Coordinating modeling and experimental research of engineered nanomaterials to improve life cycle assessment studies
Received
6th May 2015
, Accepted 24th August 2015
First published on 25th August 2015
Abstract
Life cycle assessment (LCA) – a comprehensive modeling framework used to identify environmental and human health impacts associated with products, processes, and technologies – is increasingly recommended for emerging nanotechnologies. LCA applied prospectively can guide design decisions and enable reduction of future impacts. A growing literature describes the potential for LCA to inform development of safer nanotechnologies, for example by identifying the manufacturing inputs or processes with the greatest potential for improvement. However, few published studies to date include all life cycle stages in part because of uncertainty regarding engineered nanomaterial (ENM) releases and impacts, which precludes comprehensive environmental assessment of nano-enabled products. Life cycle impact assessment (LCIA) converts emissions into environmental damages through linked fate-exposure-effect models that require robust experimental data and a mechanistic understanding for each of these components. In the case of ENMs, there are pertinent knowledge gaps, high uncertainties in experimental data, and disagreement regarding the suitability of existing fate, exposure, and effect models. This frontier review summarizes recent advances in human and aquatic ecotoxicity LCIA for ENMs and calls for greater coordination between LCA modelers and experimentalists, including those that study fate and transport, environmental transformations, occupational exposure, and toxicology, to inform responsible development of nanotechnology, enabling ENMs to reach their full potential.
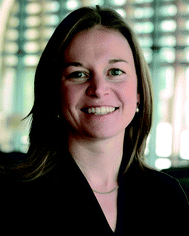
Leanne M. Gilbertson
| Leanne Marie Gilbertson is an Assistant Professor in the Department of Civil and Environmental Engineering at the University of Pittsburgh. After receiving her bachelor's degree in chemistry from Hamilton College in 2007, Dr. Gilbertson was a secondary school teacher for several years. She earned her PhD in environmental engineering from Yale University in 2014 with support from a NSF Graduate Research Fellowship and an EPA STAR Fellowship and remained at Yale as a postdoctoral associate prior to starting at Pitt. Dr. Gilbertson's research aims to inform sustainable design of emerging materials and products to ensure the safe realization of novel technologies. |
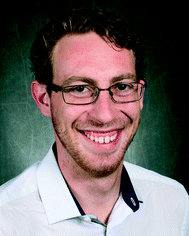
Ben A. Wender
| Benjamin Alex Wender is a PhD candidate in the School of Sustainable Engineering and the Built Environment at Arizona State University (ASU) where he researches life cycle assessment (LCA) for emerging technologies. After receiving a bachelor's degree in physics from Hampshire College in 2008, he was awarded a Center for Nanotechnology in Society-ASU Engineering Fellowship in 2011 to research the intersection of participatory governance and LCA. Most recently he was awarded an EPA STAR fellowship in 2014 to develop life cycle-based tools that aid decision makers in exploring environmental uncertainties early in technology development to support responsible innovation practices. |
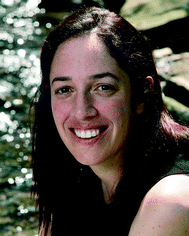
Julie B. Zimmerman
| Julie Beth Zimmerman is a Professor jointly appointed to the Department of Chemical and Environmental Engineering and the School of Forestry and Environment at Yale University. Dr. Zimmerman is the Associate Director for Research at the Yale Center for Green Chemistry and Green Engineering. Her research interests focus broadly on green chemistry and green engineering with a specific focus on renewable chemicals, materials, and fuels, sustainable water treatment, and sustainability assessments of emerging technologies. Further, to enhance the likelihood of successful implementation of these next generation designs, Dr. Zimmerman studies the effectiveness and impediments of current and potential policies developed to advance sustainability. |
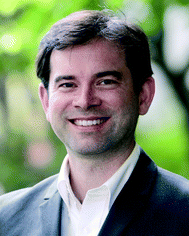
Matthew J. Eckelman
| Matthew Eckelman is an Assistant Professor at Northeastern University in Civil and Environmental Engineering, with secondary appointments in Chemical Engineering and Public Policy. His research interests include energy and emissions modeling, life cycle assessment, and environmental analysis of novel materials. Dr. Eckelman consults regularly on sustainability-related projects with a range of businesses, non-profit institutions, and government agencies, and has served on panels at the U.S. National Academies and the National Institute for Standards and Technology on sustainable materials and chemical life cycle issues. |
Nano impact
Comprehensive life cycle impact assessment (LCIA) of engineered nanomaterials (ENMs) and nano-enabled products requires quantification of impacts associated with conventional chemical and ENM releases. However few published assessments to date address nano-scale emissions, which precludes use of LCIA to identify the most significant environmental and human health ‘hot spots’. This frontier review summarizes recent advancements and challenges in LCIA for ENMs, focusing on human and ecotoxicity impact assessment models, and identifies recent nano-specific environment, health, and safety literature with promise to inform LCIA model development. Throughout, the manuscript calls for closer collaboration between experimental investigation and modeling research such that experimental data collection is prioritized according to the greatest life cycle uncertainties and modeling needs.
|
Introduction
The historical focus on designing for function without a complementary focus on hazard has led to the unintended environmental and human health consequences of widely utilized substances such as asbestos and dichlorodiphenyltrichloroethane (DDT), motivating a more proactive and comprehensive approach to evaluating emerging chemicals, materials, and products. The potential widespread use of engineered nanomaterials (ENMs) and nano-enabled products for applications in diverse sectors (e.g., health care, consumer products, electronics, national defense, and environmental remediation) is coupled with concern over adverse impacts upon exposure to humans and the environment. Releases of ENMs can occur at multiple stages along the life cycle of a nano-enabled product, for example as uncontrolled emissions during ENM synthesis, wear-and-tear during use, or from waste management facilities processing nano-wastes and nano-enabled products. The chemical and physical form of the emissions varies along these points, as does the potential for human or ecological exposures, which necessitates a life cycle perspective when approaching issues of holistically designing safer nanomaterials. In addition to the potential adverse impacts of emitted ENMs themselves, there are concerns from non-nano emissions associated with nano-enabled products. For example, the formation and potential release of harmful polyaromatic hydrocarbons and volatile organic compounds during carbon nanotube (CNT) synthesis.1,2 As such, an approach that is pro-active, life-cycle based, and uses multiple criteria is necessary to identify potential unintended consequences and contribute to responsible development of ENMs and nano-enabled products.
Life cycle assessment (LCA) is one such approach that has been recommended by the National Nanotechnology Initiative4 and the National Research Council3,4 and is increasingly applied for ENMs and nano-enabled products. LCA, widely used in the chemical and product manufacturing sectors, is a systems-level methodology for evaluating environmental and human health impacts associated with a product or process. LCA methods have been prescribed in a series of international standards,5,6 and consist of four steps: (1) goal and scope definition, where the unit of analysis and system boundary of the study is established; (2) life cycle inventory (LCI) modeling, which accounts for each discrete energy and material input and emission across the life cycle of the product – including activities such as mining, processing of primary materials, manufacturing, use, transportation, and disposal; (3) life cycle impact assessment (LCIA), which uses coupled fate-exposure-effect models to translate the mass of each emission in the LCI into a quantified measure of potential environmental and/or human health impacts using so-called characterization factors (CFs); and (4) interpretation of results. LCA is a multi-criteria assessment tool, as separate CFs are applied for each substance and across a variety of impact categories such as global warming potential, ozone depletion, human toxicity and ecotoxicity, as presented in Fig. 1.
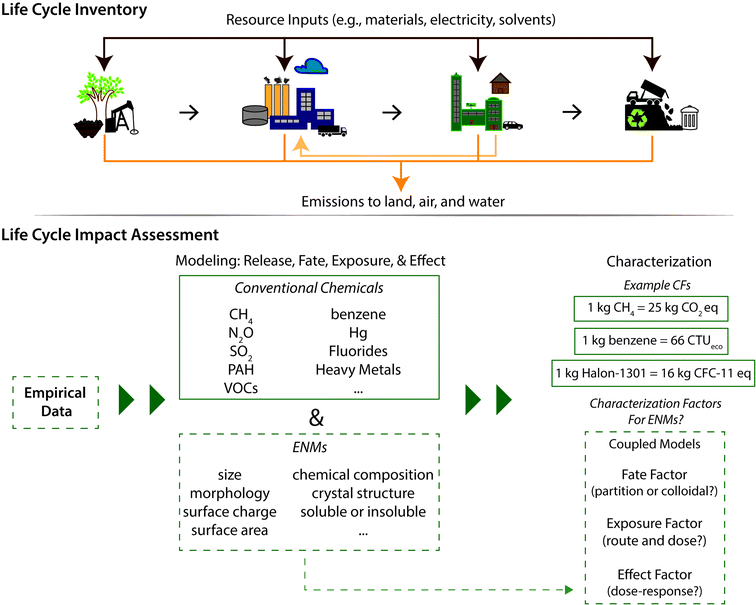 |
| Fig. 1 Schematic representation of the life cycle stages and overview of the life cycle assessment (LCA). Life cycle inventory analysis quantifies resource use and emissions across the product life cycle and mid-point impact assessment uses characterization factors to convert LCI entries into environmental and human heath damages. Dashed lines in the schematic represent active research areas in need of greater coordination between experimental and modeling efforts as it related to nanomaterials and nano-enabled products. eq. = equivalents. CTUeco = comparative toxicity units for ecotoxicity. | |
Recent reviews summarize the accomplishments and critical challenges encountered in the application of LCA to the study of ENMs and nano-enabled products.7–9 These reviews draw several important conclusions including: 1) the majority of nano LCA studies to date are cradle-to-gate and do not include use or end-of-life considerations and 2) ENM releases are not commonly considered at any stage, which is in part due to the lack of inventory data and characterization factors for ENMs, as well as the significant uncertainty in use- and end-of-life stages;7–9 3) initial incorporation of this critical information, as it becomes available, can be facilitated by using existing tools and experimental data;9 4) many nano LCAs are constrained to mass-based functional units, which is inappropriate for quantifying product functionality comparisons as is standard in LCA;8 and 5) given the previously identified shortcomings, much can be learned from qualitative or screening-level assessments.7,9 This is not an unusual state of affairs for the assessment of emerging technologies,10 as environmental modeling tools are routinely adapted to incorporate new substances and experimental data.
