Theoretical analysis of [5.5.6]cyclacenes: electronic properties, strain energies and substituent effects†
Received
19th January 2015
, Accepted 11th February 2015
First published on 12th February 2015
Abstract
A novel class of cyclic conjugated molecules, composed of annelated five- and six-membered rings, is proposed and theoretically investigated using density functional theory and multireference methods with regards to their structures, strain energies, aromaticity (NICS values), electronic ground states, band gaps, and the effect of substituents. These [5.5.6]ncyclacenes are predicted to be low band gap materials (below 1 eV) with, depending on their size, closed-shell singlet ground states. The strain energies from n = 4 upwards lie in the range of the synthetically known [n]cycloparaphenylenes. An investigation of the effect of rim-substitution by methyl, alkynyl, thiomethyl or phenyl groups on the electronic ground states showed that thiomethyl-substitution leads to [5.5.6]ncyclacenes with closed-shell singlet ground states for all sizes n investigated.
Introduction
Hoop-shaped molecules, consisting of linearly connected or annelated conjugated rings, have attracted chemists since 1954.1 Of particular interest are their potentially aromatic character, their conjugative and optoelectronic properties, and their use in host–guest chemistry.2–13 Prominent examples are [6]ncyclacenes (1n), which can be regarded as subunits of zig-zag carbon nanotubes (CNTs) as well as cyclo[n]phenacenes (2n) and [n]cycloparaphenylenes ([n]CPPs) (3n) as subunits of armchair CNTs (Fig. 1). While a derivative of cyclo[10]phenacene14,15 and several [n]CPPs7,16–33 have been synthesized, [6]ncyclacenes (1n) have remained elusive to synthesis up to date.2,5–7,34–45 The reason for this is likely found in their electronic character: [6]ncyclacenes, which have been the subject of a number of theoretical investigations,46–55 are predicted to possess open-shell singlet diradical ground states,52 while for cyclo[n]phenacenes (2n) large singlet–triplet splittings are expected.56 This difference in stability can, according to Clar,57 be deduced from the number of aromatic sextets that can be drawn: none for 1n and n for 2n, resulting in a significantly higher stability for 2n. Alternative structures to 1n consisting of not only six- but also five- to eight-membered rings have been proposed and in few cases synthesized. [6.8]3Cyclacene (4)58–60 and several derivatives of [4.8]3cyclacenes (5)61–63 could be synthesized, and [5.7]ncyclacenes64 as isomers of 1n were proposed and predicted to possess closed-shell singlet ground states. Cyclacenes consisting of annelated five- and six-membered rings have not been investigated yet, although this combination of ring sizes is prevalent in graphitic materials such as fullerenes and the end-caps of CNTs and in optoelectronic materials.65–67 Herein, [5.5.6]ncyclacenes (6n) are proposed as a new cyclacene family and investigated with regards to their structures, strain energies, aromaticity, electronic ground states, band gaps, and the effect of substituents. In cyclacenes 6n, an aromatic sextet can be drawn in every six-membered ring, thereby indicating that a high stability can be anticipated.
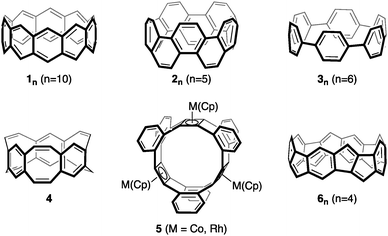 |
| Fig. 1 [6]10Cyclacene (110), cyclo[10]phenacene (25), [6]cycloparaphenylene (36), [6.8]3cyclacene (4), (Cp)M-capped [4.8]3cyclacene derivatives 5, and [5.5.6]4cyclacene (64). | |
Results and discussion
Computational details
The geometries and strain energies of 6n (n = 3–8) were calculated using density functional theory (DFT). The geometries and energies of triplet and open-shell singlet states were assessed using DFT and time-dependent DFT (TDDFT). For single-point energies a combination of complete active space self-consistent field (CASSCF)68,69 calculations with second-order perturbation theory (CASPT2) was employed.70 NMR chemical shifts for NICS (Nucleus Independent Chemical Shift)71,72 values were calculated using the GIAO (Gauge Including Atomic Orbital)73 method. All DFT calculations were performed with the Turbomole74 program package including D375 and using the resolution-of-identity (RI) approximation.76,77 CASSCF and CASPT2 calculations were done with Molpro.78,79 All singlet and triplet geometries (6n and 10n–13n) were first optimized using PBE80-D3/def2-SVP81 followed by harmonic vibrational frequency analyses to confirm minima as stationary points. The structures were then re-optimized using TPSS82-D3 or BP8683–85-D3 in combination with the triple-ζ basis set def2-TZVP.86
Geometries and strain energies
The [5.5.6]ncyclacenes (6n) (n = 3–8, optimized using TPSS-D3/def2-TZVP in C1 symmetries) all possess almost perfect Dn symmetrical structures. Bond lengths of 63 and 64 as representative examples and diameters of 6n are listed in Fig. 2. The strain energy of hoop-shaped molecules is an important parameter and can present a challenge to their synthesis. For [5.5.6]ncyclacenes (6n), strain energies were estimated by comparison with the hypothetical strain-free subunit 7 at the TPSS-D3/def2-TZVP level of theory (Fig. 3).
