMS and exhibited changes in surface roughness before and after soaking (representative images shown in Fig. 3). SDS and CTAB-modified PDMS showed roughened surfaces consistent with crystalline aggregates before soaking, which dissolved after soaking leaving behind highly structured, hydrophobic PDMS surfaces. Tween 20 formed a highly uniform film on the PDMS surface and exhibited the lowest surface roughness of all surfactants before soaking. After soaking, this surface remained relatively smooth with only a few depressions forming. This observation, coupled with the WCA measurements of Tween 20-modified surfaces, suggest that this surfactant forms uniform ordered layers that can be dissolved during the soaking step, leaving behind a smooth hydrophobic PDMS surface. PDMS modified with the Silsurf super-wetter showed surfaces presenting small dimples with a narrow size distribution before soaking. However, after soaking the morphology was drastically changed, leaving behind structured surfaces with protruding spherical aggregates. The changes in morphology observed for ionic and non-ionic surfactants can be explained by a limited interaction between the curing PDMS and the surfactant molecules, which can be solvated upon exposure to the aqueous medium leaving behind an imprint that resembles the structure of the dried thin film of surfactant (schematic in Fig. 3).
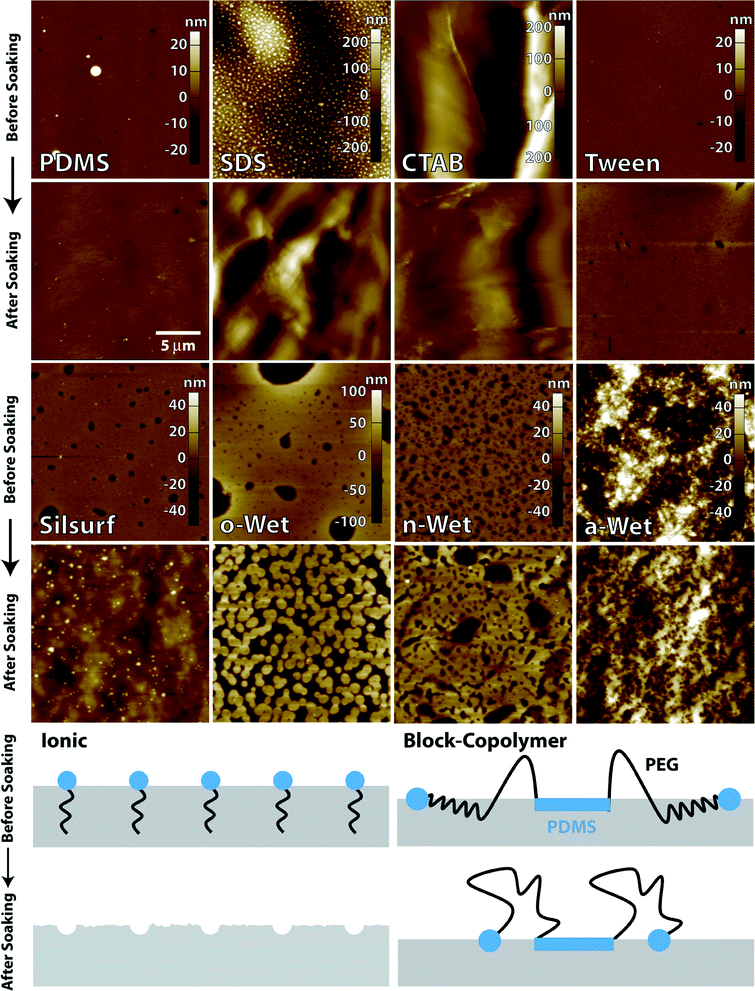 |
| Fig. 3 Representative atomic force microscopy height images of functional PDMS surfaces before and after soaking in aqueous solution. Below: Schematic of proposed molecular conformation of adsorbed and/trapped surfactant and triblock copolymer surfactant molecules at the PDMS–air interface before and after soaking. | |
Surfaces functionalized with PEG-PDMS-PEG block copolymers displayed morphologies that are consistent with polymer entrapment and polymer chain rearrangement upon hydration. Before soaking, o-Wet and n-Wet functionalized surfaces showed relatively smooth surfaces with depressions that ranged in size from large (microns wide, o-Wet) to small (sub-micron wide, n-Wet). These morphologies underwent significant changes upon soaking in water. For o-Wet, the surface showed discrete sub-micron circular domains closely packed or fused together, which suggests that the exposed polymer chains self assembled into hydrophilic–hydrophobic domains. This is consistent with the structure of o-Wet, which has a long alkyl group pendant from the end of each PEG block. For n-Wet the changes were less pronounced, with the small depressions merging into larger ones and the depression size becoming more heterogeneous. On the other hand, the morphology of a-Wet-functionalized PDMS surfaces showed small random features that remained largely unchanged after soaking. This is consistent with the structure of the a-Wet surfactant, where the more highly branched pendant groups at the end of the PEG blocks are not large enough to become segregated upon soaking and remain randomly distributed on the surface, producing a uniformly hydrophilic surface. The topographical images before and after soaking agree with the spreading patterns observed during the WCA measurements. The change in morphology for o-Wet and n-Wet treated surfaces justifies the drop in contact angle upon soaking, as polymer chain rearrangement would swell the PEG blocks and leave them exposed towards the aqueous solution, rendering the surface more hydrophilic. Similarly, the limited change in a-Wet-treated surfaces mirrors the small change in WCA value, supporting the notion that due to the limited branching of siloxane pendants groups, the polymer is randomly distributed on the surface. The morphologies of surfaces functionalized with triblock copolymer surfactants are also similar to those reported for the chemical grafting of PEG onto PDMS.28,29
