Transforming organic-rich amaranthus waste into nitrogen-doped carbon with superior performance of the oxygen reduction reaction†
Received
7th July 2014
, Accepted 5th September 2014
First published on 8th September 2014
Abstract
We present a cost-effective approach to dispose of amaranthus waste (the discarded leaves and stalks of amaranthus and the extract remains of natural amaranthus red) to yield nitrogen-doped carbon. Amaranthus waste is a natural, abundantly available, and yearly renewable source, acting as a single precursor for nitrogen (mainly from the lysine-rich amino acids) as well as carbon. It therefore eliminates the need for multiple hazardous chemicals including organic precursors for similar synthesis processes. Our facile experimental strategy without any activation supports reasonable nitrogen doping in porous carbon along with a high surface area and excellent conductivity, which leads to a superior electrocatalytic oxygen reduction activity and proves to be a promising alternative for costly Pt-based electrocatalysts in fuel cells in terms of excellent electrocatalytic performance, high selectivity, and long durability. This judicious transformation of organic-rich waste not only addresses the disposal issue, but also generates valuable functional carbon materials from the discard. Our as-synthesized carbon will certainly be believed to be a trend setter and have greater economic ramifications by creating value-added materials from waste.
Broader context
In the scarcity of fossil energy, renewable energy technologies based on sustainable biomass will necessarily grow in importance to meet our future energy demands because of their potential in reducing our reliance on such limited resources. Electrochemical devices such as fuel cells and metal–air batteries are anticipated to be used for automotive applications in the future. For this to happen, the precious noble metal (or noble metal-containing) electrocatalysts used to overcome the sluggish kinetics of the oxygen reduction reaction (ORR) at the cathode should be replaced by low-cost, earth-abundant substitutes that exhibit comparable catalytic activity and long-term stability. We present a cost-effective approach to yield nitrogen-doped carbons from amaranthus waste, which is a natural, abundantly available, and yearly renewable source, acting as a single precursor for nitrogen (mainly from the lysine-rich amino acids) as well as carbon. This study presents that highly effective waste-derived nitrogen-doped carbon materials with a high ORR electrocatalyst can be achieved by a safe and low-cost method. In addition, the application of organic-rich amaranthus waste as the heteroatom doping sources will be readily adaptable for other electrode materials applied to various battery systems.
|
Introduction
The increasing energy demand of our society has stimulated a worldwide search for alternative energy sources to fossil fuels in recent years. Electrochemical devices such as fuel cells and metal–air batteries are anticipated to be used for automotive applications in the future.1,2 However, due to the sluggish kinetics of the oxygen reduction reaction (ORR) at the cathode, more efficient electrocatalysts have been extensively studied and are still highly desired in these fields.3–5 Until now, Pt and Pt-based electrocatalysts have dominated as the best catalytic materials for the ORR,6 although the high cost, poor long-term stability, and scarcity of Pt prove to be obstructions to their commercialization.7–10 As such, the exploration of highly active non-platinum catalysts for the four-electron (4e−) ORR with comparable electrochemical performance is of prime importance to optimize the performance of fuel cells.11–14
Recently, nitrogen (N)-doped carbon (NDC) materials have been of particular interest as metal-free electrocatalysts for the ORR in an alkaline environment.11,15–22 The enhanced electrocatalytic performance could be attributed to the doped heteroatoms into sp2-hybridized carbon frameworks, which favors the adsorption and reduction of oxygen and improves the long-time operation and practical durability.23–26 Carbon doped with single,27 dual,28 or multiple heteroatoms into its framework has shown efficacy as a metal-free electrocatalyst in several studies.29 In recent developments, research on doped carbon materials has moved from expensive, often hazardous inorganic and organic chemicals to renewable biomass as precursors, mainly for sustainable consideration.30–32 Apart from edible biomass, organic-rich waste also has tremendous potential to generate graphitic carbons.33 Some efforts have been realized in this direction through the synthesis of high surface area carbons from crab shells34 and pig bones.35 N-doped carbon materials were prepared from egg-shell membranes36 and prawn shells,37 and applied as efficient electrode materials in supercapacitors or fuel cells. Taking a step further, highly conductive carbons with reasonable dual-doping of heteroatoms (N and S) and a high surface area were generated from hair and used as a metal-free catalyst for the ORR, thus emphasizing the untapped potential in organic-rich waste.38
As for metal-free nitrogen-modified carbon-based catalysts, nitrogen functional groups doped in the carbon matrix are believed to be active for the ORR (more exactly, pyrrolic-N, pyridinic-N and quaternary-N are the ORR active sites). The nitrogen-induced charge delocalization could facilitate the bridge model chemisorption of O2 on the N-doped carbon catalysts, which could effectively weaken the O–O bonding to facilitate the ORR. It should be pointed out that the selectivity of oxygen reduction is very important, that is the 4 electron reaction or 2 electron reaction. As the Popov's example, non-nitrogen doped carbon generated about 80% H2O and 20% H2O2, and nitrogen-modified carbon generated above 96% H2O and less than 4% H2O2, which means that modification of N-containing groups on carbon not only increases the ORR activity, but also improves the selectivity towards oxygen reduction.39,40
Amaranth is cultivated in Africa, India, China, Nepal, and other tropical countries. In a 1977 article in Science, amaranth was described as “the crop of the future”.41 Amaranthus contains around 14% protein, which comes mainly from the lysine-rich amino acids.42 Such an essential amino acid, lysine, is limited in other grains or plant sources.43,44 Natural amaranthus red is a much-touted natural edible dye extracted from the flowers and leaves of amaranthus. The discarded leaves and stalks of amaranthus and the extract remains of natural amaranthus red are abundantly available organic-rich wastes that remain largely unexplored for their potential to generate heteroatom-doped carbon. Hence, we decided to use them as sources for synthesizing NDC. The use of the discarded leaves, stalks of amaranthus, and the extract remains of natural amaranthus red as precursor materials for NDC is very new.45 A facile synthesis approach without any activation is explored in order to simplify the procedure and lower the cost. There are several carbon examples generated for natural leaves reported in the literature,46–63 however, to the best of our knowledge, this is a rare report on cost-effective fabrication of NDC without activation from biomass waste and its application as a metal-free catalyst for the ORR. Creating high-valued carbon materials from the discarded amaranth directly addresses its disposal issue and contributes to the environmental remediation, emphasizing the significance of our work in sustainable energy and proving that NDCs can be a promising alternative for costly Pt-based electrocatalysts.