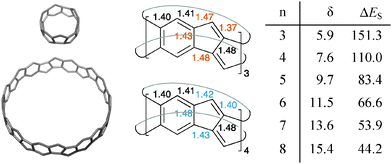 |
| Fig. 2 Calculated structures of 63 and 68, bond lengths (Å) in 63 and 64, and diameters δ (Å) and strain energies ΔES (kcal mol−1) of [5.5.6]ncyclacenes (6n) (TPSS-D3/def2-TZVP, optimized in point group C1). | |
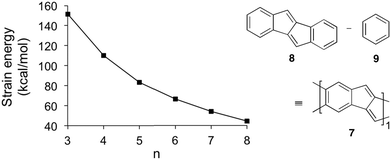 |
| Fig. 3 Plot of strain energy vs. hoop size n of [5.5.6]ncyclacenes (6n) (TPSS-D3/def2-TZVP, optimized in point group C1; strain energies were calculated by subtracting n-times the energy of 7 from the energy of 6n) and homodesmotic equation used to calculate the energy of the strain-free subunit 7. | |
The energy of 7 was calculated using the homodesmotic equation shown in Fig. 3 as the energy difference between dibenzo[a,e]pentalene (DBP, 8) and benzene (9). Homodesmotic equations87 have been used before to calculate strain energies of bent sp2 hybridized structures.60,64,88 Subtracting n-times the energy of 7 from the energy of cyclacene 6n gives a measure of its strain energy.
With increasing hoop size the strain energies of [5.5.6]ncyclacenes (6n) decrease from 151 kcal mol−1 for n = 3 to 44 kcal mol−1 for n = 8 (Fig. 2 and 3). In order to set these values in relation to experimentally known compounds, a comparison with the well-investigated [n]CPPs (3n) is suitable. For [6]CPP, which has been synthesized and is a stable compounds,21,22 the strain energy was calculated according to Segawa et al.88 to be 86 kcal mol−1 (TPSS-D3/def2-TZVP), and recently the even smaller and more strained [5]CPP was synthesized.18 While for cyclacenes 6n for n = 3 and 4 higher strain energies were calculated (110 kcal mol−1 for 64), from n = 5 upwards (83 kcal mol−1) the strain energies of cyclacenes 6n lie well below that of [6]CPP.
An evaluation of the calculated bond lengths in [5.5.6]ncyclacenes (6n) shows that four of the bonds in the five-membered rings vary between odd and even numbers of n (colored values in Fig. 2). For odd n (63, 65, and 67), all bond lengths are similar to those in DBP (8, see ESI,† Table S1), displaying little bond lengths alternation in the six-membered rings and a short length for the double bond in the five-membered rings. This indicates the presence of an aromatic sextet in each phenyl ring. For even n (64, 66, and 68), both “transannular” bonds, connecting the top and bottom rim of the cyclacenes, are relatively long (1.48 Å), formally resulting in two conjugated annulenes with equalized bond lengths between 1.40 and 1.43 Å connected by transannular (single) bonds.
Aromatic character
NICS values were calculated (B3LYP89,90/def2-QZVP91 on TPSS-D3/def2-TZVP optimized geometries) to assess the aromatic character of cyclacenes 6n (Table 1). Strongly positive values denote antiaromaticity, while significantly negative values point towards aromaticity.71,72 As location the geometrical centers of the molecules were chosen as well as a point 1 Å above the center of the individual five- and six-membered rings (NICS(1)).71,72 The NICS values vary between odd and even numbers of n: at the center of 6n, the NICS values are slightly positive for odd n, while for even n strongly negative NICS values are found. For odd n, the NICS(1) values above the five- and six-membered rings adopt values similar to those of DBP (8, Table 1) and show only small variations with increasing hoop size. The absolute NICS(1) values above the six-membered rings are small for both odd and even n, giving no clear indication of aromaticity. The NICS(1) values above the five-membered rings are slightly larger in absolute value, but adopt a significant value only for n = 8 (11.2), indicating some degree of antiaromaticity. Hence, for odd sizes of n the NICS values indicate properties similar to DBP (8), which is a stable compound in spite of its electron count (16π electrons).
Table 1 NICS valuesa of cyclacenes 6n and dibenzopentalene (8)
B3LYP/def2-QZVP//TPSS-D3/def2-TZVP.
NICS value in the center of the molecule.
NICS(1) 1 Å above the 5-membered ring.
NICS(1) 1 Å above the 6-membered ring (both outside the hoop).
|
|
Electronic ground states
Next the electronic ground states of [5.5.6]ncyclacenes (6n) were investigated. Since [6]ncyclacenes (1n) were predicted to possess open-shell singlet diradical ground states,52 which likely inhibited their synthesis up to date,2,5–7,34–44 the stability of both the triplet and open-shell singlet relative to the closed-shell singlet states in cyclacenes 6n is of interest. In order to increase computational speed, and since the optimized structures of cyclacenes 6n showed almost perfect Dn symmetries, their geometries were re-optimized in the respective Dn point group using TPSS-D3 and BP86-D3 in combination with def2-TZVP. The energy differences between structures optimized in C1 and in Dn symmetry were minimal.