Based on the wettability and AFM data we propose the following model for the surface functionalization with PEG-PDMS-PEG block copolymers through the one-step in-mould approach. The casting and drying of surfactant solutions within the mould produces ordered multilayers composed of reverse micelles where the PDMS blocks are exposed on the film surface. These blocks then interact with the silicone pre-polymer mixture during the crosslinking step, which breaks up the micelle aggregates and leads to the physical entrapment of the triblock surfactant molecules at the PDMS–air interface. This interaction produces a surface-confined interpenetrating polymer network with a fraction of the PEG blocks that, although in a partially collapsed state, remain exposed at the PDMS surface as the silicone substrate is lifted off the Teflon mask. The exposed PEG blocks confer to the modified PDMS surface the hydrophilicity observed before the soaking step. Upon soaking the modified surfaces in an aqueous solution, the PEG blocks swell in water, can adopt stretched conformations and interact with each other, leading to changes in the surface morphology (schematic in Fig. 3). The extent of the rearrangement is strongly dependent on the nature of the functional groups appended to the end of the PEG blocks. The outcome of the in-mould surface functionalization approach coupled to the chemical structure of the surfactant used leads to hydrophilic PDMS surfaces that are highly stable over time.
Fabrication of self driven microfludic devices
Having demonstrated the successful hydrophilization of PDMS surfaces using in-mould modification with a-Wet surfactant, we pursued the application of a similar approach for the fabrication of hydrophilic PDMS microfluidic devices. The fabrication process, shown in Fig. 4, utilized xurography to create vinyl moulds (red) in the shape of the microfluidic channel. An additional layer was added as a surrounding mask (Fig. 4, white) to confine the surfactant film applied and achieve the functionalization of the sidewalls of the microfluidic channel. This mask was removed after surfactant thin film deposition and prior to casting PDMS. The absence of this surrounding mask during the surfactant application caused the surfactant to flow over the mould and spread preferentially over the polystyrene dish, resulting in a loss in efficiency of the functionalization and decreased hydrophilicity (Video S1†). A total of six microfluidic channel designs were fabricated including 3 linear and 3 serpentine channels with varied geometries (0.1, 0.5 and 1 mm widths, 80 μm height, and 50 mm length). These were rendered hydrophilic using the in-mould modification process. The funtionalized PDMS microfuidic layers were adhered to commercial glass slides that were not cleaned to avoid any capillary flow due to a hydrophilic glass surface. All the fabricated devices were shown to fill through steady capillary action even against gravity (Fig. 5, Videos S2–S4†), while unmodified PDMS control devices failed to fill at all (Video S5†). The advantage of the fabrication technique presented here is that hydrophilic PDMS microfluidic channels that fill uniformly can be fabricated in one step through inexpensive bench-top methods.
