DOI:
10.1039/C4AN01491J
(Paper)
Analyst, 2015,
140, 149-155
Oligopeptide–heavy metal interaction monitoring by hybrid gold nanoparticle based assay
Received
13th August 2014
, Accepted 10th October 2014
First published on 10th October 2014
Abstract
Phytochelatins are small peptides that can be found in several organisms, which use these oligopeptides to handle heavy metal elements. Here, we report a method for monitoring interactions between lead(II) ions in aqueous solutions and phytochelatin 6 oligopeptide bioconjugated onto pegylated gold nanorods (PEG-AuNrs). This study is the first step towards a high sensitive label free optical biosensor to quantify heavy metal pollution in water.
1. Introduction
Nanostructured materials have become increasingly popular due to their unique properties as well as their promising breakthrough in the development of novel biosensors for medical diagnostics and environmental monitoring.1–3 In recent years, gold nanoparticles have been widely applied in biosensor devices due to their unique size-dependent optical properties.4–6 Therefore, more attention should be paid to find efficient synthesis methods to match the enlarging demand for gold nanoparticles (AuNPs). Several different solution synthesis methods have been employed to prepare gold nanoparticles, including biomolecule reduction of HAuCl4,7 seed mediated synthesis at room temperature8 and polymer-assisted synthesis.9 Recently, the utility of nanomaterials for any application has been strongly dependent upon their physicochemical characteristics and their interactions with surface modifiers. Let us recall the importance of stabilizers, used in the synthesis of nanoparticles, not only to protect particles against aggregation but also to control their functional properties. Biver et al.10 synthesized Au nanoparticles using thioalkylatedoligoethylene glycols and functionalized them with various fluorescent Acridine Orange derivatives.11 Exchange of organic molecules on Au nanoparticles with PEG can indeed be performed to prepare biocompatible PEG-stabilized Au nanoparticles.12 Wang et al. synthesized a polyethylene glycol (PEG)-modified gold nanoparticle complex by a one-step reaction synchrotron X-ray irradiation method.13 A low concentration of unmodified PEG macromolecules is very important to control the particle size and stabilize gold nanoparticles to demonstrate high stability under realistic biomedical conditions.14 Other approaches were applied to stabilize gold nanoparticles using sulfur-containing polymers, with a possible limitation of their suitability for specific biomedical application. Self-assembly of biomolecular probes with free thiol groups on gold nanoparticle surface is allowed by well-known specific interactions, such as the Au–SH bond, thus avoiding complex chemical procedures for covalent conjugation.15,16 Some studies17–19 showed that gold nanoparticles can interact with specifically sequenced peptides that can self-assemble on their surface. The polypeptides could induce or prevent aggregation of nanoparticles causing consequently the change of absorbance and, moreover, allow them to interact with other metal ions i.e. Cd2+, Ni2+, Co2+, Zn2+, etc. Lead is a widely used heavy metal and has a large number of industrial applications, such as battery manufacturing, paint, gasoline, alloys, radiation shielding, piping and so on. The lead content in paints and gasoline represents a severe risk of environmental pollution and, consequently, for human health. Lead is toxic by ingestion and inhalation, and can seriously affect the gut and the central nervous system, and it can also cause anemia.20,21 Furthermore, overexposure to lead can also cause birth defects, mental retardation, behavioral disorders, and death in fetuses and young children.22,23 Beside social impacts, detection and quantization of lead contamination is not an easy task since water is a complex matrix and any method proposed should be not only sensitive but also highly selective: the lead content should be determined in the presence of lot of interference substances, without any pre-treatment of the collected sample. This is the reason why bioprobes having high specific affinity with this metal must be used. Some oligopeptides, named phytochelatins (PCs), with a structural relationship to glutathione (γ-Glu–Cys–Gly) have been widely studied because of their ability to chelate heavy metal ions in plants and fungi for detoxification mechanisms.24,25 PCs are formed by dipeptide γ-Glu–Cys repeated from 2 to 11 times followed by a final Gly, so that their general structure is (γ-Glu–Cys)nGly. We report here the synthesis to prepare polymer-modified gold nanoparticles and gold nanorods using dicarboxylic PEG (DPEG) as the stabilizer. We have thus implemented and evaluated a simple and reproducible method for labeling biomolecules with PEG gold nanostructures without utilizing organic solvents and surfactants. A new kind of pegylated gold nanorod based assay to quantify lead–phytochelatin 6 (PC6) interactions in aqueous solutions by using oligopeptides as bioprobes was developed. Ultraviolet-visible (UV-Vis) spectroscopy and Fourier transform surface plasmon resonance (FT-SPR) have been used for monitoring the formation of metal–biological complexes at different concentrations of lead.