To assess whether DFT, which is based on a single-reference configuration, is appropriate to describe the electronic states of [5.5.6]ncyclacenes (6n), single-point (SP) multireference CASSCF calculations were performed on the TPSS-D3/def2-TZVP-optimized geometries. For cyclacenes 63, 64, and 65, CASSCF(8,8)/def2-SVP calculations resulted in natural occupation numbers of the four highest occupied orbitals (see ESI,† Table S2) between 1.93 and 1.91 (n = 3), 1.94 to 1.83 (n = 4), and between 1.93 and 1.92 for n = 5. For [6]6cyclacene (16) with a predicted open-shell singlet diradical ground state, the natural occupation numbers of the four highest occupied orbitals were calculated to be between 1.98 and 1.46 (CASSCF(8,8)/6-31G*), resulting in 0.76 electrons outside the closed-shell orbital space and indicating significant multi-configurational character of the singlet state.52 These values are significantly lower for [5.5.6]ncyclacenes (6n): 0.32 for 63, 0.44 for 64, and 0.29 for 65. Hence, for cyclacenes 6n, the closed-shell orbital space is almost completely occupied, and a single-reference method such as DFT is considered appropriate.
The structures and energies of the triplet states (T) of cyclacenes 6n were assessed through optimizations at the BP86-D3/def2-TZVP level of theory using unrestricted wavefunctions (UHF), and their energies were compared to the closed-shell singlet (CSS) states (Fig. 4, T-CSS, and Table 2). For all sizes investigated (n = 3–8), the triplet states are higher in energy than the CS singlet states at this level of theory. The energy differences decrease with increasing hoop size and assume rather small values (below 5 kcal mol−1) for n = 6–8. A variation between odd and even numbers of n is observed, where for odd n the closed-shell singlets are more stabilized than for even n. This could be due to the total π electron count in 6n resulting in (4m + 2) (odd n) or 4m π electrons (even n).
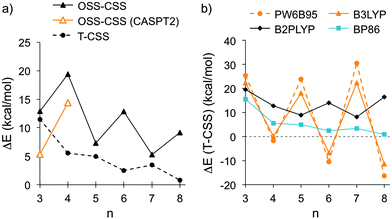 |
| Fig. 4 (a) Energy differences between open-shell and closed-shell singlet states (OSS-CSS), calculated using TDDFT (BP86-D3/def2-TZVP) or CASPT2/def2-SVP//TPSS-D3/def2-TZVP, and between triplet (T) and CSS states (BP86-D3/def2-TZVP) of cyclacenes 6n; (b) energy differences between T and CSS states of 6n calculated using different density functionals (functional-D3/def2-QZVP//TPSS-D3/def2-TZVP, see also Table 2). | |
Table 2 Energy differences (kcal mol−1) between triplet (T) or open-shell singlet (OSS) and closed-shell singlet (CSS) states of [5.5.6]ncyclacenes (6n) and band gaps (eV)
n
|
3 |
4 |
5 |
6 |
7 |
8 |
BP86-D3/def2-TZVP.
From TDDFT calculations.
CASPT2/def2-SVP//TPSS-D3/def2-TZVP.
Functional-D3/def2-QZVP//TPSS-D3/def2-TZVP.
Lowest energy singlet excitation.