Nano LCA studies to date have been informative though remain limited in scope. As noted previously, the work has largely focused on indirect impacts, such as ENM production and manufacturing of nano-enabled products, while excluding assessment of nano emissions from products or in pure form (particularly in the use and end-of-life stages).7–9 For example, recent cradle-to-gate studies on nanocellulose11,12 and graphene13 compare the environmental impacts of alternative production processes to identify the least burdensome process. Studies of carbon nanotubes (CNTs) call attention to the high resource and energy intensity of their production, associated with the high temperatures, pressures, and low reaction yields typical of CNT synthesis processes, particularly single-walled CNTs.1,14 Yet, when considering these manufacturing impacts within the context of a product that contains small amounts of CNTs, such as a semiconductor device, the contribution of CNT manufacturing to the overall life cycle impacts of the product may be minimal.15,16 This is similar for metallic nanoparticles, in which the life cycle impacts have been found to be dominated by bulk metals such as gold and silver, whose mining and refining is energy intensive.17,18
The few efforts to incorporate ENM emissions into life cycle studies examine the relative impacts of production and use- or end-of life phases by adapting current LCIA methods. A recent comparative LCA of three nano-enhanced paints included TiO2 emissions and ecotoxicity impacts using recently published characterization factors (although nano silver and silica were omitted), to show net improvement if TiO2 was substituted for other active ingredients and paint lifetime increased.19 Another study investigating the ecotoxicity of single-walled CNTs found that production impacts due to non-nano emissions were orders of magnitude greater than the impacts of CNT releases under a realistic release and environmental fate scenario.20 Furthermore, the behavior of ENMs in the environment is influenced by the chemistry of the surrounding media, such as pH, dissolved organic carbon (DOC), and ionic strength. For example, silver nanoparticles have been shown to react rapidly with sulfur, resulting in decreased Ag+ release and ecotoxicity impact potential.21,22 In addition to these exogenous factors, ENM fate, exposure, and toxicity are influenced by a number of physical and chemical properties associated with their nano length scale, including large surface area, chemistry, reactivity, charge, morphology, and agglomeration state.23,24 These factors, vitally important to describing ENM behavior, are notably different than the current modeling considerations of molecules rather than particles, which present a challenge in directly utilizing or adapting current models. One exception is that of particulate matter (PM), for which several methods25–28 consider size by using different CFs for PM 2.5–10 μm in diameter (PM10) and PM less than 2.5 μm (PM2.5); nonetheless this approach does not account for heterogeneity in PM composition, morphology, or reactivity. Demonstrating the complexity of this effort, a recent analysis accounts for the heterogeneity of PM by developing more than 2700 distinct characterization factors for the complex components of PM, and concludes that if less-harmful particles such as salt aerosols are omitted, established methods significantly overestimate PM impacts.29
Despite the progress in nano LCA described above, the capabilities of the current life cycle impact assessment models remain inapplicable in a comprehensive and universal manner to ENMs and the products they enable. Experimental studies pertaining to ENM transport, fate and transformations in the environment, occupational safety, and nanotoxicology continue to advance such that significant data and expertise are available to inform LCIA. Nonetheless, ENM-specific impact assessment models or CFs are not included in any commercial or publically available LCIA packages. Given the large number of ENMs, release scenarios, surface modifications, and possible permutations of these characteristics, there exists a need to prioritize data collection30 and improve model parsimony. Table 1 compiles prominent life cycle concerns associated with several commercially relevant ENM classes and calls attention to their similarities and differences pertinent to LCIA. The final column in Table 1 suggests those midpoint impact categories most relevant to the given ENM class based on the product categories, potential for release, exposure routes, transformations, and mechanisms of biological activity. It becomes clear that certain impact categories are more relevant for certain ENM classes and life cycle stages as indicated by the frequency of appearance throughout the table (e.g., human and ecotoxicity categories).
Table 1 Compiled overview of six engineered nanomaterial (ENM) classes,a including the respective primary applications and relevant sectors, potential benefits realized by enabling products with the nanomaterial, potential release and exposure routes, relevant transformation mechanisms, and primary life cycle impact indicators of interest
ENM class |
Primary applications & sectors |
Potential realized benefits by nano-enabling |
Potential release and exposure routes |
Relevant transformations and mechanisms |
Primary life cycle impact indicators of interest |
ENM classes were chosen based on their production volumes, current and proposed market presence.31,126–128
|
Nano-silver95,112–115 |
Anti-odor textiles (e.g., sheets, towels, shirts, socks, pants)
Composites (e.g., hospital equipment, washing machine, cosmetics)
Wound dressings
Antimicrobial surfaces (e.g., paints, coatings)
Filters (e.g. for air/water purification & sterilization)
Catalyst (e.g., CO oxidation)
Consumer electronics
|
Reduced laundering
Prevention of bacterial and viral transmission
Faster wound healing
Improved access to potable water
Increased reaction time (decreased energy barrier)
|
Manufacture: occupational exposure during handling
Use phase: release of nano-silver and Ag+ ions from products
End of life: release of Ag+ from WWTP effluent; low hazard concern when in non-ionic form (e.g., Ag2S, AgCl)
|
Oxidation of Ag0 to Ag+ leading to:
Sulfidation (Ag2S)
Chlorination (AgCl)
Re-precipitation of nano-Ag upon reduction of Ag+
Disruption of biological function (e.g., via ROS production, extra- and intra-cellular Ag+ interactions)
|
Raw materials & manufacture
Global warming potential
Fossil fuel depletion
Mineral resource depletion
Human toxicity
Use & end of life
Terrestrial, freshwater & marine ecotoxicity
Human toxicity
Non-carcinogenics
|
|
Carbon nanotubes1,20,112,114,116–118 |
Composites (e.g., vehicles, infrastructure, athletic gear, protective clothing)
Electronics (e.g., memory chips, sensors)
Batteries
Biomedical (e.g., sensors, scaffolds)
Flame retardant
Filters and membranes (e.g., water purification & disinfection)
Antimicrobial surfaces
|
Light-weight high performance materials
Reduced energy consumption
Enhanced detection of harmful analytes
Improved early detection of maladies
Improved access to potable water
|
Manufacture: VOC & PAH formation; occupational exposure (e.g., inhalation) during handling
Use phase: release as raw material, transformed material, or as composite during wear-and-tear of products
End of life: release and exposure during resource recovery (e.g., electronics, plastics or fabric recycle); landfill accumulation; complete combustion during incineration
|
Surface transformations
Agglomeration, homo/hetero aggregation and settling
Physical (e.g., frustrated phagocytosis) and chemical (e.g., oxidative stress) induction of adverse impacts on cells and organisms (e.g., delayed growth or hatching, developmental malformations)
|
Raw materials
Global warming potential
Fossil fuel depletion
Mineral resource depletion
Human toxicity
Manufacture
Fossil fuel depletion
Mineral resource depletion
Particulate matter formation
Criteria air pollutants
Acidification
Eutrophication
Human toxicity
Carcinogenics
Non-carcinogenics
Use & end of life
Particulate matter formation
Terrestrial, freshwater, & marine ecotoxicity
Human toxicity
Carcinogenics
Non-carcinogenics
|
|
Nano-TiO2 112,114,119,120 |
Nano-TiO2 functions primarily as a pigment (i.e., whitening agent), UV protectant, and photocatalyst in:
Paint
Food
Sunscreen
Deodorant
Self-cleaning coatings
Air & water filters
Environmental remediation
Dye-sensitized solar cells
|
Reduced washing of exterior surfaces
Reduced risk of skin cancer
Improved access to potable water
|
Manufacture: occupational exposure during handling
Use phase: direct application (i.e., cosmetic applications), release during wear and washing
End of life: landfill accumulation (e.g., residual cosmetic during container disposal)
|
Agglomeration, homo/hetero aggregation and settling
Photoinduced production of reactive oxygen species (e.g., oxygen radical) causing disruption of healthy biological function
|
Raw materials & manufacture
Global warming
Fossil fuel depletion
Mineral resource depletion
Human toxicity
Use & end of life
Terrestrial, freshwater, & marine ecotoxicity
Human toxicity
Non-carcinogenics
|
|
Nano-ZnO114,115,120,121 |
Nano-ZnO functions primarily as a pigment and semiconductor in:
Sunscreen
Paints
Antimicrobial fabrics
Deodorant
Rubber additives
Solar cells
LCDs
|
Reduced risk of skin cancer
Reduced exterior washing
Realization of alternative energy resources
|
Manufacture: occupational exposure during handling
Use phase: direct application (e.g., cosmetic applications), release during wear and washing
End of life: landfill accumulation (e.g., residual cosmetic during container disposal)
|
Agglomeration, homo/hetero aggregation and settling
Oxidative stress induced adverse response
Adverse impacts caused by dissolution (Zn2+)
|
Raw materials & manufacture
Global warming
Fossil fuel depletion
Mineral resource depletion
Human toxicity
Use & end of life
Terrestrial, freshwater, & marine ecotoxicity
Human toxicity
Carcinogenics
Non-carcinogenics
|
|
Nano-CeOx 112,114,122–125 |
Catalyst (e.g., methane steam reforming)
Fuel additive
Polishing agent
UV coatings & paints
Chemical mechanical planarization
Solid oxide fuel cells