Experimental section
Preparation/synthesis of NDCs
For the synthesis of NDCs, 10 g of thoroughly dried amaranthus waste was heated in an alumina crucible at 600, 700, 800, and 900 °C under a N2 atmosphere for 2 h to generate a carbonized material. After cooling, the black powder was treated with 2.0 M HCl and hot deionized water to remove metal components, and dried at 40 °C overnight. The generated carbons were labeled as NDC-X-Y, where X represents the kinds of amaranthus waste (L is the leaf, S is the stalk, and E is the extract remains), and Y stands for the annealing temperature.
Preparation of the working electrodes
The glassy carbon (GC) electrode was polished to a mirror finish using alumina powder. After that, the electrodes were sonicated in DI water for 30 min and washed several times with DI water and ethanol to remove any bound particles, and dried under a gentle air stream. Then, catalyst ink prepared by dispersing the as-prepared catalysts in DI water with 5 wt% Nafion® solution was drop-coated on the polished GC electrode surface. This electrode was then dried under ambient conditions for electrochemical measurements. By using the same electrode configuration, the commercial 20% Pt/C catalyst with the same amount was also studied for comparison.
Characterization
The size and morphology of all the samples were visualized using electron microscopy facilities (a Hitachi S-4700 microscope operating at an acceleration voltage of 10 kV and a JEOL FE-2010 microscope operating at 200 kV). Nitrogen adsorption–desorption isotherms were measured at 77 K using an ASAP 2020-Physisorption Analyzer (Micromeritics, USA) where the BET method was used for surface area determination. Before performing the measurements, all the samples were outgassed under vacuum at 350 °C for 12 h until the pressure was less than 5 × 10−3 Torr. X-ray diffraction (XRD) patterns were recorded with a Bruker-D8 apparatus with Cu radiation at a scan rate of 2° min−1. The Raman spectra were recorded with a Renishaw inVia unit using an Ar ion laser with an excitation wavelength of 514.5 nm. X-ray photoelectron spectroscopy (XPS) was conducted with a VG Microtech ESCA 2000 using a monochromic Al X-ray source.
Electrochemical measurements
Electrochemical experiments were conducted at room temperature on a CHI660D electrochemical workstation (CH instrument Co.) with a three-electrode cell equipped with gas-flow systems at 25 °C. The glassy carbon electrode (GCE) and RD-GCE were the working electrodes and a piece of Pt foil (1 cm2) served as the counter electrode. The Hg/HgO reference electrode (0.098 V relative to the reversible hydrogen electrode, RHE) was placed in a separate chamber, which was located near the working electrode chamber through a Luggin capillary tube. For ORR measurements, the electrolyte was pre-saturated with O2 by purging pure O2 for 30 min before the experimental measurements. RDE linear sweep voltammetry was performed in O2-saturated 0.1 M KOH solution at a scan rate of 10 mV s−1 from −0.8 V to +0.4 V at different rotation speeds from 400–2025 rpm. The methanol tolerance test was performed in O2-saturated 0.1 M KOH in the presence and absence of 3.0 M methanol. The potential was scanned between −0.8 V to +0.2 V at a scan rate of 10 mV s−1, at room temperature. The stability of the catalyst was evaluated by the CVs in O2-saturated 0.1 M KOH for 1000 cycles. Fresh electrolyte solution was used for each electrochemical measurement to ensure reproducible results. All the potentials reported herein were versus the Hg/HgO electrode.
The kinetic parameters can be analyzed using the Koutecky–Levich (K–L) equation:64
where
J is the measured current density,
Jk and
JL are the kinetic and diffusion limiting current densities, respectively,
n is the number of electrons transferred per oxygen molecule,
F is the Faraday constant,
CO is the bulk concentration of O
2 dissolved in the electrolyte (1.2 × 10
−3 mol L
−1),
DO is the diffusion coefficient of O
2 in the NaOH electrolyte (1.9 × 10
−5 cm
−2 s),
υ is the kinetic viscosity of the electrolyte (1.0 × 10
−2 cm
−2 s),
ω is the rotation rate of the electrode, and
k is the electron transfer rate constant. According to the K–L equation, the number of electrons transferred could be calculated from the slope of the K–L plots.