|
ΔE (OSS-CSS)a,b |
12.9 |
19.4 |
7.3 |
12.8 |
5.3 |
9.1 |
ΔE (OSS-CSS)c |
5.3 |
14.4 |
— |
— |
— |
— |
ΔE (T-CSS)a |
11.4 |
5.5 |
5.0 |
2.5 |
3.5 |
0.8 |
|
ΔE (T-CSS)d |
PW6B95 |
25.3 |
−1.6 |
23.9 |
−10.5 |
30.5 |
−16.3 |
B3LYP |
22.4 |
0.1 |
18.1 |
−6.8 |
22.3 |
−11.4 |
B2PLYP |
19.5 |
12.7 |
8.9 |
14.0 |
8.1 |
16.4 |
BP86 |
15.6 |
5.6 |
4.9 |
2.5 |
3.4 |
0.8 |
|
Band gapa,b,e [eV] |
0.72 |
0.60 |
0.44 |
0.35 |
0.33 |
0.22 |
The geometries and energies of the open-shell singlet (OSS) states of cyclacenes 6n were assessed using TDDFT, and energies for n = 3 and 4 were also calculated at the CASPT2 level of theory. In attempted structure optimizations of the OSS states of cyclacenes 6n using unrestricted wavefunctions (UHF), relaxation to the CSS states occurred. Hence, OSS energies were obtained through structural optimizations of the lowest excited singlet states using TDDFT with BP86-D3/def2-TZVP and compared to the CSS energies (Fig. 4, OSS-CSS, and Table 2). For all sizes investigated (n = 3–8), the open-shell singlet states are higher in energy than the closed-shell singlet as well as the triplet states. A variation between odd and even numbers of n is observed, but the trend is reversed compared to the triplet states. The reason for this is not clear, however, an odd–even variation in relative energies has also been found for [6]ncyclacenes (1n).47,48,52 The SP energies of the CSS and OSS states of 6n (n = 3 and 4) were also calculated using CASPT2/def2-SVP. In CASPT2, as opposed to CASSCF, dynamic electron correlation is included.92 As in the TDDFT calculations, the open-shell singlets have a higher energy then the closed-shell singlets (Fig. 4, OSS-CSS (CASPT2), and Table 2), but the energy differences are smaller. This result is not surprising, since it is known that the CASPT2 approximation overestimates the stabilization of biradical states.93,94
The assessment of the electronic states of [5.5.6]ncyclacenes (6n) shows that at the BP86-D3/def2-TZVP level of theory their ground states are closed-shell singlets. However, with increasing n the triplet states become more stabilized, and hence the question arose which ground states other density functionals would predict. For this investigation a variety of exchange–correlation functionals was chosen: the generalized gradient approximation (GGA) functional BP86, the meta-GGA functional TPSS, the hybrid GGA functionals BHLYP,95 PW6B95,96 PBE0,97,98 and B3LYP, and the double-hybrid functional B2PLYP,99 each in combination with the quadruple-ζ basis set def2-QZVP. SP energies of the singlet and triplet states of cyclacenes 6n were calculated on the respective TPSS-D3/def2-TZVP-optimized Dn geometries. A comparison of the resulting T-CSS energy differences (Fig. 4, Table 2 and ESI,† Table S8) allows several conclusions: a variation between odd and even numbers of n is found for all types of functionals. Its extent increases with growing amount of exact (HF) exchange in the functional (0% HF exchange in BP86 and TPSS vs. 28% in PW6B95 and 50% in BHLYP). However, with B2PLYP, which in addition to a large amount (53%) of HF exchange employs perturbative second-order correlation (PT2), the preference for odd or even n is reversed compared to all other functionals employed.
As a result, all hybrid (GGA) functionals investigated predict closed-shell singlet ground states for odd 6n with n = 3, 5, 7 and triplet ground states for n = 6, 8, while the (meta-)GGA functionals investigated predict a preference for the closed-shell singlet states for all n. According to the double-hybrid functional B2PLYP, which has been shown to outperform the other functionals employed,100 the ground states of cyclacenes 6n are closed-shell singlets for all n (3–8) with significant energy differences to the triplet states between 8 and 20 kcal mol−1.
Band gaps
The band gaps of cyclacenes 6n were estimated from TDDFT calculations as the excitation energies of the first singlet excited states (Table 2). This has been shown to give more accurate values than using the differences between the calculated HOMO and LUMO energies.101 The band gaps of [5.5.6]ncyclacenes (6n) decrease with growing hoop size from 0.72 eV (n = 3) to 0.22 eV (n = 8), classifying them as organic semiconductors with small band gaps. In comparison, the band gap for DBP (8) is 1.98 eV at the same level of theory. The frontier molecular orbitals of cyclacenes 6n are predominantly localized on the pentalene units (Fig. 5). In the odd-membered cyclacenes 6n (n = 3, 5, 7), HOMO and LUMO resemble their counterparts in DBP (8), while for even n (64, 66, and 68), the reverse is true: the HOMO resembles the LUMO in 8 and vice versa.
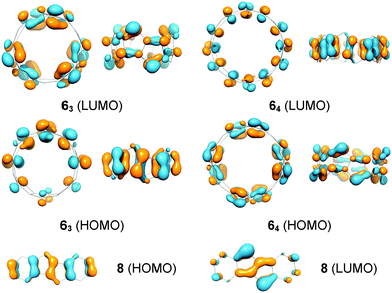 |
| Fig. 5 Frontier molecular orbitals of [5.5.6]ncyclacenes 63 and 64 and dibenzopentalene (8) (BP86-D3/def2-TZVP). | |
Substituent effects
The stability of the closed-shell singlets of [5.5.6]ncyclacenes (6n) showed a strong dependence on the density functional employed. Hence it was wondered whether substituents on the cyclacene rim would lead to a stronger preference for the CSS states. This effect has been investigated for linear acenes, where substituents, in particular thioaryl substituents, were shown to stabilize the closed-shell singlet ground state.102 In cyclacenes 6n, substituents can be attached either on the five- or on the six-membered rings. Considering the orbital coefficients at the respective carbon atoms in the HOMO and LUMO (Fig. 5), the strongest electronic influence (larger coefficient) can be expected from substitution at the five-membered rings. As substituents, methyl, thiomethyl, alkynyl and phenyl were chosen (10n–13n, Fig. 6). The structures of 10n–13n were optimized in Dn symmetries (apart from 133 and the OSS of 103, for which minima were found only in C1 symmetry) using TPSS-D3/def2-TZVP as well as BP86-D3/def2-TZVP. SP energies of the closed-shell singlets and triplets were calculated using the two hybrid functionals PW6B95 and B3LYP as well as the GGA functional BP86 in combination with def2-QZVP for 10n–11n and def2-TZVP for 12n–13n. OSS energies were calculated using TDDFT (BP86-D3/def2-TZVP).