Batteries
|
Reduced criteria pollutants (e.g., oxidation of CO to CO2) emissions
UV blocker
Bioprotective effects against oxidant injury
|
Manufacture: occupational exposure during handling
Use phase: release to environment from use (e.g., fuel additive, coatings) or WWTP effluent discharge
End of life: proper disposal and recycle of high value applications limits exposure (e.g., batteries, fuel cells)
|
Agglomeration, homo/hetero aggregation and settling
Surface adsorption (e.g., via hydroxyl groups)
Storage and release of oxygen
Dual oxidation state (Ce+3–Ce+4) gives rise to unique redox properties (e.g., oxidant and antioxidant effects)
|
Raw materials & manufacture
Global warming
Fossil fuel depletion
Mineral resource depletion
Human toxicity
Use & end of life
Terrestrial, freshwater, & marine ecotoxicity
Human toxicity
Non-carcinogenics
|
The need for environmental research prioritization is not unique to ENMs and nano-enabled products, but rather is shared by other emerging technologies. Meaningful inclusion of ENM releases, fate, exposure, and effects in LCIA models can be accelerated through coordinated efforts between experimentalists and life cycle modelers. Specifically, cooperative efforts early in experimental design can tailor data collection toward the greatest modeling uncertainties while fostering development of innovative modeling approaches. In particular, there is a need for sensitivity analyses of LCIA models to identify which parameters are most influential to model results and then integrating these data needs into experimental design to narrow specific uncertainty ranges. These data needs and modeling advances are discussed first as they relate to ENM releases (treated as environmental emissions in the life cycle inventory) and second to development of nano-specific characterization factors (life cycle impact assessment). While this review identifies many nano-LCIA experimental and modeling challenges, it cautions attempts to create nano-specific models that are overly detailed and have limited utility for risk modeling or decision making. Rather, coordination between both experimental and modeling approaches can enable iterative sensitivity analyses, direct data sharing that can identify which uncertainties are significant while others may be revealed as low priorities for further investigation, and inform experimental designs and priorities according to the greatest life cycle uncertainties.
Quantifying and characterizing life cycle releases of ENMs
Estimating the quantity and characteristics of ENM releases is the first step in LCI modeling, which tracks the mass and receiving compartment (e.g., air, water, soil) of all emissions across the life cycle. Initial global estimates of ENM emissions31,32 have since been improved through increased geospatial resolution33 and site specificity such as waste water treatment plants34,35 and end-of-life disposal activities such as incineration.36 In addition to considering emissions at the global scale, ENM emissions are likely to differ across end-use applications and the physiochemical characteristics of emitted ENMs may change in each life cycle stage. Several recent studies consider potential ENM releases from select product classes including personal care products,37,38 composite materials,39,40 and paint formulations.41,42 Since it is impracticable to include all possible permutations of raw, transformed and composite ENM emissions, there is active discussion regarding which physicochemical properties of ENM emissions are most important to characterize and how these may be integrated into mechanistic impact assessment models.43
Incorporation of ENM properties, namely shape and size, in LC inventory modeling was recently recommended in addition to mass and chemical composition44 that are currently considered for conventional chemicals. This approach stops short of tracking changes in ENM morphology and physiochemical properties when released from different products and life cycle stages – for example the loss or gain of surface functional groups – that will influence their fate, exposure, and toxicity potentials. This is problematic, as LCI modeling of conventional chemicals sums the total mass of each emitted material across all life cycle stages, implicitly assuming that releases from manufacturing, use, and end-of-life are environmentally equivalent. Thus, experimental efforts to quantify life cycle ENM releases should prioritize commercially-relevant nano-enabled products and explore the extent to which environmental residence times, bioavailability, and toxicity change across the life cycle or with different surface modifications. This can inform LCI modeling by identifying which ENM emissions and release scenarios are suitably different to necessitate distinct LCI entries and those that may be grouped into one entry with minimal increases in uncertainty.
Toward the development of nano-specific characterization factors
LCIA begins with classification of all emissions according to the impact categories to which they contribute, followed by quantification of their relative impact through characterization factors (CFs). CFs provide a quantitative measure of the impact potential associated with each emission, and are calculated per unit mass emitted to a specified environmental compartment. The consensus model USEtox45,46 is recommended practice for human and aquatic ecotoxicity impact assessment,47 and has recently been adapted – as discussed in greater detail below – to estimate CFs for several ENMs. CFs are calculated as the product of:
1. Fate factor (FF), which represents the fate, transport, and residence time of an emission in each environmental compartment, and is obtained through simplified multi-media box models,
2. Exposure factor (XF), which accounts for intake by multiple species and/or humans through known exposure routes, including ingestion and inhalation, and
3. Effect factor (EF), which represents the aggregated toxicological response of multiple organisms or humans upon exposure to a known dose.
Experimental data and mechanistically appropriate models are required to calculate each of these factors and to reduce the high ENM parameter and model uncertainty. In the following sections the relatively small but growing body of literature advancing LCIA of ENMs is reviewed, with a focus on published methodological improvements arising from the sustained interest in the environmental impacts of ENMs.
Fate factor: modeling environmental transport and residence times of ENMs
LCIA fate models – for example the European-developed USES-LCA 2.048,49 used in ReCiPe50 and the consensus model USEtox45,46 now adopted by TRACI25 – rely on multi-media mass balance models following a fugacity approach.51 The applicability of this approach to ENMs, which is based on equilibrium partitioning coefficients originally developed and applied to organic pollutants, is the subject of recent scrutiny and debate.52–55 Use of equilibrium partitioning coefficients as an indicator of ENM fate may be misleading given that ENM suspensions violate key thermodynamic assumptions associated with use of equilibrium partitioning coefficients.55 To address this critique, several fate models developed specifically for ENMs56–59 – described in greater detail in Dale et al.53 and Scheringer et al.60 – replace partitioning assumptions with elements of colloid theory. Nonetheless, substitution of colloidal models requires simplifying assumptions and does not reduce model uncertainty. Thus, informed, skeptical use of partitioning-based models remains a recommended practice until further research validates one approach over another.54 Following this reasoning, several recent nanomaterial fate models employ partitioning approaches with ENM-specific data and size-dependent binning61,62 of results. This presents LCIA model developers with a decision of how best to evaluate the fate of ENMs using existing models or by developing ENM-specific models based on limited understanding.7
To date necessary, albeit minor, modifications to existing LCIA models have been made to account for both model and parameter uncertainty in FF calculations for ENMs, and focus largely on freshwater aquatic residence time for CNTs20,63,64 and TiO2.65 Miseljic and Olsen7 simply assume a FF equal to one day for nano Ag and TiO2 ENMs, citing lack of information and likelihood of rapid transformation, aggregation, and sedimentation. Other studies account for removal through aggregation/agglomeration by comparing scenarios of fixed percentage removal,20 modeling FFs probabilistically based on the wide range of published parameter estimates,64 or with qualitative discussion of uncertainty.63 Salieri et al.,65 make the most significant modifications to USEtox by: 1) developing a simplified heteroaggregation model and exploring scenarios of alternative attachment efficiencies, and 2) binning TiO2 ENM emissions based on particle size and calculating distinct FFs for each size range. Aside from aggregation and deposition, none of these analyses quantitatively consider other ENM transformations or surface modifications – for example, stabilization of CNTs by natural organic matter66 or sulfidation of nano Ag.21 A probabilistic approach may capture some of this uncertainty and material variability20,64 though not mechanistically, and further experimental investigation is necessary to validate one fate modeling approach over another.67
While there is significant uncertainty surrounding current ENM fate modeling approaches, experimentalists can expedite resolution that is more appropriate for a given class of ENMs. Future LCIA model development should assess the sensitivity of CF results to those mechanisms relevant to ENMs, including removal, stabilization and transformations for separate classes of ENMs since their behavior can be markedly different (e.g., carbonaceous vs. nanocellulose vs. metal and metal oxide ENMs). Those physicochemical properties that most determine fate for each ENM class should then be required in specifying ENM emissions in the life cycle inventory. In addition, adjustments made to existing models, such as USEtox, should aim to include these nano-relevant removal pathways, including aggregation and settling.