Results and discussion
Preparation of NDCs was accomplished by pyrolysis of the amaranthus waste under a nitrogen atmosphere and washing with HCl (Scheme 1). The generated carbons were labeled as NDC-X-Y, where X represents the kinds of amaranthus waste (L is the leaf, S is the stalk, and E is the extract remains), and Y stands for the annealing temperature. Take NDC-L-Y as an example to display the results. XPS analysis was carried out to investigate the elemental composition and the percentage of N-incorporation. C 1s, O 1s, and N 1s are observed in the full survey XPS spectra of NDC-L-Y (Fig. 1A). The peak corresponding to C 1s of NDC-L-800 can be adequately deconvoluted into four components with binding energies of 284.4, 285.2, 286.1 and 289.1 eV and relative proportions of 45%, 28%, 21% and 6%, respectively, attributable to graphitic sp2 carbon, amorphous carbon, sp2 carbon atoms bonded to nitrogen and sp2 carbon atoms bonded to oxygen, respectively (Fig. 1B). Fig. S1† displays the C 1s peaks of other NDC-L-Y samples. We have also observed the presence of the N 1s peak, suggesting successful incorporation of N into the resulting carbons. The N contents for all the carbons were in the range of 1.42–2.47 at%, with the maximum content found in NDC-L-700. The annealing temperatures did highly affect the overall N content, whereas NDC-L-800 and NDC-L-900 lost about two thirds of the original N content compared to NDC-L-700 (Table 1). The chemical status of N in the doped carbons was further probed by analyzing the high resolution N 1s peaks. The deconvoluted N 1s photoelectron envelopes are shown in Fig. 1C and S2.† They show three different peaks revealing the presence of pyridinic N1 (398.40 ± 0.2), pyrrolic N2 (399.9 ± 0.2), quaternary or graphitic N3 (401.2 eV ± 0.2).28,44 Interestingly, the relative intensities of all three peaks change with increasing annealing temperature, indicating the difference in temperature dependency of the N-bonding configurations. With increasing annealing temperatures, the N2 peak basically remains, and the N3 peak becomes dominant, suggesting the formation of more graphitic N with increasing temperature. The N1 content in the NDC-L-800 and NDC-L-900 decreased largely, possibly due to its lower stability at higher temperatures.65 Overall, the XPS analysis suggests that the lysine, which is made of basic C and N, has been successfully transformed into a carbon framework with N doping. A complete tabular overview showing the respective peak assignments and binding energies of N and C for all the carbons is provided in Table 1.
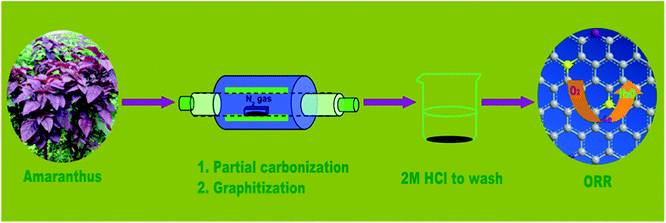 |
| Scheme 1 Schematic procedure for the fabrication of N-doped, high surface area carbon from amaranthus waste. | |
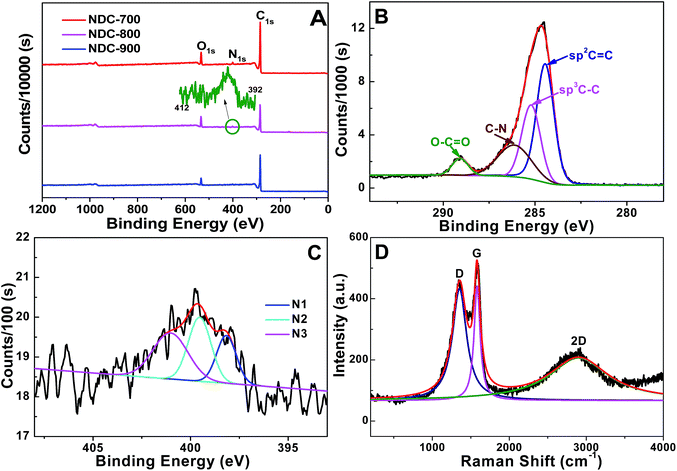 |
| Fig. 1 (A) Full-scan XPS of NDC-L-700, NDC-L-800, and NDC-L-900. (B and C) C 1s and high resolution N 1s XPS scan of NDC-L-800. (D) Raman spectrum of NDC-L-800. | |
Table 1 Summary of textural parameters obtained from nitrogen adsorption analysis and chemical composition analysis from XPS and electrochemical properties of samples
Sample |
S
BET (m−2 g−1) |
Total pore volumes (cm3 g−1) |
Micropore volumes (cm3 g−1) |
At% (C) |
At% (N) |
At% (O) |
N1 (%) |
N2 (%) |
N3 (%) |
Onset potential |
Half-wave potential |
NDC-L-600 |
662.6393 |
0.4981 |
0.1357 |
82.69 |
4.05 |
13.26 |
42.4 |
31.7 |
25.9 |
0.24 |
−0.08 |
NDC-L-700 |
949.0234 |
0.6760 |
0.4189 |
87.72 |
2.47 |
9.58 |
31.1 |
34.8 |
34.1 |
0.25 |
−0.02 |
NDC-L-800 |
988.4265 |
0.7433 |
0.4327 |
83.38 |
1.63 |
12.99 |
23.6 |
36.1 |
40.3 |
0.27 |
0 |
NDC-L-900 |
1008.4305 |
0.7950 |
0.4241 |
90.94 |
1.42 |
7.39 |
7.5 |
40.8 |
51.7 |
0.23 |
0.02 |
In contrast to the information provided by XPS, the Raman spectra of all the samples were almost coincident (Fig. 1D and S3†), exhibiting the characteristic “G” and “D” peaks at 1580 and 1380 cm−1, respectively. No significant shifts in the position of the “G” and “D” peaks as a function of the pyrolysis temperature were observed. In general, the D-band being located at 1350 cm−1 relates to a zone-edge A1g mode, because of the disorder in graphitic structures.66 A high disorder in carbon leads to broadening of both the G and D bands with a higher relative intensity of the D-band compared to that of the G-band.67 However, it should be noted that in a related precedent describing the Raman spectra of NDCs,68 observation of an intense and broad “D” band, as recorded also in the present case, was attributed to the presence of N atoms in the graphene layers acting as defect sites.69 The formation of graphitic phases with temperature and the amount of disorder introduced by heteroatom N doping were also validated by studying the XRD patterns. Fig. 2A shows wide-angle XRD patterns of the four NDCs with two broad peaks centered at 23.6° and 43.8°, corresponding to the (002) and (100) lattice planes of a typical turbostratic carbon. These suggest the presence of graphitic carbon.70 As is well known, the carbon graphitization in the catalysts can enhance the electronic conductivity and corrosion resistance during electrocatalysis.71 The sample has a conductivity of 0.492 S cm−1, which is a fairly good value. The interlayer spacing between the stacks of thin carbon sheets is higher in turbostratic carbon than in graphite, along with a higher degree of disorder. Therefore, the XPS, Raman, and XRD results support the finding that the doped N heteroatom causes increased defects in the carbon structure.