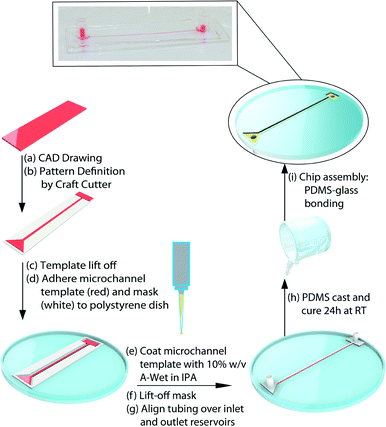 |
| Fig. 4 Self-driven microfluidic device: a schematic of the fabrication technique for immobilizing surfactant molecules onto microfluidic channel surfaces via a one-step in-mould method. | |
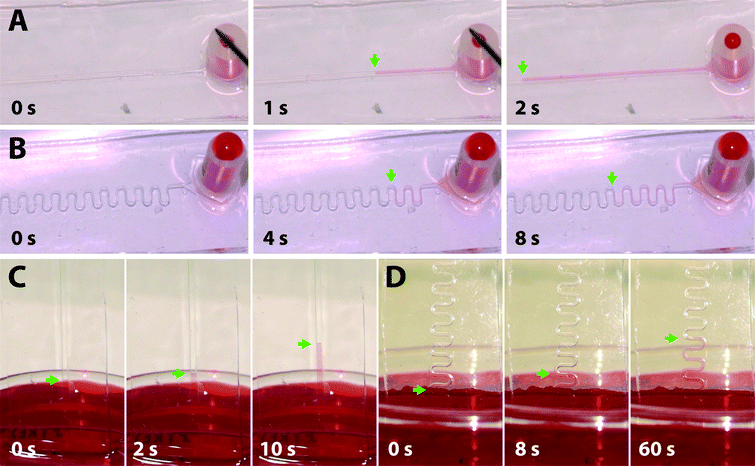 |
| Fig. 5 Capillary-driven filling of hydrophilic PDMS channels with aqueous solutions. Still frames of filling process of A) 0.5 mm wide linear and B) 0.1 mm wide serpentine channels filled horizontally, and 0.5 C) linear and D) serpentine channels filled vertically against gravity. Arrows indicate advancing fluid meniscus position. | |
Given that the flow rate within microfluidic channels is dependent on channel geometry, liquid viscosity, total flow resistance, and pressure differential, we compared the flow rates generated experimentally within a-Wet modified microfluidic channels with those calculated theoretically. The flow rate Q in a capillary system is governed by the expression:
|
 | (1) |
where
η is the viscosity of the liquid, Δ
P can be taken as the capillary pressure
PC, and
RF is the total flow resistance along the flow path. For our system,
PC at the liquid–air interface within the channel can be calculated from equation
30 |
 | (2) |
where
γ is the surface tension of the liquid, while
αt,b,l,r are the contact angles on the top, bottom, left and right walls of the channel. For top and sidewalls of the channel we used the contact angle measured for a-Wet-modified surfaces before soaking, while for the bottom wall we used an experimentally measured contact angle for non-cleaned glass slides of 54°. Furthermore, for the microfluidic channels used in this study the total flow resistance in rectangular channels was approximated by
31 |
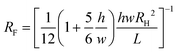 | (3) |
where
h is the height (80 μm),
w is the width (0.1, 0.5 or 1 mm),
L is the length and
RH is the hydraulic radius of the microchannel, which was calculated as the ratio of the cross sectional area by half the wetted perimeter,
|
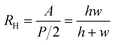 | (4) |
Using these equations, we estimated theoretical flow rates of 270, 1080 and 2530 nl s
−1 for linear functionalized microfluidic channels with widths of 0.1, 0.5 and 1 mm. The experimental flow rates measured were 40, 430 and 450 nL s
−1 for linear microfluidic channel with 0.1, 0.5 and 1 mm widths respectively (
cf.Fig. 5, Video S2
†). The experimental flow rates were expected to be lower than the theoretical ones because they are influenced by reservoir geometry as well as any inhomogeneity in the fabricated device. Similarly, serpentine microfluidic channels had experimental flow rates of 2.4, 36 and 91 nL s
−1 for channel widths of 0.1, 0.5 and 1 mm respectively (
cf.Fig. 5, Video S3
†). These results show that the capillary flow rate can be tuned by changing the dimensions and channel morphology of hydrophilic PDMS microfluidic devices fabricated
via the one-step in-mould modification using surfactant a-Wet. In addition, the observed flow rates are comparable to those reported in the literature for hydrophilic microfluidic devices fabricated by other methods.