Exposure factor: quantifying the fraction of ENMs available to humans and organisms
For conventional chemical emissions, exposure modeling in (eco)toxicity impact assessment relates the residence times of an emission in each compartment to the intake fraction by people or biota, called the exposure factor (XF). The presently available version of USEtox includes human exposure via inhalation and ingestion of plants, meat, fish and dairy products, but does not yet include workplace, indoor, or dermal exposure routes. For conventional pollutants, inhalation dominates for air-phase releases or emissions to water that are volatile, whereas consumption exceeds inhalation only in cases of low volatility and high lipophilicity.68 Exposure to aquatic organisms is modeled as the dissolved fraction of an emission, where partitioning to suspended solids, dissolved organic carbon, and biota (again, calculated using equilibrium partitioning coefficients) decreases the bioavailable fraction of the emission.69 None of these approaches are designed for nanomaterials, for which aggregation influences the number of free particles and their effective size, density, and diffusion rates.70 Thus mass and particle concentrations reported and administered in many in vivo studies may overestimate the delivered dose.71 LCIA exposure models for ENMs could adopt additional dose-conversion calculations,72 potentially taking guidance from recent protocols coupling experimental characterization of life cycle ENM releases with dosimetry modeling.73 Human exposure research has focused on consumer74–76 and occupational settings77 with strong guidance for ENM inhalation XF and CF calculations recently described in Walser et al.78
No published ENM ecotoxicity CFs mechanistically account for uncertainty in exposure modeling. Several studies adopt the precautionary assumption that 100 percent of ENM emissions are bioavailable7,65 while others apply Monte Carlo sampling across published partitioning coefficient ranges.20,64 Similar to FF calculations, none of these studies directly account for ENM transformations and how these may influence exposure estimates. Reported XF values for CNTs span approximately six orders of magnitude,63 suggesting that research directed towards elucidating exposure mechanisms and improving resolution in XF calculations can substantially reduce CF uncertainty. Only one study calculates XF for human toxicity using USEtox, and concludes that – regardless of emission compartment – ingestion of CNTs through consumption of fish and plants significantly exceeds intake through inhalation.63 Nonetheless, like chemicals, exposure through inhalation may be significant in the case of localized high-concentrations of ENMs (e.g., occupational settings), and LCIA model developers should continue to improve existing workplace studies79,80 and build consensus and wider adoption of recent guidance.78,81,82
Effect factor: linking toxicity data to adverse environmental and human health impacts
For conventional chemicals, toxic effect modeling in LCIA is one of the greatest contributors to uncertainty in CF calculations. The effect factor (EF) represents potential adverse human and environmental health impacts derived from the toxicity of the emission, and is based on extrapolation from animal models or multi-species toxicity data, respectively. Quantification of (eco)toxicity effects is based on chemical hazard indicators such as average LC or EC50 values obtained from experimental measurement of the concentration at which the effect was observed in 50% of the population. ReCiPe and USEtox rely on the same toxicity databases,83 in which coverage of conventional chemicals is oftentimes incomplete or based on relatively few data points.84 Extensive human health and ecotoxicological testing of all new chemicals to determine inherent toxicity characteristics is not feasible due to the number of new substances introduced daily, the time it takes to conduct reviews, and the prohibitive economic and social costs of testing, particularly in vivo.85,86 These challenges are exacerbated in the context of ENMs, where even less data are available and the role of transformations and colloidal behavior may require modifications to existing testing protocols.87,88 The few early studies calculating EFs for ENMs use ENM-specific toxicity values reported in the literature to build multi-species sensitivity distributions following USEtox guidelines.45 Similar to XF and FF, calculating EF probabilistically as demonstrated in application to CNTs20,64 provides one pathway to address parameter uncertainty in reported EC50 values. Furthermore, enhanced mechanistic understanding, specifically increased resolution of toxic modes of action (TMoA) and reduced inter-species uncertainties, has been shown to contribute orders of magnitude uncertainty to EF calculations for conventional chemicals.89–91
In addition, there are several challenges to obtaining reliable data for ENMs using conventional toxicological assays. First, many nanomaterials are insoluble and thus, have a tendency to rapidly agglomerate and settle out of solution. This makes dosimetry determination challenging, as discussed previously. As it relates to the EF specifically, insoluble ENM behavior hinders determination of the actual delivered dose – a topic that remains under critical review70,92,93 – because the delivered dose likely differs from the mass-based concentration of ENMs in the system. Alternative approaches to estimating the delivered dose include on the basis of number of particles (#/L) or total surface area of the particles (m2 L−1).94,95 Another challenge to utilizing conventional toxicological assays to study ENMs is that carbon-based ENMs have been shown to interact with many of the commonly used dyes and essential nutrient compounds leading to false readings from fluorescent quenching and organism growth inhibition, respectively.96,97 Combined, these make the applicability of high throughput screening (HTS) – a rapid, highly automated approach to in vivo and in vitro toxicity testing – across all ENM classes virtually impossible. Still, HTS offers a promising avenue for efficient data collection and has provided useful results for certain classes of ENMs (e.g., metal and metal oxide).98–101 Finally, there is mounting concern over the relevance of the studied toxicological endpoints under extremely high ENM concentrations and relatively few studies that consider the transformed or weathered ENMs that may be of greater relevance to actual release scenarios.102–104 Impacts of ENM releases combined with experimental investigations that demonstrate the importance of ENM transformations in environmentally relevant conditions102,105–107 can motivate improvements to latent issues in LCIA. Specifically, LCIA toxicity modeling tends to exclude impacts of transformation byproducts, inclusion of which has been shown to significantly alter EF estimates for conventional chemicals.108
While there remain several challenges for insoluble ENMs, there is increased evidence for a distinct difference in the magnitude of toxicity of released ions upon dissolution of nanomaterials compared to the parent nanomaterial, and is particularly true for silver nanoparticles (with enhanced surface area to volume ratio and thus, more surface atoms, nanomaterials undergo dissolution more rapidly than their compared to their bulk counterparts).103,109 A recent meta-analysis comparing the ecotoxicity of three soluble ENMs – nano Ag, CuO, and ZnO – to their ionic counterparts found that the ENMs displayed reduced toxicity in the majority of studies and exceed the ionic form only in worst case scenarios.110 Thus, studies that assume a fixed percentage ionic release from ENMs18,111 and rely on existing EFs and CFs for ionic metal potentially overestimate toxicity impacts. To this end, there is the opportunity for enhanced resolution of environmental and human health impact evaluation of ENMs. With the currently available data it is possible to develop novel or update current effect models that will elucidate relevant obstacles and therefore, be able to direct future toxicological data acquisition.
Catalyzing research synergies to inform development of safer nano-enabled products
The numerous data gaps, high uncertainty in experimental protocols and published parameter estimates, and rapid evolution of modeling approaches leaves development of robust LCI data and ENM-specific impact assessment models an ongoing endeavor. The possible permutations resulting from the large number of ENMs, associated surface modifications, and release scenarios creates a need for research prioritization that can be facilitated through greater coordination between modeling and experimental approaches. The early efforts reviewed herein point to innovative LCIA modeling approaches that, in the absence of clear mechanistic understanding, have combined probabilistic uncertainty modeling with scenario development to produce actionable results. In outlining where recent experimental advances can inform modifications to CF calculation for ENMs this review identifies several specific recommendations for experimentalists and LCA modelers to coordinate research agenda to streamline progress toward responsible development of nano-enabled products.
1) It is of critical importance to include ENM releases in nano LCA studies – despite uncertainties of current models and data – to assess the relative magnitude of ENM emissions within broader life cycle impacts.
2) Rather than adopting one fate modeling approach (e.g., either partitioning or colloidal), LCIA method developers should evaluate the sensitivity of FF and CF results to the choice of fate model as a way to prioritize further experimental investigation.
3) Robust impact assessments rely on relevant information being included in the life cycle inventory. Size and morphology have been recommended in specifying ENM emissions. In addition to identifying ENM attributes that govern property-hazard relationships, we recommend a consensus process around which ENM attributes are important in determining ENM fate and subsequent inclusion of these attributes during the life cycle inventory stage.
4) Not all life cycle impact categories are of equal concern when considering direct environmental and human health impacts of ENMs, especially under different release scenarios. As such, it is suggested to build consensus regarding priority categories and release scenarios for which nano-specific characterization factors will most improve understanding of these impacts.
5) Experimental investigations should follow an analytical sequence that considers first, the potential and likelihood of ENM release at each life cycle stage, the transport and fate of the released ENM (in parent, transformed, and/or complex matrix form), exposure of the released ENM (including appropriate dosimetry considerations), and finally, the effect (adverse or otherwise) caused by exposure to the delivered dose.
6) LCIA of ENM-enabled products requires more detailed characterization of ENMs as they are released from products (e.g., aged, transformed, composites) and overtime, as opposed to the raw or pristine forms.