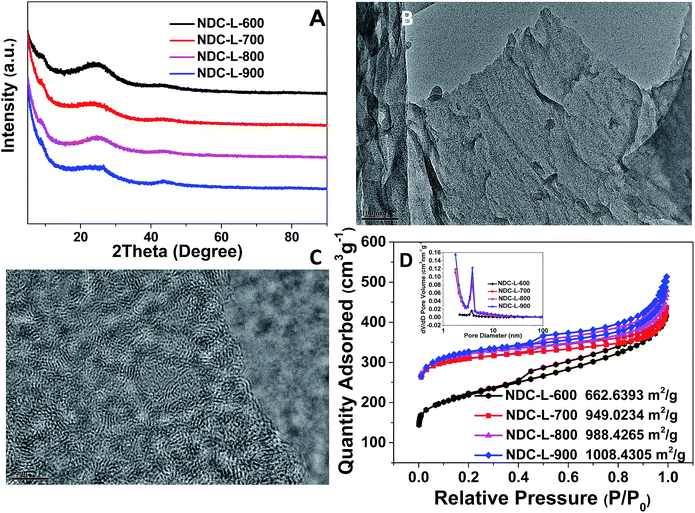 |
| Fig. 2 (A) XRD pattern over the 2θ range of 5–90° of NDC-L-600, NDC-L-700, NDC-L-800, and NDC-L-900. (B) TEM and (C) HRTEM images of NDC-L-800. (D) N2 adsorption–desorption isotherms of NDC-L-700, NDC-L-800, and NDC-L-900. The inset is pore-size distributions (PSDs) of the four samples. | |
The morphology of the as-prepared carbons was investigated by transmission electron microscopy (TEM) and high-resolution transmission electron microscopy (HR-TEM). Activated carbons are normally composed of randomly stacked graphite-like planes with a high level of disorder, which results in a high porosity and surface area. Thin stacks of disordered carbon layers were often seen in the high-temperature annealed NDC-L-800 sample, as shown in Fig. 2B and C. The surface area is an important feature of doped carbon materials in context to their electrochemical activity. Fig. 2D shows the nitrogen adsorption–desorption isotherms and pore-size distribution (PSD) of the four samples. All the carbons showed typical type-I isotherms, indicating their microporous nature. The PSD calculated using the Barrett–Joyner–Halenda (BJH) method indicates the prevalence of micropores and small mesopores ranging from 0.5 to 4.0 nm in size with surface areas of 949.02, 988.43, and 1008.43 m2 g−1 for NDC-L-700, NDC-L-800, and NDC-L-900, respectively. The impact of the annealing temperature on the surface area and the heteroatom content is displayed in Table 1. It can be inferred that our experimental protocol preserves the high surface area without losing the valuable heteroatoms essential for electrocatalytic activity. Therefore, the above characterization data support the formation of NDCs from the discarded amaranthus leaves as a single source of carbon and nitrogen atoms. Considering that the discarded amaranthus leaves come from the main waste of agriculture, the synthesis of NDC constitutes a remarkable example of biomass valorization.
The as-synthesized NDCs were applied as electrocatalysts for the ORR and the electrocatalytic performance was analyzed using cyclic voltammetry (CV) and rotating disk electrode (RDE) experiments. CV experiments for NDC-L-Y were performed in 0.1 M KOH solution saturated with N2 or O2 at the scan rate of 10 mV s−1. A quasi-rectangular voltammogram without an obvious redox peak was observed for all the four samples when the electrolyte was saturated with N2. In contrast, upon introduction of O2 into the electrolyte, a well-defined ORR peak was observed, implying the sensitivity of NDCs towards oxygen reduction as shown in Fig. 3A. In terms of peak potential, NDC-L-800 showed a more positive shift (−0.09 V) followed by NDC-L-700 (−0.10 V), NDC-L-900 (−0.12 V), and NDC-L-600 (−0.14 V), indicating highly pronounced ORR activity by NDC-L-800 compared to its high-temperature annealed counterparts.