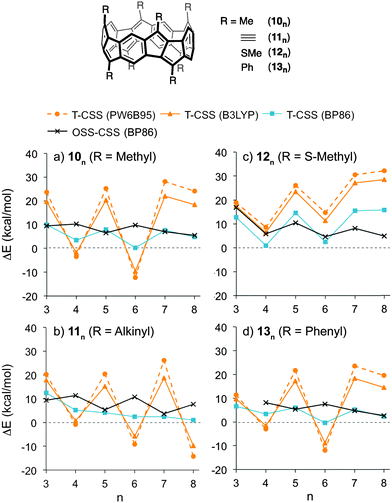 |
| Fig. 6 Energy differences between triplet (T) or open-shell singlet (OSS) and closed-shell singlet (CSS) states of substituted cyclacenes 10n–13n calculated with different density functionals (functional-D3/def2-QZVP (a, b) or def2-TZVP (c, d) on TPSS-D3/def2-TZVP-optimized geometries). | |
A comparison of the relative energies (Fig. 6 and ESI†) shows that for methyl (10n), alkynyl (11n) or phenyl (13n) substituents, the T-CSS and OSS-CSS energies are similar to those of the unsubstituted cyclacenes 6n (Fig. 4) (apart from n = 8 for 10n and 13n). Thiomethyl substituents (12n), on the other hand, have a stabilizing effect on the CSS states. With all functionals investigated, the closed-shell singlet states of 12n are more stable than the triplet or open-shell singlet states. Hence thiomethyl groups seem to be the best substituent in order to achieve electronically stable ground states.
Conclusions
In summary, [5.5.6]ncyclacenes (6n), composed of annelated five- and six-membered rings, have been proposed as a new cyclacene family and theoretically investigated with regards to their structures, strain energies, aromaticity, electronic ground states, band gaps, and the effect of substituents. Generally, a variation between odd and even numbers of n is observed. While in the unsubstituted cyclacenes 6n for even numbers of n the calculated properties indicate structures with potentially antiaromatic character or low-lying diradical states, for odd n closed-shell singlet ground states are predicted and electronic properties similar to dibenzopentalene (8). For all sizes n, low bandgaps below 1 eV were found. An investigation of substituent effects on the electronic ground states showed that rim-substitution by thiomethyl groups leads to cyclacenes with closed-shell singlet ground states for all sizes n investigated. Hence cyclacenes 6n or in particular their thiomethyl substituted derivatives 12n are attractive synthetic targets, and studies towards their synthesis are currently underway in our laboratory.
Acknowledgements
B.E. thanks Prof. Stefan Grimme and Prof. Gebhard Haberhauer for helpful discussions and S. Grimme for usage of computational resources. Generous support by the German Research Foundation (Emmy Noether fellowship for B.E., ES 361/2-1) and the Chemical Industry Trust (Liebig Fellowship, Li 189/11) is gratefully acknowledged.
Notes and references
- E. Heilbronner, Helv. Chim. Acta, 1954, 37, 921–935 CrossRef CAS
.
- R. Gleiter, B. Esser and S. C. Kornmayer, Acc. Chem. Res., 2009, 42, 1108–1116 CrossRef CAS PubMed
.
- L. T. Scott, Angew. Chem., 2003, 115, 4265–4267 CrossRef
.
- L. T. Scott, Angew. Chem., Int. Ed., 2003, 42, 4133–4135 CrossRef CAS PubMed
.
- R. Gleiter, B. Hellbach, S. Gath and R. J. Schaller, Pure Appl. Chem., 2006, 78, 699–706 CrossRef CAS
.
- K. Tahara and Y. Tobe, Chem. Rev., 2006, 106, 5274–5290 CrossRef CAS PubMed
.
- P. J. Evans and R. Jasti, Top. Curr. Chem., 2014, 349, 249–290 CrossRef
.
- B. D. Steinberg and L. T. Scott, Angew. Chem., 2009, 121, 5504–5507 CrossRef
.
- B. D. Steinberg and L. T. Scott, Angew. Chem., Int. Ed., 2009, 48, 5400–5402 CrossRef CAS PubMed
.
- Q. Ye and C. Chi, Chem. Mater., 2014, 26, 4046–4056 CrossRef CAS
.
- K. Itami, Pure Appl. Chem., 2012, 84, 907–916 CrossRef CAS PubMed
.
-
R. Herges, Modern Cyclophane Chemistry, Wiley-VCH, Weinheim, 2004 Search PubMed
.
- A. Schröder, H.-B. Mekelburger and F. Vögtle, Top. Curr. Chem., 1994, 172, 179–201 CrossRef
.