Acknowledgements
LMG, BAW, and JBZ acknowledge the generous support of the U.S. Environmental Protection Agency (EPA) Assistance Agreement no. RD83558001-0. BAW acknowledges support from the EPA Science to Achieve Results program through grant no. FP917643 and the National Science Foundation (NSF) through grant no. ECCS-1140190 and grant no. SES-0937591. MJE recognizes the support from the NSF through award SNM-1120329. This work has not been formally reviewed by EPA. The views expressed in this document are solely those of the authors and do not necessarily reflect those of the Agency. EPA does not endorse any products or commercial services mentioned in this publication.
References
- D. L. Plata, A. J. Hart, C. M. Reddy and P. M. Gschwend, Early evaluation of potential environmental impacts of carbon nanotube synthesis by chemical vaport deposition, Environ. Sci. Technol., 2009, 43(21), 8367–8373 CrossRef CAS PubMed.
- S. Gavankar, S. Suh and A. A. Keller, The role of scale and technology maturity in life cycle assessment of emerging technologies: A case study on carbon nanotubes, J. Ind. Ecol., 2015, 19(1), 51–60 CrossRef CAS PubMed.
-
A Research Strategy for Environmental, Health, and Safety Aspects of Engineered Nanomaterials, National Research Council of The National Academies, Washington, D.C., 2012 Search PubMed.
-
National Nanotechnology Initiative: Environmental, Health, and Safety Research Strategy, National Science and Technology Council Committee on Technology, 2011 Search PubMed.
- ISO 14044: Environmental management - Life cycle assessment - Requirements and guidelines.; Switzerland, 2006.
-
ISO 14040: Environmental management - Life cycle assessment - Principles and framework, Switzerland, 2006 Search PubMed.
- M. Miseljic and S. I. Olsen, Life-cycle assessment of engineered nanomaterials: A literature review of assessment status, J. Nanopart. Res., 2014, 16, 1–33, DOI:10.1007/s11051-014-2427-x.
- R. Hischier and T. Walser, Life cycle assessment of engineered nanomaterials: State of the art and strategies to overcome existing gaps, Sci. Total Environ., 2012, 425, 271–282 CrossRef CAS PubMed.
- S. Gavankar, S. Suh and A. A. Keller, Life cycle assessment at nanoscale: Review and Recommendations, Int. J. Life Cycle Assess., 2012, 17(3), 295–303 CrossRef.
- A. C. Hetherington, A. L. Borrion, O. G. Griffiths and M. S. McManus, Use of LCA as a development tool within early research: Challenges and issues across different sectors, Int. J. Life Cycle Assess., 2014, 19(1), 130–143 CrossRef.
- Q. Li, S. McGinnis, C. Sydnor, A. Wong and S. Renneckar, Nanocellulose life cycle assessment, ACS Sustainable Chem. Eng., 2013, 1(8), 919–928 CrossRef CAS.
- R. Arvidsson, D. Nguyen and M. Svanstrom, Life cycle assessment of cellulose nanofibrils production by mechanical treatment and two different pretreatment processes, Environ. Sci. Technol., 2015, 49(11), 6881–6890 CrossRef CAS PubMed.
- R. Arvidsson, D. Kushnir, B. Sanden and S. Molander, Prospective life cycle assessment of graphene production by ultrasonication and chemical reduction, Environ. Sci. Technol., 2014, 48(8), 4529–4536 CrossRef CAS PubMed.
- V. K. Upadhyayula, D. E. Meyer, M. A. Curran and M. A. Gonzalez, Life cycle assessment as a tool to enhance the environmental performance of carbon nanotube products: A review, J. Cleaner Prod., 2012, 26, 37–47 CrossRef CAS PubMed.
- L. J. Dahlben, M. J. Eckelman, A. Hakimian, S. Somu and J. A. Isaacs, Environmental life cycle assessment of a carbon nanotube-enabled semiconductor device, Environ. Sci. Technol., 2013, 47, 8471–8478 CAS.
- L. M. Gilbertson, A. A. Busnaina, J. A. Isaacs, J. B. Zimmerman and M. J. Eckelman, Life cycle impacts and benefits of a carbon nanotube-enabled chemical gas sensor, Environ. Sci. Technol., 2014, 48, 11360–11368 CrossRef CAS PubMed.
- P. Pati, S. McGinnis and P. J. Vikesland, Life cycle assessment of ‘green’ nanoparticle synthesis methods, Environ. Eng. Sci., 2014, 31(7), 410–420 CrossRef CAS.
- L. Pourzahedi and M. J. Eckelman, Environmental life cycle assessment of nanosilver-enabled bandages, Environ. Sci. Technol., 2015, 49(1), 361–368 CrossRef CAS PubMed.
- R. Hischier, B. Nowack, F. Gottschalk, I. Hincapie, M. Steinfeldt and C. Som, Life cycle assessment of facade coating systems containing manufactured nanomaterials, J. Nanopart. Res., 2015, 17(68), 1–13, DOI:10.1007/s11051-015-2881-0.
- M. J. Eckelman, M. S. Mauter, J. A. Isaacs and M. Elimelech, New Perspectives on Nanomaterial Aquatic Ecotoxicity: Production Impacts Exceed Direct Exposure Impacts for Carbon Nanotubes, Environ. Sci. Technol., 2012, 46(5), 2902–2910 CrossRef CAS PubMed.
- D. L. Starnes, J. M. Unrine, C.
P. Starnes, B. E. Collin, E. K. Oostveen, R. Ma, G. V. Lowry, P. M. Bertsch and O. V. Tsyusko, Impact of sulfidation on the bioavailability and toxicity of silver nanoparticles to Caenorhabditis elegans, Environ. Pollut., 2015, 196, 239–246 CrossRef CAS PubMed.
- R. D. Kent, J. G. Oser and P. J. Vikesland, Controlled evaluation of silver nanoparticle sulfidation in a full-scale wastewater treatment plant, Environ. Sci. Technol., 2014, 48(15), 8564–8572 CrossRef CAS PubMed.
- B. Fubini, M. Ghiazza and I. Fenoglio, Physico-chemical features of engineered nanoparticles relevant to their toxicity, Nanotoxicology, 2010, 4(4), 347–363 CrossRef CAS PubMed.
- M. Zhu, G. Nie, H. Meng, T. Xia, A. Nel and Y. Zhao, Physicochemical properties determine nanomaterial cellular uptake, transport, and fate, Acc. Chem. Res., 2013, 46(3), 622–631 CrossRef CAS PubMed.
-
Tool for the reduction and assessment of chemical and other environmental impacts (TRACI): TRACI version 2.1, User's guide, U.S. Environmental Protection Agency: Cincinnati, OH, 2012 Search PubMed.
- O. Jolliet, M. Margni, R. Charles, S. Humbert, J. Payet, G. Rebitzer and R. Rosenbaum, Impact 2002+: A new life cycle impact assessment methodology, Int. J. Life Cycle Assess., 2003, 8(6), 324–330 CrossRef.
-
Characterisation factors of the ILCD: Recommended life cycle impact assessment methods, JRC-IES, Luxembourg, 2012 Search PubMed.
- P. Fantke, O. Jolliet, J. S. Evans, S. C. Apte, A. J. Cohen, O. O. Hanninen, F. Hurley, M. J. Jantunen, M. Jerrett, J. I. Levy, M. M. Loh, J. D. Marshall, B. G. Miller, P. Preiss, J. V. Spadaro, M. Tainio, J. T. Tuomisto, C. J. Wschler and T. E. McKone, Health effects of fine particulate matter in life cycle impact assessment: findings from the Basel Guidance Workshop, Int. J. Life Cycle Assess, 2015, 20(2), 276–288 CrossRef CAS.
- D. A. Notter, Life cycle impact assessment modeling for particular matter: A new approach based on physico-chemical particle properties, Environ. Int., 2015, 82, 10–20 CrossRef CAS PubMed.
- V. Stone, S. Pozzi-Mucelli, L. Tran, K. Aschberger, S. Sabella, U. Vogel, C. Poland, D. Balharry, T. Fernandes, S. Gottardo, S. Hankin, M. G. J. Hartl, N. Hartmann, D. Hristozov, K. Hund-Rinke, H. Johnston, A. Marcomini, O. Panzer, D. Roncato, A. T. Saber, H. Wallin and J. J. Scott-Fordsmand, ITS-NANO - Prioritising nanosafety research to develop a stakeholder driven intelligent testing strategy, Part. Fibre Toxicol., 2014, 11(9), 1–9, DOI:10.1186/1743-8977-11-9.
- A. A. Keller, S. McFerran, A. Lazareva and S. Suh, Global life cycle releases of engineered nanomaterials, J. Nanopart. Res., 2013, 15, 1–17, DOI:10.1007/s11051-013-1692-4.
- T. Y. Sun, F. Gottschalk, K. Hungerbuhler and B. Nowack, Comprehensive probablistic modelling of environmental emissions of engineered nanomaterials, Environ. Pollut., 2014, 185, 69–76 CrossRef CAS PubMed.
- A. A. Keller and A. Lazareva, Predicted releases of engineered nanomaterials: from global to regional to local, Environ. Sci. Technol. Lett., 2014, 1(1), 65–70 CrossRef CAS.