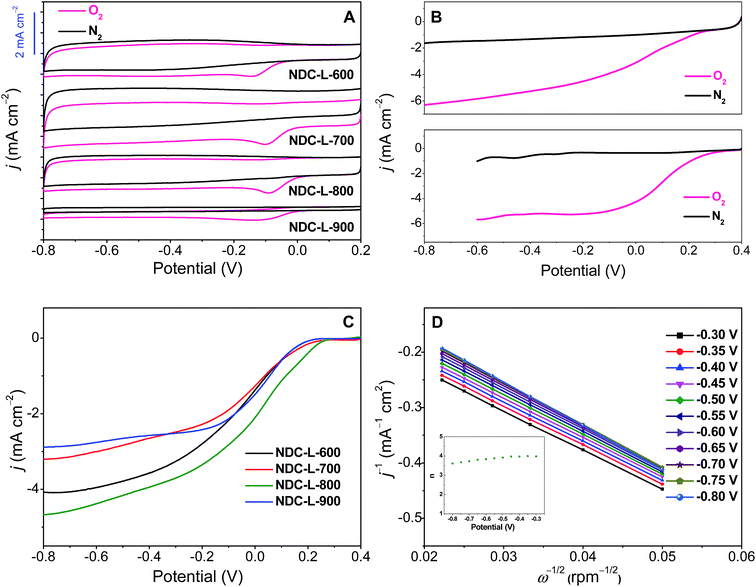 |
| Fig. 3 (A) CV curves of NDC-L-600, NDC-L-700, NDC-L-800, and NDC-L-900 in N2-saturated, O2-saturated 0.1 M KOH solution at a scan rate of 10 mV s−1. (B) RDE curves for NDC-L-800 (upper) and 20 wt% Pt/C (lower) in N2-saturated, O2-saturated 0.1 M KOH solution at a scanning rate of 10 mV s−1 and a rotation speed of 1600 rpm. (C) RDE curves for NDC-L-600, NDC-L-700, NDC-L-800, and NDC-L-900 in O2-saturated 0.1 M KOH with a scan rate of 10 mV s−1 and a rotation speed of 1600 rpm. (D) K–L plots for the ORR in O2-saturated 0.1 M KOH solution for NDC-L-600, NDC-L-700, NDC-L-800, NDC-L-900 and 20 wt% Pt/C. The inset is the electron number calculated from K–L plots. | |
To further understand the kinetics of the ORR on the carbons, linear sweep voltammetry (LSV) measurements were performed in comparison with commercial Pt/C catalysts (20 wt% JM) in an O2-saturated electrolyte as shown in Fig. 3B and C and S4.† Compared to the other samples, a significant positive shift in the onset potential (0.27 V) was demonstrated by NDC-L-800, which is very close to that of the commercial Pt/C (0.30 V). The ORR current of NDC-L-800 (−4.38 mA cm−2) approached that of Pt/C (−4.66 mA cm−2) at −0.6 V. We also performed RDE measurements at various rotating speeds from 400 to 2025 rpm (Fig. 3C). The limiting current density increases with increasing rotation rate. This indicates a shortened diffusion distance at higher rotation rates, which is in accordance with previous reports.15 The ORR kinetics were studied by K–L plots (Fig. 3D and S5†).72 The electrons transferred per O2 (n) were calculated from the K–L equation (inset of Fig. 3D), demonstrating that the ORR proceeds on NDC-L-800 via a 4e− pathway as is the case for the Pt/C catalyst.73,74 The electrochemical properties of all the samples are summarized in Table 1 and a systematic performance comparison of the current work with the others is provided in Table S1.†
The possible crossover effect of catalytic materials is also very important for practical applications. Therefore, the electrocatalytic selectivity of NDC-L-800 as a model material was measured versus the electro-oxidation of methanol. It can be seen from Fig. 4A that after the addition of 3 M methanol to a 0.1 M KOH solution saturated with O2, no noticeable change was observed in the ORR current on the NDC-L-800 electrode. This indicates that the NDC-L-800 exhibits high selectivity towards the ORR and has good ability to avoid crossover effects. However, one pair of peaks was observed for methanol oxidation in the CV curve for the commercial Pt/C, whereas the cathodic peak for the O2 reduction reaction had disappeared after introduction of methanol (Fig. 4B). To examine the effect of CO on the electrocatalytic activity of NDC-L-800, 10% (v/v) CO in air was introduced into the electrolyte. The NDC-L-800 electrode was insensitive to CO (Fig. 4C), whereas the Pt/C electrode was rapidly poisoned under the same conditions. The stability of the catalyst is a key feature in deciding the possibility for its final application in fuel cells. To investigate the electrochemical stability of NDC-L-800, the continuous CV tests were performed in O2-saturated solution and the results were compared with those obtained for the 20% Pt/C catalysts. As shown in Fig. 4D, after 1000 cycles, a characteristic reduction current with a well-defined cathodic peak (at −0.09 V vs. Hg/HgO) was also retained for the NDC-L-800 electrode in an O2-supplied electrolyte, whereas the Pt/C current density decreased to a large extent over this time period (Fig. S6†). The higher stability of NDC-L-800 compared with the Pt/C catalyst may be attributed to the higher strength of the covalent C–N bond and the absence of activity degradation caused by Pt nanoparticle agglomeration and dissolution.75–77
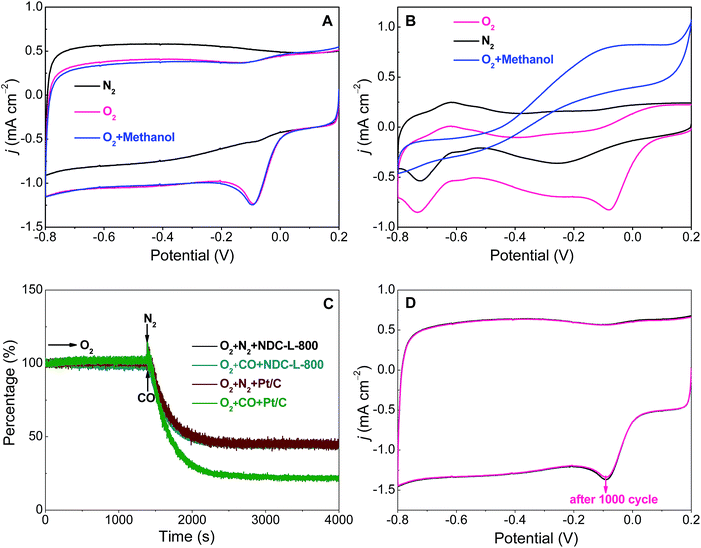 |
| Fig. 4 (A and B) CV curves of NDC-L-800 and 20 wt% Pt/C in N2-saturated, O2-saturated 0.1 M KOH solution, and O2-saturated 0.1 M KOH with 10 vol% methanol at a scan rate of 10 mV s−1. (C) The percentage of current density (j) vs. time chronoamperometric responses obtained at the NDC-L-800 and 20 wt% Pt/C electrodes at −0.30 V in O2-saturated 0.1 M KOH. The arrow indicates the introduction of N2 or CO into the electrolyte. (D) Electrochemical stability of NDC-L-800 as determined by continuous CV scanning in O2-saturated 0.1 M KOH. Scan rate: 10 mV s−1. | |
Where does this enhanced ORR performance come from? The electronegativity difference between doped nitrogen and carbon atoms facilitates the adsorption of oxygen molecules on the carbon surface. From the literature survey and the trend in general, a consensus seems to indicate pyridinic and quaternary N as the active centers for the ORR.78–82 The RDE results of NDC-L-Y demonstrate that the NDC-L-800 sample shows a more positive onset potential with higher current density. According to the XPS results, 23.6% of pyridinic nitrogen contribution in the overall nitrogen content of NDC-L-800 is believed to be the main active site for the ORR. Besides, NDC-L-800 also possesses a contribution from the quaternary form of nitrogen, which is estimated to be 36.1 in the overall nitrogen content. Hence, there is a strong possibility that pyridinic N1 and quaternary N3 play vital roles in reducing the overpotential for the ORR process in our sample, which is also in good agreement with the general consensus. Since the ORR is a surface reaction, specific surface area and nitrogen content could influence the catalyst performance on the ORR. However, in our case, there is not much difference on BET among samples (see Table 1), so nitrogen doping might play an important role in the catalyst performance. Reasonable nitrogen doping in porous carbon along with a high surface area and excellent conductivity leads to a superior electrocatalytic oxygen reduction activity and proves to be a promising alternative for costly Pt-based electrocatalysts in fuel cells in terms of excellent electrocatalytic performance, high selectivity, and long durability.