- E. Nakamura, K. Tahara, Y. Matsuo and M. Sawamura, J. Am. Chem. Soc., 2003, 125, 2834–2835 CrossRef CAS PubMed
.
- Y. Matsuo, K. Tahara, M. Sawamura and E. Nakamura, J. Am. Chem. Soc., 2004, 126, 8725–8734 CrossRef CAS PubMed
.
- R. Friederich, M. Nieger and F. Vögtle, Chem. Ber., 1993, 126, 1723–1732 CrossRef CAS
.
- F. Sibbel, K. Matsui, Y. Segawa, A. Studer and K. Itami, Chem. Commun., 2014, 50, 954–956 RSC
.
- P. J. Evans, E. R. Darzi and R. Jasti, Nat. Chem., 2014, 6, 404–408 CrossRef CAS PubMed
.
- E. R. Darzi, T. J. Sisto and R. Jasti, J. Org. Chem., 2012, 77, 6624–6628 CrossRef CAS PubMed
.
- J. Xia, J. W. Bacon and R. Jasti, Chem. Sci., 2012, 3, 3018–3021 RSC
.
- J. Xia and R. Jasti, Angew. Chem., 2012, 124, 2524–2526 CrossRef
.
- J. Xia and R. Jasti, Angew. Chem., Int. Ed., 2012, 51, 2474–2476 CrossRef CAS PubMed
.
- T. J. Sisto, M. R. Golder, E. S. Hirst and R. Jasti, J. Am. Chem. Soc., 2011, 133, 15800–15802 CrossRef CAS PubMed
.
- T. Iwamoto, Y. Watanabe, Y. Sakamoto, T. Suzuki and S. Yamago, J. Am. Chem. Soc., 2011, 133, 8354–8361 CrossRef CAS PubMed
.
- Y. Segawa, P. Šenel, S. Matsuura, H. Omachi and K. Itami, Chem. Lett., 2011, 40, 423–425 CrossRef CAS
.
- Y. Segawa, S. Miyamoto, H. Omachi, S. Matsuura, P. Šenel, T. Sasamori, N. Tokitoh and K. Itami, Angew. Chem., Int. Ed., 2011, 50, 3244–3248 CrossRef CAS PubMed
.
- S. Yamago, Y. Watanabe and T. Iwamoto, Angew. Chem., 2010, 122, 769–771 CrossRef
.
- S. Yamago, Y. Watanabe and T. Iwamoto, Angew. Chem., Int. Ed., 2010, 49, 757–759 CrossRef CAS PubMed
.
- H. Omachi, S. Matsuura, Y. Segawa and K. Itami, Angew. Chem., 2010, 122, 10400–10403 CrossRef
.
- H. Omachi, S. Matsuura, Y. Segawa and K. Itami, Angew. Chem., Int. Ed., 2010, 49, 10202–10205 CrossRef CAS PubMed
.
- H. Takaba, H. Omachi, Y. Yamamoto, J. Bouffard and K. Itami, Angew. Chem., 2009, 121, 6228–6232 CrossRef
.
- H. Takaba, H. Omachi, Y. Yamamoto, J. Bouffard and K. Itami, Angew. Chem., Int. Ed., 2009, 48, 6112–6116 CrossRef CAS PubMed
.
- R. Jasti, J. Bhattacharjee, J. B. Neaton and C. R. Bertozzi, J. Am. Chem. Soc., 2008, 130, 17646–17647 CrossRef CAS PubMed
.
- D. Eisenberg, R. Shenhar and M. Rabinovitz, Chem. Soc. Rev., 2010, 39, 2879–2890 RSC
.
- C. Denekamp, A. Etinger, W. Amrein, A. Stanger, M. Stuparu and A. D. Schlüter, Chem. – Eur. J., 2008, 14, 1628–1637 CrossRef CAS PubMed
.
- M. Stuparu, D. Lentz, H. Rüegger and A. D. Schlüter, Eur. J. Org. Chem., 2007, 88–100 CrossRef CAS
.
- M. Stuparu, V. Gramlich, A. Stanger and A. D. Schlüter, J. Org. Chem., 2007, 72, 424–430 CrossRef CAS PubMed
.
- W. D. Neudorff, D. Lentz, M. Anibarro and A. D. Schlüter, Chem. – Eur. J., 2003, 9, 2745–2757 CrossRef CAS PubMed
.
- O. Kintzel, P. Luger, M. Weber and A.-D. Schlüter, Eur. J. Org. Chem., 1998, 99–105 CrossRef CAS
.
- R. M. Cory and C. L. McPhail, Tetrahedron Lett., 1996, 37, 1987–1990 CrossRef CAS
.
- R. M. Cory, C. L. McPhail, A. J. Dikmans and J. J. Vittal, Tetrahedron Lett., 1996, 37, 1983–1986 CrossRef CAS
.
- P. R. Ashton, J. P. Mathias and F. J. Stoddart, Synthesis, 1993, 221–224 CrossRef CAS PubMed
.