- Y. Yang, Y. Wang, K. Hristovski and P. Westerhoff, Simultaneous removal of nanosilver and fullerene in sequencing batch reactors for biological wastewater treatment, Chemosphere, 2015, 125(0), 115–121 CrossRef CAS PubMed.
- A. Lazareva and A. A. Keller, Estimating potential life cycle releases of engineered nanomaterials from wastewater treatment plants, ACS Sustainable Chem. Eng., 2014, 2(7), 1656–1665 CrossRef CAS.
- G. A. Sotiriou, D. Singh, F. Zhang, W. Wohlleben, M.-C. G. Chalbot, I. G. Kavouras and P. Demokritou, An integrated methodology for the assessment of environmental health implications during thermal decomposition of nano-enabled products, Environ. Sci.: Nano, 2015, 262–272 RSC.
- A. Keller, W. Vosti, H. Wang and A. Lazareva, Release of engineered nanomaterials from personal care products throughout their life cycle, J. Nanopart. Res., 2014, 16(7), 1–10 CrossRef CAS.
-
Y. Yang and P. Westerhoff, Presence in, and Release of, Nanomaterials from Consumer Products, in Nanomaterial, ed. D. G. Capco and Y. Chen, Springer Netherlands, 2014, vol. 811, pp. 1–17 Search PubMed.
- J. Ging, R. Tejerina-Anton, G. Ramakrishnan, M. Nielsen, K. Murphy, J. M. Gorham, T. Nguyen and A. Orlov, Development of a conceptual framework for evaluation of nanomaterials release from nanocomposites: Environmental and toxicological implications, Sci. Total Environ., 2014, 473–474(0), 9–19 CrossRef CAS PubMed.
- C. Kingston, R. Zepp, A. Andrady, D. Boverhof, R. Fehir, D. Hawkins, J. Roberts, P. Sayre, B. Shelton, Y. Sultan, V. Vejins and W. Wohlleben, Release characteristics of selected carbon nanotube polymer composites, Carbon, 2014, 68(0), 33–57 CrossRef CAS PubMed.
- B. Fiorentino, L. Golanski, A. Guiot, J.-F. Damlencourt and D. Boutry, Influence of paints formulations on nanoparticles release during their life cycle, J. Nanopart. Res., 2015, 17(3), 1–13 CrossRef CAS.
- A. Al-Kattan, A. Wichser, R. Vonbank, S. Brunner, A. Ulrich, S. Zuin, Y. Arroyo, L. Golanski and B. Nowack, Characterization of materials released into water from paint containing nano-SiO2, Chemosphere, 2015, 119(0), 1314–1321 CrossRef CAS PubMed.
- A. E. Nel, W. J. Parak, W. C. W. Chan, T. Xia, M. C. Hersam, C. J. Brinker, J. I. Zink, K. E. Pinkerton, D. R. Baer and P. S. Weiss, Where are we heading in nanotechnology environmental health and safety and materials characterization?, ACS Nano, 2015, 9(6), 5627–5630 CrossRef CAS PubMed.
- R. Hischier, Framework for LCI modelling of releases of manufactured nanomaterials along their life cycle, Int. J. Life Cycle Assess., 2014, 19(4), 838–849 CrossRef CAS.
-
M. A. Huijbregts, M. Z. Hauschild, O. Jolliet, M. Margni, M. McKone, R. K. Rosenbaum and D. van de Meent, USEtox User Manual, 2010 Search PubMed.
- R. K. Rosenbaum, T. Bachmann, L. Gold, M. J. Huijbregts, O. Jolliet, R. Juraske, H. Koehler, M. Larsen, M. MacLeod, M. Margni, T. McKone, J. Payet, M. Schuhmacher and D. van de Meent, USEtox—the UNEP-SETAC toxicity model: recommended characterisation factors for human toxicity and freshwater ecotoxicity in life cycle impact assessment, Int. J. Life Cycle Assess., 2008, 13(7), 532–546 CrossRef CAS.
-
ILCD Handbook: Recommendations for life cycle impact assessment in the European context - based on existing environmental impact assessment models and factors, JRC-IES, Luxemburg, 2011 Search PubMed.
- M. A. J. Huijbregts, U. Thissenb, J. B. Guinéec, T. Jagerd, D. Kalfe, D. van de Meentd, A. M. J. Ragasb, A. Wegener Sleeswijkc and L. Reijndersa, Priority assessment of toxic substances in life cycle assessment. Part I: Calculation of toxicity potentials for 181 substances with the nested multi-media fate, exposure and effects model USES–LCA, Chemosphere, 2000, 41(4), 541–573 CrossRef CAS.
- R. van Zelm, M. Huijbregts and D. van de Meent, USES-LCA 2.0-a global nested multimedia fate, exposure, and effects model, Int. J. Life Cycle Assess., 2009, 14, 282–284 CrossRef.
-
M. Goedkoop, R. Heijungs, M. Huijbregts, A. D. Schryver, J. Struijs and R. van Zelm, ReCiPe 2008: A life cycle impact assessment method which comprises harmonised category indicators at the midpoint and the endpoint level, 2013 Search PubMed.
-
D. Mackay, Multimedia environmental models: The fugacity approach, CRC Press, 2010 Search PubMed.
- G. Cornelis, Fate descriptors for engineered nanoparticles: The good, the bad, the ugly, Environ. Sci.: Nano, 2015, 2, 19–26 RSC.
- A. L. Dale, E. A. Casman, G. V. Lowry, J. R. Lead, E. Viparelli and M. Baalousha, Modeling nanomaterial environmental fate in aquatic systems, Environ. Sci. Technol., 2015, 49, 2587–2593 CrossRef CAS PubMed.
- A. L. Dale, G. V. Lowry and E. A. Casman, Much ado about α: Reframing the debate over appropriate fate descriptors in nanoparticle environmental risk modeling, Environ. Sci.: Nano, 2015, 2, 27–32 RSC.
- A. Praetorius, N. Tufenkji, K.-U. Goss, M. Scheringer, F. von der Kammer and M. Elimelech, The road to nowhere: Equilibrium partition coefficients for nanoparticles, Environ. Sci.: Nano, 2014, 1, 317–323 RSC.
- J. A. J. Meesters, A. A. Koelmans, J. T. K. Quik, A. J. Hendriks and D. van de Meent, Multimedia modeling of engineered nanoparticles with SimpleBox4nano: Model definition and evaluation, Environ. Sci. Technol., 2014, 48, 5726–5736 CrossRef CAS PubMed.
- J. T. K. Quik, J. M. de Klein and A. A. Koelmans, Spatially explicit fate modelling of nanomaterials in natural waters, Water Res., 2015, 80, 200–208 CrossRef CAS PubMed.
- A. Praetorius, M. Scheringer and K. Hungerbuhler, Development of environmental fate models for engineered nanoparticles - A case study of TiO2 nanoparticles in the Rhine River, Environ. Sci. Technol., 2012, 46, 6705–6713 CrossRef CAS PubMed.
- J. T. K. Quik, D. van de Meent and A. A. Koelmans, Simplifying modeling of nanoparticle aggregation-sedimentation behavior in environmental systems: A theoretical analysis, Water Res., 2014, 62, 193–201 CrossRef CAS PubMed.
- M. Scheringer, A. Praetorius and E. S. Goldberg, Chapter 3 - Environmental fate and exposure modeling of nanomaterials, Front. Nanosci., 2014, 7, 89–125 Search PubMed.
- H. H. Liu and Y. Cohen, Multimedia environmental distribution of engineered nanomaterials, Environ. Sci. Technol., 2014, 48, 3281–3292 CrossRef CAS PubMed.
- A. L. Dale, G. V. Lowry and E. A. Casman, Stream dynamics and chemical transformations control the environmental fate of silver and zinc oxide nanoparticles in a watershed-scale model, Environ. Sci. Technol., 2015, 49(12), 7285–7293 CrossRef CAS PubMed.
- G. Rodriguez-Garcia, B. Zimmerman and M. Weil, Nanotoxicity and life cycle assessment: First attempt towards the determination of characterization factors for carbon nanotubes, IOP Conf. Ser.: Mater. Sci. Eng., 2014, 64 DOI:10.1088/1757-899X/64/1/012029.
- B. A. Wender, R. W. Foley, V. Prado-Lopez, D. Ravikumar, D. A. Eisenberg, T. A. Hottle, J. Sadowski, W. P. Flanagan, A. Fisher, L. Laurin, M. E. Bates, I. Linkov, T. P. Seager, M. P. Fraser and D. H. Guston, Illustrating anticipatory life cycle assessment for emerging photovoltaic technologies, Environ. Sci. Technol., 2014, 48, 10531–10538 CrossRef CAS PubMed.
- B. Salieri, S. Righi, A. Pasteris and S. I. Olsen, Freshwater ecotoxicity characterization factor for metal oxide nanoparticles: A case study on titanium dioxide nanoparticle, Sci. Total Environ., 2015, 505, 494–502 CrossRef CAS PubMed.
- R. Grillo, A. H. Rosa and L. F. Fraceto, Engineered nanoparticles and organic matter: A review of the state-of-the-art, Chemosphere, 2015, 119, 608–619 CrossRef CAS PubMed.