The discarded stalks of amaranthus and the extract remains of natural amaranthus red are also treated under the same conditions to prepare NDC-S-Y and NDC-E-Y to make full use of the organic-rich waste. Their structural and ORR characterizations are displayed in Fig. S7–S18.† The products obtained at 800 °C show the best ORR performance, comparable to Pt/C under alkaline conditions. Among all the samples, NDC-L-800 shows the best ORR performance.
Conclusion
In summary, NDCs with a high surface area were synthesized from amaranthus waste, which is a natural, abundantly available, and constantly renewable source and acts as a single precursor for nitrogen as well as carbon. The carbon framework is ingrained with nitrogen, which otherwise is incorporated externally through energy-intensive, hazardous, chemical reactions using appropriate organic precursors. The technique is simple, but extremely successful, offering naturally doped conductive highly porous carbon, which leads to a superior electrocatalytic oxygen reduction activity and could prove to be a valuable material for fuel-cell technologies. The effect of temperature on the structural and functional properties is studied, and all the as-obtained carbons are applied as metal-free catalysts for the ORR. Carbon graphitized at 800 °C emerges as a superior ORR electrocatalyst with excellent electrocatalytic performance, high selectivity, and long durability, demonstrating that amaranthus waste carbon can be a promising alternative for costly Pt-based electrocatalysts in fuel cells. Because of its excellent electrocatalytic properties, such a material can act as a promising catalyst for next-generation fuel cells and still has vast unexplored potential in the fields of supercapacitors, water purification, CO2 adsorption, and hydrogen storage. We believe that our as-synthesized carbon will certainly be a trend setter and have greater economic ramifications by creating value-added materials from waste, which otherwise will become an environmental burden.
Acknowledgements
The authors are grateful to the National Science Foundation of China (21071047, 51125015 and 21471048), the Program for New Century Excellent Talents in University of Ministry of Education of China (NCET-11-0944), the Scientific Research Foundation for the Returned Overseas Chinese Scholars, the Excellent Youth Foundation of Henan Scientific Committee (124100510004), the Program for Science & Technology Innovation Talents in Universities of Henan Province (2011HASTIT010), the Research Project of Chinese Ministry of Education (no. 213023A), and the Program for Innovative Research Team in University of Henan Province (no. 14IRTSTHN005).
Notes and references
- M. Winter and R. J. Brodd, Chem. Rev., 2004, 104, 4245 Search PubMed.
- P. G. Bruce, L. J. Hardwick and K. M. Abraham, Mater. Res. Soc. Bull., 2011, 36, 506 CrossRef CAS.
- M. K. Debe, Nature, 2012, 486, 43 CrossRef CAS PubMed.
- Y. Y. Liang, Y. G. Li, H. L. Wang, J. G. Zhou, J. Wang, T. Regier and H. J. Dai, Nat. Mater., 2011, 10, 780 CrossRef CAS PubMed.
- F. Cheng and J. Chen, Chem. Soc. Rev., 2012, 41, 2172 Search PubMed.
- Y. Li, Y. Li, E. Zhu, T. McLouth, C.-Y. Chiu, X. Huang and Y. Huang, J. Am. Chem. Soc., 2012, 134, 12326 Search PubMed.
- A. Morozan, B. Jousselme and S. Palacin, Energy Environ. Sci., 2011, 4, 1238 CAS.
- W. Wang, R. F. Wang, S. Ji, H. Q. Feng and H. Wang, J. Power Sources, 2010, 195, 3498 CrossRef CAS PubMed.
- H. A. Gasteiger, S. S. Kocha, B. Sompalli and F. T. Wagner, Appl. Catal., B, 2005, 56, 9035 CrossRef PubMed.
- H. Wang, R. F. Wang, H. Li, Q. F. Wang, J. Kang and Z. Q. Lei, Int. J. Hydrogen Energy, 2011, 36, 839 CrossRef CAS PubMed.
- K. Gong, F. Du, Z. Xia, M. Durstock and L. Dai, Science, 2009, 323, 760 CrossRef CAS PubMed.
- J. Snyder, T. Fujita, M. Chen and J. Erlebacher, Nat. Mater., 2010, 9, 904 CrossRef CAS PubMed.
- M. Lefevre, E. Proietti, F. Jaouen and J. P. Dodelet, Science, 2009, 324, 71 CrossRef CAS PubMed.