30,31 This highlights the ability of the developed method for generating self-driven microfluidic devices with steady flow rates in a simple and cost effective manner.
Conclusions
This work introduces in-mould modification as a simple one-step fabrication method to spatially graft surfactant chains onto unstructured and structured PDMS surfaces. An array format was used to directly compare the hydrophilic nature of surfaces modified with ionic, non-ionic and block co-polymer surfactants. It was shown that although all surfactants tested could produce hydrophilic surfaces, only PEG-PDMS-PEG block co-polymer surfactants were stable against repeated wetting/drying cycles. Furthermore, surfaces modified with the a-Wet surfactant retained their hydrophilicity even after prolonged storage at the bench top. Changes in morphology of the modified surfaces support the notion that the entrapped surfactant chains can rearrange upon exposure to aqueous media, leading to potential changes in surface hydrophilicity. In addition, this work demonstrates the ability to fabricate hydrophilic PDMS microfluidic devices that can be self-driven by capillary force using a modified in-mould functionalization approach. We anticipate that similar strategies can be used to spatially pattern PDMS surfaces and microfluidic devices with functional groups attached to molecules containing a PDMS moiety as a surface anchor. Despite the time required to fabricate the modified surfaces (~48 hours) and the need for masking techniques to confine the modifications to the inner walls of microfluidic channels, we believe this method holds potential as a simple and direct way to modify PDMS surfaces with functionalities for applications such as analytical separations, biosensing, cell targeting and isolation, or small molecule discovery.
Acknowledgements
We thank Dr. Emily Cranston for access to AFM equipment and Mouhanad Babi for aid in computer graphics generation. This research was supported through the Natural Sciences and Engineering Research Council (RGPIN/418326) and a Canada Foundation for Innovation Leaders Opportunity Fund. A. F. is partially supported by the BioInterfaces CREATE grant. J. M. M. is the recipient of an Early Researcher Award through the Ontario Ministry of Research and Innovation. We thank EnRoute Interfaces for providing the silicone-based wetting agents. This research made use of instrumentation available through the BioInterfaces Institute at McMaster University.
References
- J. Zhou, D. A. Khodakov, A. V. Ellis and N. H. Voelcker, Electrophoresis, 2012, 33, 89–104 CrossRef CAS PubMed.
- W. Zhang, D. S. Choi, Y. H. Nguyen, J. Chang and L. Qin, Sci. Rep., 2013, 3 Search PubMed.
- V. Sharma, M. Dhayal, Govind, S. M. Shivaprasad and S. C. Jain, Vacuum, 2007, 81, 1094–1100 CrossRef CAS PubMed.
- M. Y. A. J. Fang, J. Micromech. Microeng., 2012, 22, 025012 CrossRef.
- K. Boxshall, M.-H. Wu, Z. Cui, Z. Cui, J. F. Watts and M. A. Baker, Surf. Interface Anal., 2006, 38, 198–201 CrossRef CAS PubMed.
- J. A. Vickers, M. M. Caulum and C. S. Henry, Anal. Chem., 2006, 78, 7446–7452 CrossRef CAS PubMed.
- J. Zhou, A. V. Ellis and N. H. Voelcker, J. Nanosci. Nanotechnol., 2010, 10, 7266–7270 CrossRef CAS PubMed.