- L. E. Barton, M. Auffman, L. Olivi, J. Y. Bottero and M. R. Wiesner, Heteroaggregation, transformation and fate of CeO2 nanoparticles in wastewater treatment, Environ. Pollut., 2015, 203, 122–129 CrossRef CAS PubMed.
- R. K. Rosenbaum, M. A. J. Huijbregts, A. D. Henderson, M. Margni, T. E. McKone, D. van de Meent, M. Z. Hauschild, S. Shaked, D. S. Li, L. S. Gold and O. Jolliet, USEtox human exposure and toxicity factors for comparative assessment of toxic emissions in life cycle analysis: Sensitivity to key chemical properties, Int. J. Life Cycle Assess., 2011, 16(8), 710–727 CrossRef CAS.
- A. D. Henderson, M. Z. Hauschild, D. van de Meent, M. A. J. Huijbregts, H. F. Larsen, M. Margni, T. E. McKone, J. Payet, R. K. Rosenbaum and O. Jolliet, USEtox fate and ecotoxicity factors for comparative assessment of toxic emissions in life cycle analysis: sensitivity to key chemical properties, Int. J. Life Cycle Assess., 2011, 16(8), 701–709 CrossRef CAS.
- J. M. Cohen, J. G. Teeguarden and P. Demokritou, An integrated approach for the in vitro dosimetry of engineered nanomaterials, Part. Fibre Toxicol., 2014, 11, 20 CrossRef PubMed.
- R. A. Khanbeigi, A. Kumar, F. Sadouki, C. Lorenz, B. Forbes, L. Dailey and H. Collins, The delivered dose: Applying particokinetics to in vitro investigations of nanoparticle internalization by macrophages, J. Controlled Release, 2012, 162(2), 259–266 CrossRef PubMed.
- A. K. Pal, D. Bello, J. M. Cohen and P. Demokritou, Implications of in vitro dosimetry on toxicological ranking of low aspect ratio engineered nanomaterials, Nanotoxicology, 2015, 1–15, DOI:10.3109/17435390.2014.986670.
- A. K. Pal, C. Watson, S. V. Pirela, D. Singh, M.-C. G. Chalbot, I. G. Kavouras and P. Demokritou, Linking exposure of particles released from nano-enabled products to toxicology: An integrated methodology for particle sampling, extraction, dispersion and dosing, Toxicol. Sci., 2015 DOI:10.1093/toxsci/kfv095.
- C. Bianco, S. Kezic, M. J. Visser, O. Pluut, G. Adami and P. Krystek, Pilot study on the identification of silver in skin layers and urine after dermal exposure to functionalized textile, Talanta, 2015, 136, 23–28 CrossRef CAS PubMed.
- Y. Nazarenko, P. J. Lioy and G. Mainelis, Quantitative assessment of inhalation exposure and deposited dose of aerosol from nanotechnology-based consumer sprays, Environ. Sci.: Nano, 2014, 1, 161–171 RSC.
- N. von Goetz, C. Lorenz, L. Windler, B. Nowack, M. Heuberger and K. Hungerbuhler, Migration of Ag- and TiO2-(nano)particles from textiles into artificial sweat under physical stress: Experiments and exposure modeling, Environ. Sci. Technol., 2013, 47, 9979–9987 CrossRef CAS PubMed.
- C. Bekker, E. Kuijpers, D. H. Brouwer, R. Vermeulen and W. Fransman, Occupational exposure to nano-objects and their agglomerates and aggregates across various life cycle stages: A broad-scale exposure study, Ann. Occup. Hyg., 2015, 681–704 CrossRef PubMed.
- T. Walser, D. Meyer, W. Fransman, H. Buist, E. Kuijpers and D. Brouwer, Life-cycle assessment framework for indoor emissions of synthetic nanoparticles, J. Nanopart. Res., 2015, 17(245) DOI:10.1007/s11051-015-3053-y.
- D. Bello, B. L. Wardle, N. Yamamoto, R. G. deVilloria, E. J. Garcia, A. J. Hart, K. Ahn, M. J. Ellenbecker and M. Hallock, Exposure to nanoscale particles and fibers during machining of hybrid advanced composites containing carbon nanotubes, J. Nanopart. Res., 2009, 11(1), 231–249 CrossRef CAS.
- S. A. Brenner, N. M. Neu-Baker, C. Caglayan and I. G. Zurbenko, Occupational Exposure to Aerosolized Metal Oxide Nanoparticles in Semiconductor Wastewater Treatment, J. Occup. Environ. Hyg., 2015, 12(7), 469–481 CrossRef CAS PubMed.
- K. A. Scanlon, S. M. Lloyd, G. M. Gray, R. A. Francis and P. LaPuma, An approach to integrating occupational safety and health into life cycle assessment, J. Ind.
Ecol., 2014, 19(1), 27–37 CrossRef PubMed.
- S. Hellweg, E. Demou, R. Bruzzi, A. Meijer, R. K. Rosenbaum, M. A. J. Huijbregts and T. E. McKone, Integrating human indoor air pollutant exposure within life cycle impact assessment, Environ. Sci. Technol., 2009, 43(6), 1670–1679 CrossRef CAS.
- R. V. van Zelm, M. A. J. Huijbregts, J. V. Harbers, A. Wintersen, J. Struijs, L. Posthuma and D. Meent, Uncertainty in msPAF-based ecotoxicological freshwater effect factors for chemicals with a non-specific mode of action in life cycle impact assessment, Integr. Environ. Assess. Manage., 2007, 3(2), 203–210 CrossRef CAS PubMed.
- L. Golsteijn, H. W. Hendriks, R. van Zelm, A. M. Ragas and M. A. Huijbregts, Do interspecies correlation estimations increase the reliability of toxicity estimates for wildlife?, Ecotoxicol. Environ. Saf., 2012, 80, 238–243 CrossRef CAS PubMed.
- J. Kostal, A. Voutchkova-Kostal, P. T. Anastas and J. B. Zimmerman, Identifying and designing chemicals with minimal acute aquatic toxicity, Proc. Natl. Acad. Sci. U. S. A., 2015, 112(20), 6289–6294 CrossRef CAS PubMed.
- C. Rovida and T. Hartung, Re-evaluation of animal numbers and costs for in vivo test to accomplish REACH legislation requirements for chemical - a report by the transatlantic think tank for toxicology, Altex, 2009, 26(3), 187 Search PubMed.
- A. E. Nel, Implementation of alternative test strategies for the safety assessment of engineered nanomaterials, J. Intern. Med., 2013, 274, 561–577 CrossRef CAS PubMed.
- Z. A. Collier, A. J. Kennedy, A. R. Poda, M. F. Cuddy, R. D. Moser, R. I. MacCuspie, A. Harmon, K. Plourde, C. D. Haines and J. A. Steevens, Tiered guidance for risk-informed environmental health and safety testing of nanotechnologies, J. Nanopart. Res., 2015, 17 Search PubMed.
- D. van de Meent and M. A. J. Huijbregts, Calculating life-cycle assessment effect factors from potentially affected fraction-based ecotoxicological response functions, Environ. Toxicol. Chem., 2005, 24(6), 1573–1578 CrossRef CAS PubMed.
- R. van Zelm, M. A. J. Huijbregts, J. V. Harbers, A. Wintersen, J. Struijs, L. Posthuma and D. van de Meent, Uncertainty in msPAF-based ecotoxicological effect factors for freshwater ecosystems in life cycle impact assessment, Integr. Environ. Assess. Manage., 2007, 3, 203–210 CrossRef CAS PubMed.
- R. van Zelm, M. A. J. Huijbregts, L. Posthuma, A. Wintersen and D. van de Meent, Pesticide ecotoxicological effect factors and their uncertainties for freshwater ecosystems, Int. J. Life Cycle Assess., 2009, 14, 43–51 CrossRef.
- G. DeLoid, J. M. Cohen, T. Darrah, R. Derk, L. Rojanasakul, G. Pyrgiotakis, W. Wohlleben and P. Demokritou, Estimating the effective density of engineered nanomaterials for in vitro dosimetry, Nat. Commun., 2014, 5 CAS.
- C. J. E. Delmaar, W. J. G. M. Peijnenburg, A. G. Oomen, J. Chen, W. H. de Jong, A. J. A. M. Sips, Z. Wang and M. V. D. Z. Park, A practical approach to determine dose metrics for nanomaterials, Environ. Toxicol. Chem., 2015, 34(5), 1015–1022 CrossRef CAS PubMed.
- M. Hull, A. J. Kennedy, C. Detzel, P. J. Vikesland and M. A. Chappell, Moving beyond mass: The unmet need to consider dose metrics in environmental nanotoxicology studies, Environ. Sci. Technol., 2012, 46, 10881–10882 CrossRef CAS PubMed.
- L. Pourzahdei and M. J. Eckelman, Comparative life cycle assessment of silver nanoparticle synthesis routes, Environ. Sci.: Nano, 2015, 361–369 Search PubMed.