- H. Tang, H. Yin, J. Wang, N. Yang, D. Wang and Z. Tang, Angew. Chem., Int. Ed, 2013, 52, 5585 Search PubMed.
- L. Qu, Y. Liu, J.-B. Baek and L. Dai, ACS Nano, 2010, 4, 1321 CrossRef CAS PubMed.
- E. Biddinger, D. Deak and U. Ozkan, Top. Catal., 2009, 52, 1566 CrossRef CAS.
- Y. Shao, S. Zhang, M. H. Engelhard, G. Li, G. Shao, Y. Wang, J. Liu, I. A. Aksay and Y. Lin, J. Mater. Chem., 2010, 20, 7491 RSC.
- W. Yang, T. P. Fellinger and M. Antonietti, J. Am. Chem. Soc., 2010, 133, 206 CrossRef PubMed.
- T. C. Nagaiah, A. Bordoloi, M. D. Sánchez, M. Muhler and W. Schuhmann, ChemSusChem, 2012, 5, 637 CrossRef CAS PubMed.
- Y. Zheng, Y. Jiao, M. Jaroniec, Y. Jin and S. Z. Qiao, Small, 2012, 8, 3550 CrossRef CAS PubMed.
- Y. Tang, B. L. Allen, D. R. Kauffman and A. Star, J. Am. Chem. Soc., 2009, 131, 13200 CrossRef CAS PubMed.
- R. Liu, D. Wu, X. Feng and K. Müllen, Angew. Chem., Int. Ed., 2010, 49, 2565 CrossRef CAS PubMed.
- K. L. Ai, Y. L. Liu, C. P. Ruan, L. H. Lu and G. Q. Lu, Adv. Mater., 2013, 25, 998 CrossRef CAS PubMed.
- H. T. Liu, Y. Q. Liu and D. B. Zhu, J. Mater. Chem., 2011, 21, 3335 Search PubMed.
- D. W. Boukhvalov and Y. W. Son, Nanoscale, 2012, 4, 417 RSC.
- Y. Gao, G. Hu, J. Zhong, Z. Shi, Y. Zhu, D. S. Su, J. Wang, X. Bao and D. Ma, Angew. Chem., Int. Ed., 2013, 52, 2109 Search PubMed.
- Z. Lin, G. Waller, Y. Liu, M. Liu and C.-P. Wong, Adv. Energy Mater., 2012, 2, 884 CrossRef CAS.
- Z. Liu, H. Nie, Z. Yang, J. Zhang, Z. Jin, Y. Lu, Z. Xiao and S. Huang, Nanoscale, 2013, 5, 3283 RSC.
- C. H. Choi, S. H. Park and S. I. Woo, ACS Nano, 2012, 6, 7084 CrossRef CAS PubMed.
- D. S. Su, ChemSusChem, 2009, 2, 1009 Search PubMed.
- C. Lavorato, A. Primo, R. Molinari and H. Garcia, Chem.–Eur. J., 2014, 20, 187 CrossRef CAS PubMed.
- E. Raymundo-Piñero, M. Cadek and F. Béguin, Adv. Funct. Mater., 2009, 19, 1032 CrossRef.
- G. P. Mane, S. N. Talapaneni, C. Anand, S. Varghese, H. Iwai, Q. Ji, K. Ariga, T. Mori and A. Vinu, Adv. Funct. Mater., 2012, 22, 3596 Search PubMed.
- H.-J. Liu, X.-M. Wang, W.-J. Cui, Y.-Q. Dou, D.-Y. Zhao and Y.-Y. Xia, J. Mater. Chem., 2010, 20, 4223 Search PubMed.
- W. Huang, H. Zhang, Y. Huang, W. Wang and S. Wei, Carbon, 2011, 49, 838 CrossRef CAS PubMed.
- Z. Li, L. Zhang, B. S. Amirkhiz, X. Tan, Z. Xu, H. Wang, B. C. Olsen, C. M. B. Holt and D. Mitlin, Adv. Energy Mater., 2012, 2, 431 CrossRef CAS.
- R. J. White, M. Antonietti and M.-M. Titirici, J. Mater. Chem., 2009, 19, 8645 RSC.
- K. N. Chaudhari, M. Y. Song and J.-S. Yu, Small, 2014, 10, 2625 CrossRef CAS PubMed.
- G. Liu, X. Li, J.-W. Lee and B. N. Popov, Catal. Sci. Technol., 2011, 1, 207 CAS.
- X. Zhou, Z. Yang, H. Nie, Z. Yao, L. Zhang and S. Huang, J. Power Sources, 2011, 196, 9970 CrossRef CAS PubMed.
- J. L. Marx, Science, 1977, 198, 40 CrossRef CAS PubMed.
-
http://en.wikipedia.org/wiki/Amaranth
.
-
http://www.wholehealthmd.com/ME2/dirmod.asp?sid=17E09E7CFFF640448FFB0B4FC1B7FEF0%26nm=Reference+Library%26type=AWHN_Foods%26mod=Foods%26mid=%26id=7404261D57C74A058ACB873C7E17627F%26tier=2
.
- J. Tucker, Bioscience, 1986, 36, 9 CrossRef.
- W. Qian, F. Sun, Y. Xu, L. Qiu, C. Liu, S. Wang and F. Yan, Energy Environ. Sci., 2014, 7, 379 CAS.
- Z. Ju, T. Wang, L. Wang, Z. Xing, L. Xu and Y. Qian, Carbon, 2010, 48, 3420 CrossRef CAS PubMed.
- T. Teklay, A. Nordgren, G. Nyberg and A. Malmer, Appl. Soil Ecol., 2007, 35, 193 CrossRef PubMed.
- S. Wang, Y. Zhu, F. Xia, J. Xi, N. Wang, L. Feng and L. Jiang, Carbon, 2006, 44, 1845 Search PubMed.