- P. R. Ashton, G. R. Brown, N. S. Isaacs, D. Giuffrida, F. H. Kohnke, J. P. Mathias, A. M. Z. Slawin, D. R. Smith, J. F. Stoddart and D. J. Williams, J. Am. Chem. Soc., 1992, 114, 6330–6353 CrossRef CAS
.
- A. Godt, V. Enkelmann and A.-D. Schlüter, Angew. Chem., 1989, 101, 1704–1706 CrossRef CAS
.
- A. Godt, V. Enkelmann and A.-D. Schlüter, Angew. Chem., Int. Ed. Engl., 1989, 28, 1680–1682 CrossRef
.
- J. Aihara, J. Chem. Soc., Perkin Trans. 2, 1994, 971–974 RSC
.
- H. S. Choi and K. S. Kim, Angew. Chem., 1999, 111, 2400–2402 CrossRef
.
- H. S. Choi and K. S. Kim, Angew. Chem., Int. Ed., 1999, 38, 2256–2258 CrossRef CAS
.
- L. Türker, J. Mol. Struct.: THEOCHEM, 2000, 531, 333–337 CrossRef
.
- L. Türker, J. Mol. Struct.: THEOCHEM, 2001, 536, 235–241 CrossRef
.
- K. N. Houk, P. S. Lee and M. Nendel, J. Org. Chem., 2001, 66, 5517–5521 CrossRef CAS PubMed
.
- Z. Chen, D. Jiang, X. Lu, H. F. Bettinger, S. Dai, P. von R. Schleyer and K. N. Houk, Org. Lett., 2007, 9, 5449–5452 CrossRef CAS PubMed
.
- M. Z. Kassaee, H. Aref Rad and S. Soleimani Amiri, Monatsh. Chem., 2010, 141, 1313–1319 CrossRef CAS
.
- D. Sadowsky, K. McNeill and C. J. Cramer, Faraday Discuss., 2010, 145, 507–521 RSC
.
- H. S. Rzepa, Chem. Rev., 2005, 105, 3697–3715 CrossRef CAS PubMed
.
- Y. Matsuo, K. Tahara and E. Nakamura, Org. Lett., 2003, 5, 3181–3184 CrossRef CAS PubMed
.
-
E. Clar, The aromatic sextet, Wiley, New York, 1972 Search PubMed
.
- B. Esser, F. Rominger and R. Gleiter, J. Am. Chem. Soc., 2008, 130, 6716–6717 CrossRef CAS PubMed
.
- B. Esser, A. Bandyopadhyay, F. Rominger and R. Gleiter, Chem. – Eur. J., 2009, 15, 3368–3379 CrossRef CAS PubMed
.
- B. Esser, J. A. Raskatov and R. Gleiter, Org. Lett., 2007, 9, 4037–4040 CrossRef CAS PubMed
.
- B. Hellbach, F. Rominger and R. Gleiter, Angew. Chem., 2004, 116, 5970–5973 CrossRef
.
- B. Hellbach, F. Rominger and R. Gleiter, Angew. Chem., Int. Ed., 2004, 43, 5846–5849 CrossRef CAS PubMed
.
- S. C. Kornmayer, B. Hellbach, F. Rominger and R. Gleiter, Chem. – Eur. J., 2009, 15, 3380–3389 CrossRef CAS PubMed
.
- E. S. Hirst, F. Wang and R. Jasti, Org. Lett., 2011, 13, 6220–6223 CrossRef CAS PubMed
.
- D. T. Chase, A. G. Fix, S. J. Kang, B. D. Rose, C. D. Weber, Y. Zhong, L. N. Zakharov, M. C. Lonergan, C. Nuckolls and M. M. Haley, J. Am. Chem. Soc., 2012, 134, 10349–10352 CrossRef CAS PubMed
.
- J. D. Wood, J. L. Jellison, A. D. Finke, L. Wang and K. N. Plunkett, J. Am. Chem. Soc., 2012, 134, 15783–15789 CrossRef CAS PubMed
.
- M. Nakano, I. Osaka, K. Takimiya and T. Koganezawa, J. Mater. Chem. C, 2014, 2, 64–70 RSC
.
- H.-J. Werner and P. J. Knowles, J. Chem. Phys., 1985, 82, 5053–5063 CrossRef CAS PubMed
.
- P. J. Knowles and H.-J. Werner, Chem. Phys. Lett., 1985, 115, 259–267 CrossRef CAS
.
- P. Celani and H.-J. Werner, J. Chem. Phys., 2000, 112, 5546–5557 CrossRef CAS PubMed
.
- P. V. R. Schleyer, C. Maerker, A. Dransfeld, H. Jiao and N. J. R. van E. Hommes, J. Am. Chem. Soc., 1996, 118, 6317–6318 CrossRef CAS
.
- P. von R. Schleyer, J. Jiao, N. J. R. van E. Hommes, V. G. Malkin and O. L. Malkina, J. Am. Chem. Soc., 1997, 119, 12669–12670 CrossRef CAS
.
- M. Häser, R. Ahlrichs, H. P. Baron, P. Weis and H. Horn, Theor. Chim. Acta, 1992, 83, 455–470 CrossRef
.