- A. Casey, M. Davoren, E. Herzog, F. M. Lyng, H. J. Byrne and G. Chambers, Probing the interaction of single walled carbon nanotubes within cell culture medium as a precursor to toxicity testing, Carbon, 2007, 45, 34–40 CrossRef CAS PubMed.
- A. Casey, E. Herzog, M. Davoren, F. M. Lyng, H. J. Byrne and G. Chambers, Spectroscopic analysis confirms the interactions between single walled carbon nanotubes and various dyes commonly used to assess cytotoxicity, Carbon, 2007, 45, 1425–1432 CrossRef CAS PubMed.
- A. Nel, T. Xia, H. Meng, X. Wang, S. Lin, Z. Ji and H. Zhang, Nanomaterial toxicity testing in the 21st century: Use of a predictive toxicological approach and high-throughput screening, Acc. Chem. Res., 2013, 46(3), 607–621 CrossRef CAS PubMed.
- S. L. Harper, J. L. Carriere, J. M. Miller, J. E. Hutchison, B. L. S. Maddux and R. L. Tanguay, Systematic evaluation of nanomaterial toxicity: Utility of standardized materials and rapid assays, ACS Nano, 2011, 5(6), 4688–4697 CrossRef CAS PubMed.
- R. Liu, H. Y. Zhang, Z. X. Ji, R. Rallo, T. Xia, C. H. Chang, A. Nel and Y. Cohen, Development of structure-activity relationship for metal oxide nanoparticles, Nanoscale, 2013, 5, 5644 RSC.
- S. George, T. Xia, R. Rallo, Y. Zhao, Z. Ji, S. Lin, X. Wang, H. Zhang, B. France, D. Schoenfeld, R. Damoiseaux, R. Liu, S. Lin, K. A. Bradley, Y. Cohen and A. E. Nel, Use of a high throughput screening approach coupled with in vivo zebrafish embryo screening to develop hazard ranking for engineered nanomaterials, ACS Nano, 2011, 5(3), 1805–1817 CrossRef CAS PubMed.
- D. M. Mitrano, S. Motellier, S. Clavaguera and B. Nowack, Review of nanomaterial aging and transformations through the life cycle of nano-enhanced products, Environ. Int., 2015, 77, 132–147 CrossRef CAS PubMed.
- B. Reidy, A. Haase, A. Luch, K. A. Dawson and I. Lynch, Mechanisms of silver nanoparticle release, transformation and toxicity: A critical review of current knowledge and recommendations for future studies and applications, Materials, 2013, 6, 2295–2350 CrossRef CAS PubMed.
- B. Nowack, J. F. Ranville, S. Diamond, J. A. Gallego-Urrea, C. Metcalfe, J. Rose, N. Horne, A. A. Koelmans and S. J. Klaine, Potential scenarios for nanomaterial releasae and subsequent alteration in the environment, Environ. Toxicol. Chem., 2012, 31(1), 50–59 CrossRef CAS PubMed.
- J. L. Bitter, J. Yang, S. B. Milani, C. T. Jafvert and H. D. Fairbrother, Transformations of oxidized multiwalled carbon nanotubes exposed to UVC (254 nm) irradiation, Environ. Sci.: Nano, 2014, 1, 324–337 RSC.
- W.-C. Hou, I. Chowdhury, D. G. Goodwin, M. W. Henderson, H. D. Fairbrother, D. Bouchard and R. G. Zepp, Photochemical transformation of Graphene Oxide in Sunlight, Environ. Sci. Technol., 2015, 49(6), 3435–3443 CrossRef CAS PubMed.
- G. V. Lowry, K. B. Gregory, S. C. Apte and J. R. Lead, Transformations of nanomaterials in the environment, Environ. Sci. Technol., 2012, 46, 6893–6899 CrossRef CAS PubMed.
- R. van Zelm, M. A. J. Huijbregts and D. van de Meent, Transformation products in the life cycle impact assessment of chemicals, Environ. Sci. Technol., 2010, 44(3), 1004–1009 CrossRef CAS PubMed.
- S. K. Misra, A. Dybowska, D. Berhanu, S. N. Luoma and E. Valsami-Jones, The complexity of nanoparticle dissolution and its importance in nanotoxicological studies, Sci. Total Environ., 2012, 438, 225–232 CrossRef CAS PubMed.
- D. A. Notter, D. M. Mitrano and B. Nowack, Are nanosized or dissolved metals more toxic in the environment? A meta-analysis, Environ. Toxicol. Chem., 2014, 33(12), 2733–2739 CrossRef CAS PubMed.
- T. Walser, E. Demou, D. J. Lang and S. Hellweg, Prospective environmental life cycle assessment of nanosilver t-shirts, Environ. Sci. Technol., 2011, 45(10), 4570–4578 CrossRef CAS PubMed.
- G. E. Batley, J. K. Kirby and M. J. McLaughlin, Fate and risks of nanomaterials in aquatic and terrestrial environments, Acc. Chem. Res., 2013, 46(3), 854–862 CrossRef CAS PubMed.
-
Scientific, Technical, Research, Engineering and Modeling Support Final Report: State of the Science Literature Review: Everything Nanosilver and More, U.S. EPA Office of Research and Development, Washington, DC, 2010 Search PubMed.
- K. Garner and A. Keller, Emerging patterns for engineered nanomaterials in the environment: a review of fate and toxicity studies, J. Nanopart. Res., 2014, 16(8), 1–28 CrossRef.
- A. Ivask, K. Juganson, O. Bondarenko, M. Mortimer, V. Aruoja, K. Kasemets, I. Blinova, M. Heinlaan, V. Slaveykova and A. Kahru, Mechanisms of toxic action of Ag, ZnO, and CuO nanoparticles to selected ecotoxicological test organisms and mammalian cells in vitro: A comparative review, Nanotoxicology, 2014, 8, 57–71 CrossRef CAS PubMed.
- Y. Liu, Y. Zhao, B. Sun and C. Chen, Understanding the toxicity of carbon nanotubes, Acc. Chem. Res., 2013, 46(3), 702–713 CrossRef CAS PubMed.
- M. F. L. De Volder, S. H. Tawfick, R. H. Baughman and A. J. Hart, Carbon nanotubes: Present and future commercial applications, Science, 339, 535–539 CrossRef CAS PubMed.
- V. Castranova, P. A. Schulte and R. D. Zumwalde, Occupational nanosafety considerations for carbon nanotubes and carbon nanofibers, Acc. Chem. Res., 46(3), 642–649 CrossRef CAS PubMed.
-
Scientific, Technical, Research, Engineering and Modeling Support (STREAMS) Final Report: State of the Science Literature Review: Nano Titanium Dioxide Environmental Matters, U.S. EPA Office of Research and Development, Washington, DC, 2010 Search PubMed.
- Y. Ge, J. P. Schimel and P. A. Holden, Evidence for negative effects of TiO2 and ZnO nanoparticles on soil bacterial communities, Environ. Sci. Technol., 2011, 45(4), 1659–1664 CrossRef CAS PubMed.
- R. J. Vandebriel and W. H. de Jong, A review of mammalian toxicity of ZnO nanoparticles, Nanotechnol., Sci. Appl., 2012, 5, 61–71 CrossRef CAS PubMed.
- D. Andreescu, G. Bulbul, R. E. Ozel, A. Hayat, N. Sardesai and S. Andreescu, Applications and implications of nanoceria reactivity: measurement tools and environmental impact, Environ. Sci.: Nano, 2014, 1, 445–458 RSC.
- B. Collin, M. Auffan, A. C. Johnson, I. Kaur, A. A. Keller, A. Lazareva, J. R. Lead, X. Ma, R. C. Merrifield, C. Svendsen, J. C. White and J. M. Unrine, Environmental release, fate and ecotoxicological effects of manufactured ceria nanomaterials, Environ. Sci.: Nano, 2014, 1, 533–548 RSC.
- K. Reed, A. Cormack, A. Kulkarni, M. Mayton, D. Sayle, F. Klaessig and B. Stadler, Exploring the properties and applications of nanoceria: is there still plenty of room at the bottom?, Environ. Sci.: Nano, 2014, 1, 390–405 RSC.
- R. A. Yokel, S. Hussain, S. Garantziotis, P. Demokritou, V. Castranova and F. R. Cassee, The yin: an adverse health perspective of nanoceria: uptake, distribution, accumulation, and mechanisms of its toxicity, Environ. Sci.: Nano, 2014, 1, 406–428 RSC.
- F. Piccinno, F. Gottschalk, S. Seeger and B. Nowack, Industrial production quantities and uses of ten engineered nanomaterials in Europe and the world, J. Nanopart. Res., 2012, 14, 1–11, DOI:10.1007/s11051-012-1109-9.
- F. Gottschalk, T. Y. Sun and B. Nowack, Environmental concentrations of engineered nanomaterials: Review of modeling and analytical studies, Environ. Pollut., 2013, 181, 287–300 CrossRef CAS PubMed.
- The Project of Emerging Nanotechnologies: Consumer Products Inventory. http://www.nanotechproject.org/cpi/ (Accessed March 15, 2015).
Footnote |
† Contributed equally to this work. |
|
This journal is © The Royal Society of Chemistry 2015 |
Click here to see how this site uses Cookies. View our privacy policy here.