2. Experimental
2.1. Materials
Tetrachloroauric acid (HAuCl4), sodium borohydride (NaBH4), ethanol (C2H5OH), polyethylene glycol 600 Diacid (DPEG; Mw = 600 Da), cetyl-trimethyl ammonium bromide (CTAB), β-mercaptoethylamine (cysteamine), N-hydroxysuccinimide (NHS), 1-(3-dimethylaminopropyl)-N′-ethylcarbodiimide hydrochloride (EDC), PBS (phosphate buffer solution, pH 7.2), and lead(II) methanesulfonate solution were purchased from Sigma Aldrich. Phytochelatin 6 (PC6) was purchased from the Anaspec IGT group. All chemicals were used without any further purification. Gold substrates for FT-SPR measurements were deposited at IMM-CNR in Lecce (Italy).
2.2. Synthesis of pegylated Au nanorods (PEG-AuNRs)
The synthesis of pegylated gold nanorods (PEG-AuNRs) was performed following the well established seed-mediated procedure, in the presence of CTAB and DPEG in growth solution. Seed solution (S): seed particles were prepared following the method described elsewhere.26,27 Briefly, 5 mL of CTAB (0.20 mol L−1) were added to 5 mL of an aqueous solution containing HAuCl4 (2.5 × 10−4 mol L−1) under stirring conditions at room temperature. 0.6 mL of ice-cooled NaBH4 (0.01 mol L−1) was then added. Growth solution (G): growth solution was prepared by adding 5 mL of CTAB (0.02 mol L−1) to a solution of 0.75 mL of DPEG and under stirring for 10 min at room temperature. After 10 min 0.25 mL AgNO3 (4 × 10−3 mol L−1) and 5 mL of HAuCl4 (1 × 10−3 mol L−1) were transferred to the mixture for 5 min. After this time, 70 μl of ascorbic acid (8 × 10−3 M) was added. The synthesis of PEG hybrid gold nanorods (AuNrs) was achieved as follows: 12 μl of gold seed solution (S) was transferred to the growth solution (G) under room temperature conditions.
2.2.1. Synthesis of pegylated Au nanoparticles (PEG-AuNPs).
Li et al.28 have reported a facile method to synthesize AuNPs from concentrated chloroauric acid by adding sodium hydroxide in the presence of citrate as the stabilizer. We modified this protocol by adding dicarboxylic PEG as the surfactant, in the mixture reaction. Briefly, 25 mL of chloroauric acid (HAuCl4) aqueous solution (2.5 × 10−4 M) was added to 0.25 mL of dicarboxylic PEG and mixed under magnetic stirring for 10 min at room temperature. To this solution, 20 mL of aqueous 0.01 M NaBH4 was added at once. The formation of the PEG-AuNPs was observed as an instantaneous color change of the solution from pale yellow to bright red after addition of the reducing agent. The as-prepared PEG-AuNP solution was centrifuged at 15
000 rpm for 26 min three times and then the supernatant was discarded and the residue was redispersed in an equivalent amount of buffer solution (PBS pH: 7). This was repeated twice principally to remove the excess dicarboxylic PEG. Stock solutions were stored at 27–29 °C and characterized using UV-Vis spectroscopy and transmission electron microscopy (TEM).
2.2.2. Bioconjugation of PEG-AuNrs and PEG-AuNPs with PC6.
The gold nanorod surface was modified with PC6 peptides according to the following procedure (see Scheme 2). 0.5 mL of the PEG-AuNrs and PEG-AuNPs in a buffered solution (PBS, pH: 7.2) were added into separate tubes containing 0.5 mL of PC6 (0.7 μM). Next, the AuNr–AuNP/PC6 suspension was centrifuged twice at 6000 rpm for 20 min to remove the excess protein and then the pellets were redispersed in 1 mL MilliQ water. The resultant colloidal solution was sonicated for 5 min and then stirred for 1 h at room temperature. Scheme 1 depicts the bioconjugation of gold nanospheres and nanorods.
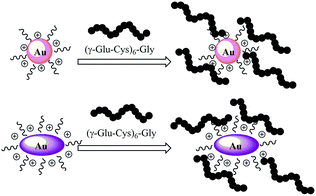 |
| Scheme 1 Ionic interaction mechanism of PC6 protein grafting onto gold nanospheres (above) and gold nanorods (below). | |
2.2.3. PC6 immobilization on gold substrates.
For PM-IRRAS analyses, glass substrates (11 × 11 mm2), successively coated with a 5 nm thick layer of chromium and a 200 nm thick layer of gold, were purchased from Arrandee (Werther, Germany). The gold-coated substrates were annealed in a butane flame to ensure good crystallinity of the topmost layers and rinsed in a bath of absolute ethanol for 15 min before use. Chemistry procedures based on SAMs of β-mercaptoethylamine (cysteamine) in absolute ethanol have been described previously.29 Briefly, the freshly cleaned gold substrate was immersed in an unstirred 10 mM ethanol cysteamine solution at room temperature, in the dark, for 6 h. The gold substrate was then washed with ethanol and ultrapure water (Milli-Q, Millipore, France) to remove the excess thiols. The cysteamine-modified gold substrates were immersed in EDC/NHS (80 mg/20 mg)-PC6 and PC6-AuNr solutions for 1 h and then rinsed with phosphate buffer solution and MilliQ water three times for 5 minutes.