- K. Efimenko, W. E. Wallace and J. Genzer, J. Colloid Interface Sci., 2002, 254, 306–315 CrossRef CAS.
- Y. Berdichevsky, J. Khandurina, A. Guttman and Y. H. Lo, Sens. Actuators, B, 2004, 97, 402–408 CrossRef CAS PubMed.
- V. Di Virgilio, S. Bermejo and L. Castañer, Langmuir, 2011, 27, 9614–9620 CrossRef CAS PubMed.
- J. Xu and K. K. Gleason, Chem. Mater., 2010, 22, 1732–1738 CrossRef CAS.
- C. Séguin, J. M. McLachlan, P. R. Norton and F. Lagugné-Labarthet, Appl. Surf. Sci., 2010, 256, 2524–2531 CrossRef PubMed.
- Z. Zhang, X. Feng, Q. Luo and B.-F. Liu, Electrophoresis, 2009, 30, 3174–3180 CrossRef CAS PubMed.
- H. Mao, T. Yang and P. S. Cremer, Anal. Chem., 2001, 74, 379–385 CrossRef.
- T. Yang, S.-Y. Jung, H. Mao and P. S. Cremer, Anal. Chem., 2000, 73, 165–169 CrossRef.
- H. Makamba, Y. Y. Hsieh, W. C. Sung and S. H. Chen, Anal. Chem., 2005, 77, 3971–3978 CrossRef CAS PubMed.
- Y. Liu, J. C. Fanguy, J. M. Bledsoe and C. S. Henry, Anal. Chem., 2000, 72, 5939–5944 CrossRef CAS.
- D. Xiao, H. Zhang and M. Wirth, Langmuir, 2002, 18, 9971–9976 CrossRef CAS.
- S. Tugulu and H.-A. Klok, Macromol. Symp., 2009, 279, 103–109 CrossRef CAS PubMed.
- L. K. Fiddes, H. K. Chan, B. Lau, E. Kumacheva and A. R. Wheeler, Biomaterials, 2010, 31, 315–320 CrossRef CAS PubMed.
- C.-Y. Liao and Y.-C. Su, Biomed. Microdevices, 2010, 12, 125–133 CrossRef CAS PubMed.
- H. Chen, L. Wang, Y. Zhang, D. Li, W. G. McClung, M. A. Brook, H. Sheardown and J. L. Brash, Macromol. Biosci., 2008, 8, 863–870 CrossRef CAS PubMed.
- Z. Zhang, X. Feng, F. Xu, X. Liu and B.-F. Liu, Electrophoresis, 2010, 31, 3129–3136 CrossRef CAS PubMed.
-
M. A. Brook, Y. Wang and Y. Chen, US8,648,211, (to McMaster University) 2014 Search PubMed.
- J. Zhang, Y. Chen and M. A. Brook, Langmuir, 2013, 29, 12432–12442 CrossRef CAS PubMed.
- S. Hu, X. Ren, M. Bachman, C. E. Sims, G. P. Li and N. Allbritton, Electrophoresis, 2003, 24, 3679–3688 CrossRef CAS PubMed.
- L. Song and Y. M. Lam, J. Nanosci. Nanotechnol., 2006, 6, 3904–3909 CrossRef CAS PubMed.
- A. Synytska, E. Biehlig and L. Ionov, Macromolecules, 2014, 47, 8377–8385 CrossRef CAS.
- A. Synytska, E. Svetushkina, D. Martina, C. Bellmann, F. Simon, L. Ionov, M. Stamm and C. Creton, Langmuir, 2012, 28, 16444–16454 CrossRef CAS PubMed.
- P. B. Lillehoj, F. Wei and C.-M. Ho, Lab Chip, 2010, 10, 2265–2270 RSC.
- M. Zimmermann, H. Schmid, P. Hunziker and E. Delamarche, Lab Chip, 2007, 7, 119–125 RSC.
Footnote |
† Electronic supplementary information (ESI) available: Three supplemental figures and five supplemental videos are included. See DOI: 10.1039/c5lc00741k |
|
This journal is © The Royal Society of Chemistry 2015 |
Click here to see how this site uses Cookies. View our privacy policy here.