- C. Yang, Y.-R. Xu and W.-X. Yao, J. Agric. Food Chem., 2002, 50, 846 Search PubMed.
- R. Wang, P. Wang, X. Yan, J. Lang, C. Peng and Q. Xue, ACS Appl. Mater. Interfaces, 2012, 4, 5800 CAS.
- D. C. Huang, Q. L. Liu, W. Zhang, J. Ding, J. J. Gu, S. M. Zhu, Q. X. Guo and D. Zhan, J. Mater. Sci., 2011, 46, 5064 CrossRef CAS.
- M. U. Dural, L. Cavas, S. K. Papageorgiou and F. K. Katsaros, Chem. Eng. J., 2011, 168, 77 CrossRef CAS PubMed.
- K. Palanichamy and A. Ariharaputhiran, Int. J. Hydrogen Energy, 2013, 38, 2263 Search PubMed.
- Z. Jiang, X. Lv, D. Jiang, J. Xie and D. Mao, J. Mater. Chem. A, 2013, 1, 14963 CAS.
- L. Zhu, Y. Yin, C.-F. Wang and S. Chen, J. Mater. Chem. C, 2013, 1, 4925 RSC.
- C. Peng, X.-b. Yan, R.-t. Wang, J.-w. Lang, Y.-j. Ou and Q.-j. Xue, Electrochim. Acta, 2013, 87, 401 CrossRef CAS PubMed.
- J. Qu, Q. Cong, C. Luo and X. Yuan, RSC Adv., 2013, 3, 961 RSC.
- T. Shanthi and V. M. Selvarajan, J. Chem., 2013, 304970 Search PubMed.
- A. Jain and S. K. Tripathi, J. Solid State Electrochem., 2013, 17, 2545 Search PubMed.
- R. Madhu, K. V. Sankar, S.-M. Chen and R. K. Selvan, RSC Adv., 2014, 4, 1225 RSC.
- E. Akar, A. Altinişik and Y. Seki, Ecol. Eng., 2013, 52, 19 Search PubMed.
- Y. Liu, Y. Zhao and Y. Zhang, Sens. Actuators, B, 2014, 196, 647 CrossRef CAS PubMed.
- Y.-j. Ou, C. Peng, J.-w. Lang, D.-d. Zhu and X.-b. Yan, New Carbon Mater., 2014, 29, 209 CrossRef.
- S. Yang, L. Zhi, K. Tang, X. Feng, J. Maier and K. Müllen, Adv. Funct. Mater., 2012, 22, 3634 CrossRef CAS.
- K. Staczyk, R. Dziembaj, Z. Piwowarska and S. Witkowski, Carbon, 1995, 33, 1383 Search PubMed.
- P. K. Chu and L. Li, Mater. Chem. Phys., 2006, 96, 253 CrossRef CAS PubMed.
- K. N. Kudin, B. Ozbas, H. C. Schniepp, R. K. Prud'homme, I. A. Aksay and R. Car, Nano Lett., 2007, 8, 36 Search PubMed.
- K. S. Kim, Y. Zhao, H. Jang, S. Y. Lee, J. M. Kim, K. S. Kim, J. H. Ahn, P. Kim, J. Y. Choi and B. H. Hong, Nature, 2009, 457, 706 CrossRef CAS PubMed.
- D. Wei, Y. Liu, Y. Wang, H. Zhang, L. Huang and G. Yu, Nano Lett., 2009, 9, 1752 CrossRef CAS PubMed.
- C. Hu, Y. Xiao, Y. Zhao, N. Chen, Z. Zhang, M. Cao and L. Qu, Nanoscale, 2013, 5, 2726 Search PubMed.
- W. M. Zhang, P. Sherrell, A. I. Minett, J. M. Razal and J. Chen, Energy Environ. Sci., 2010, 3, 1286 CAS.
- W. Chen and S. W. Chen, Angew. Chem., Int. Ed., 2009, 48, 4386 CrossRef CAS PubMed.
- S. Gao, H. Fan, X. Wei, L. Li, Y. Bando and D. Golberg, Part. Part. Syst. Charact., 2013, 30, 864 CAS.
- M. S. EI-Deab, T. Sotomora and T. Ohsaka, Electrochem. Commun., 2005, 7, 29 CrossRef PubMed.
- H. Jin, H. M. Zhang, H. X. Zhong and J. L. Zhang, Energy Environ. Sci., 2011, 4, 3389 CAS.
- H. Z. Zhong, H. M. Zhang, Z. Xu, S. S. Liu and J. X. Mao, ChemSusChem, 2012, 5, 1689 Search PubMed.
- H. Zhong, H. Zhang, S. Liu, C. Deng and M. Wang, ChemSusChem, 2013, 6, 807 Search PubMed.
- J.-i. Ozaki, S.-i. Tanifuji, N. Kimura, A. Furuichi and A. Oya, Carbon, 2006, 44, 1324 CrossRef CAS PubMed.
- N. P. Subramanian, X. Li, V. Nallathambi, S. P. Kumaraguru, H. Colon-Mercado, G. Wu, J.-W. Lee and B. N. Popov, J. Power Sources, 2009, 188, 38 CrossRef CAS PubMed.
- S. Yasuda, L. Yu, J. Kim and K. Murakoshi, Chem. Commun., 2013, 49, 9627 RSC.
- D. Geng, Y. Chen, Y. Chen, Y. Li, R. Li, X. Sun, S. Ye and S. Knights, Energy Environ. Sci., 2011, 4, 760 CAS.
- G. Liu, X. Li, J.-W. Lee and B. N. Popov, Catal. Sci. Technol., 2011, 1, 207 CAS.
Footnote |
† Electronic supplementary information (ESI) available: Additional experimental data. See DOI: 10.1039/c4ee02087a |
|
This journal is © The Royal Society of Chemistry 2015 |