2.2.4. Lead(II) detection.
The interaction between PC6-AuNrs and lead(II) solutions was followed using UV-Vis spectra (50 μL lead solution added to 1 mL PC6-AuNr solution to obtain final lead concentrations of 100, 50 and 25 ppb) and FT-SPR shifts of PC6-modified gold substrates.
2.3. Instrumentation
2.3.1. UV/Vis measurements.
Absorption spectra were recorded using a Jasco V-570 UV/VIS/NIR Spectrophotometer from Jasco Int. Co. Ltd., Tokyo, Japan in the 200–800 nm range.
2.3.2. TEM imaging.
Transmission electron microscopy measurements were recorded on a JEOL JEM 1011 microscope operating at an accelerating voltage of 100 KV. The TEM graphs were taken after separating the surfactant from the metal particles by centrifugation. Typically 1 mL of the sample was centrifuged for 20 min at a speed of 14
000 rpm min−1. The upper part of the colourless solution was removed and the solid portion was re-dispersed in 1 ml of water. 2 μL of this redispersed particle suspension was placed on a carbon coated copper grid and dried at room temperature.
2.3.3. PM-IRRAS.
PM-IRRAS spectra were recorded on a commercial Thermo (Les Ulis-France) Nexus spectrometer. The external beam was focused on the sample with a mirror, at an optimal incident angle of 80°. A ZnSe grid polarizer and a ZnSe photoelastic modulator, modulating the incident beam between p- and s-polarizations (HINDS Instruments, PEM 90, modulation frequency = 37 kHz), were placed prior to the sample. The light reflected at the sample was then focused onto a nitrogen-cooled MCT detector. The presented spectra resulted from the sum of 128 scans recorded at a 8 cm−1 resolution.
2.3.4. FT-SPR.
FT-SPR measurements were performed with an SPR 100 module from thermo equipped with a flow cell mounted on a goniometer. It was inserted in a Thermo-scientific Nexus FT-IR spectrometer using a near-IR tungsten halogen light source. The incidence angle was adjusted to have minimal reflectivity located at 9500 cm−1, at the beginning of each experiment, so as to be in the best sensitivity region of the InGaAs detector. Interactions between nanostructured surfaces and lead solution were carried out in the test chamber (10 μL min−1; T = 27 °C).
3. Results and discussion
3.1. Synthesis of PEG-AuNps and PEG-AuNrs
It is well established that PEG-functionalized AuNPs have an increased stability in aqueous and biological media with respect to simple gold nanoparticles.26,30 In order to improve the biointerfacial properties of AuNPs, we coated them with a bifunctional PEG linker carrying two carboxylic groups. The synthesis of PEG-capped gold nanoparticles (DPEG-AuNPs) was achieved by reducing-tetrachloroauric acid (HAuCl4) with sodium borohydride (NaBH4) in the presence of PEG-diacid as a capping agent. The main difference with other synthesis procedures of DPEG-AuNPs is that PEG-diacid is used in the same way as the citrate for the stabilization of the particles through electrostatic interactions between the carboxylic acid groups and the gold surface.28 Particle formation and growth were tuned by the amphiphilic character of the PEG-diacid polymer and include three steps: (1) reduction of HAuCl4 facilitated by dicarboxylic acid-terminated PEG to form gold clusters; (2) adsorption of PEG diacid molecules on the surface of the gold clusters and reduction of metal ions in that vicinity; and (3) growth of gold particles and colloidal stabilization by PEG polymers. The TEM picture of PEG-AuNPs highlights well and monodisperse Au nanospheres with a mean size of 7.2 nm and with a standard deviation of 2 nm (Fig. 1 (left)). The growth of gold nanostructures, synthesized by a seed-mediated procedure, is known to be strongly dependent on the seed nanocrystal structure, the latter being influenced by the nature of surfactants.31 In the present study, the influence of PEG diacid molecules on the growth nanostructures was investigated. Based on the literature, the protocol conducted in the absence of PEG diacid at any step is expected to lead to the formation of gold nanorods.26,32
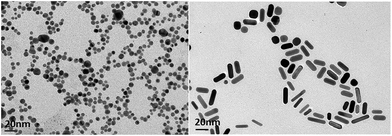 |
| Fig. 1 Transmission Electronic Microscopy images of AuNPs (left) and AuNrs (right). | |