- R. Ahlrichs, M. Bär, H. Marco, H. Horn and C. Kölmel, Chem. Phys. Lett., 1989, 162, 165–169 CrossRef CAS
(TURBOMOLE, University of Karlsruhe, Germany, 2012, http://www.turbomole.com).
- S. Grimme, J. Antony, S. Ehrlich and H. Krieg, J. Chem. Phys., 2010, 132, 154104 CrossRef PubMed
.
- K. Eichkorn, O. Treutler, H. Öhm, H. Marco and R. Ahlrichs, Chem. Phys. Lett., 1995, 240, 283–290 CrossRef CAS
.
- K. Eichkorn, O. Treutler, H. Öhm, H. Marco and R. Ahlrichs, Chem. Phys. Lett., 1995, 242, 652–660 CrossRef CAS
.
- H.-J. Werner, P. J. Knowles, G. Knizia, F. R. Manby and M. Schütz, Wiley Interdiscip. Rev.: Comput. Mol. Sci., 2012, 2, 242–253 CrossRef CAS
.
- MOLPRO, Version 2012.1, a package of ab initio programs. H.-J. Werner, P. J. Knowles, G. Knizia, F. R. Manby, M. Schütz and others, 2012.
- J. P. Perdew, K. Burke and M. Ernzerhof, Phys. Rev. Lett., 1996, 77, 3865–3868 CrossRef CAS
.
- A. Schaäfer, H. Horn and R. Ahlrichs, J. Chem. Phys., 1992, 97, 2571–2577 CrossRef PubMed
.
- J. Tao, J. P. Perdew, V. N. Staroverov and G. E. Scuseria, Phys. Rev. Lett., 2003, 91, 146401 CrossRef
.
- A. D. Becke, Phys. Rev. A, 1988, 38, 3098–3100 CrossRef CAS
.
- J. P. Perdew, Phys. Rev. B, 1986, 33, 8822–8824 CrossRef
.
- J. P. Perdew, Phys. Rev. B, 1986, 34, 7406 CrossRef
.
- A. Schäfer, C. Huber and R. Ahlrichs, J. Chem. Phys., 1994, 100, 5829–5835 CrossRef PubMed
.
-
IUPAC, Compendium of Chemical Terminology (the “Gold Book”), Blackwell Scientific Publications, Oxford, 2nd edn, 1997 Search PubMed
.
- Y. Segawa, H. Omachi and K. Itami, Org. Lett., 2010, 12, 2262–2265 CrossRef CAS PubMed
.
- A. D. Becke, J. Chem. Phys., 1993, 98, 5648–5652 CrossRef CAS PubMed
.
- P. J. Stephens, F. J. Devlin, C. F. Chabalowski and M. J. Frisch, J. Phys. Chem., 1994, 98, 11623–11627 CrossRef CAS
.
- F. Weigend, F. Furche and R. Ahlrichs, J. Chem. Phys., 2003, 119, 12753–12762 CrossRef CAS PubMed
.
- W. T. Borden and E. R. Davidson, Acc. Chem. Res., 1996, 29, 67–75 CrossRef CAS
.
- G. Haberhauer and R. Gleiter, J. Am. Chem. Soc., 1999, 121, 4664–4668 CrossRef CAS
.
- G. Haberhauer and R. Gleiter, J. Am. Chem. Soc., 2013, 135, 8022–8030 CrossRef CAS PubMed
.
- A. D. Becke, J. Chem. Phys., 1993, 98, 1372–1377 CrossRef CAS PubMed
.
- Y. Zhao and D. G. Truhlar, J. Phys. Chem. A, 2005, 109, 5656–5667 CrossRef CAS PubMed
.
- M. Ernzerhof and G. E. Scuseria, J. Chem. Phys., 1999, 110, 5029–5036 CrossRef CAS PubMed
.
- C. Adamo and V. Barone, J. Chem. Phys., 1999, 110, 6158–6170 CrossRef CAS PubMed
.
- S. Grimme, J. Chem. Phys., 2006, 124, 034108 CrossRef PubMed
.
- L. Goerigk and S. Grimme, Phys. Chem. Chem. Phys., 2011, 13, 6670–6688 RSC
.
- G. Zhang and C. B. Musgrave, J. Phys. Chem. A, 2007, 111, 1554–1561 CrossRef CAS PubMed
.
- I. Kaur, W. Jia, R. P. Kopreski, S. Selvarasah, M. R. Dokmeci, C. Pramanik, N. E. McGruer and G. P. Miller, J. Am. Chem. Soc., 2008, 130, 16274–16286 CrossRef CAS PubMed
.
Footnotes |
† Electronic supplementary information (ESI) available: Computational details including total energies, Cartesian coordinates, and geometrical parameters. See DOI: 10.1039/c5cp00341e |
‡ Current address: Institut für Organische Chemie, Albert-Ludwigs-Universität Freiburg, Albertstr. 21, 79104 Freiburg, Germany. E-mail: E-mail: besser@oc.uni-freiburg.de. |
|
This journal is © the Owner Societies 2015 |