Fig. 1 (right) reports TEM images which confirm the morphology and the remarkable dispersion of the nanorods, with a typical diameter of ca. 5 nm and a length of ca. 20 nm. Fig. 2 displays the LSPR bands of AuNrs: the UV-Vis spectrum shows a strong resonance band at around 780 nm corresponding to the longitudinal plasmon oscillation, and a weaker one at ca. 530 nm corresponding to the transverse plasmon oscillation band confirming the presence of elongated gold nanorods, well isolated from each other, which is in agreement with previous published findings.33 In a remarkable review, Pèrez-Juste et al., have clarified the role of CTAB concentration and temperature upon the nanoparticle aspect ratio, based on a large set of experimental results.33 In particular, these authors proposed that the binding of Au ions, and thus the growth of Au nanorods in aqueous surfactant solution, is controlled by the electric field around the CTAB micelles.34 In the present study, CTAB present in the seed solution is supposed to be in the micellized form, as the concentration used is significantly higher than the critical micelle concentration, reported to be equal to 0.92 mM.35 When PEG diacid is added in the growth solution, leading to the formation of a presumable CTAB–PEG diacid complexes, the same reduction process involving ascorbic acid may occur. Further reduction of Au can then proceed via an electron transfer at the surface of electron-rich, CTAB–PEG diacid capped, seed particles. The rate of hybrid NP formation depends in this case on the reaction of the AuCl2−–CTAB–PEG diacid complex with CTAB–PEG diacid capped seed particles that confer more stability in aqueous medium.
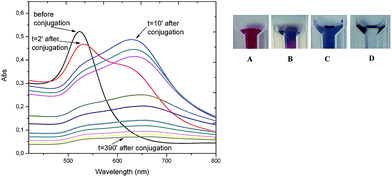 |
| Fig. 2 On the left: UV-Vis spectra of PC6-modified AuNPs as a function of time; on the right: images of AuNPs color changing during bioconjugation. | |
3.2. PC6 adsorption onto pegylated gold nanostructures (PEG-AuNPs and PEG AuNrs)
Interactions of PC6 biomolecules with gold nanoparticles (PEG-AuNPs) or nanorods (PEG-AuNrs) were monitored by observing the Localized Surface Plasmon (LSP) band in the UV-Vis spectrum. Fig. 2 displays the LSP bands of AuNPs before and during interactions with PC6 molecules at equal concentrations of AuNPs in aqueous solution (10−4 M). It is well visible that AuNPs before adsorption show one peak at 530 nm and their water solution has a typical red-rose color (Fig. 2A), while after 2 minutes of mixing with PC6 peptides, a strong color change can be clearly observed (Fig. 2B and C), which corresponds to the appearance of a second LSP peak around 650 nm. UV-Vis spectra were recorded up to 390 minutes after PC6 molecule interactions but just after 120 minutes when the hybrid biological–metal complex becomes instable, since the solution turns transparent (Fig. 2D), due to agglomeration and sedimentation of nanoparticles. Fig. 3 shows the variation of absorption peaks of PEG-AuNrs before and after binding with PC6.
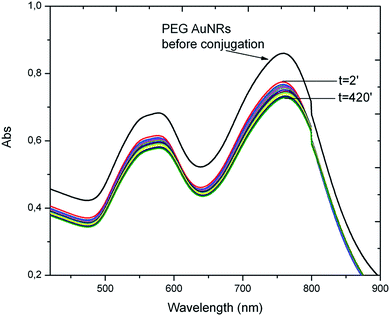 |
| Fig. 3 UV-Vis spectra of AuNrs before and after bio-conjugation with PC6 protein. | |
These measurements were carried out at room temperature for 18 h. The violet color of PEG-AuNr solution resulting after the interaction of PC6 did not change any more. A decrease of the plasmon absorption can be attributed to the change of localized refractive index near the AuNr surface indicating that PC6 was attached to the AuNr surface. From UV-Vis recorded spectra, it is clear that AuNrs bind PC6 molecules and the resulting complexes are stable since there is not any degradation of the absorption peak. We remark that the stability of PEG-AuNrs is due to the presence of two different capping agents, such as DPEG and CTAB, respectively. After their synthesis, gold nanorods carry positive and negative charges on their surfaces due to strongly adsorbed CTA+–PEG–COO− ions, preferentially along the side surfaces. PC6 may adsorb to the PEG-AuNr sides via electrostatic attractions. As a matter of fact, with positively charged CTAB, PC6 likely interacts via its negative charges, thus exposing neutral, or positively charged, groups towards the external sides. Conversely, on PC6 interacting with the end of nanorods, charges are evenly distributed thus favoring classical Van der Waals inter-phytochelatin interactions. We do not exclude the interaction of COO− groups of DPEG with positive charges of PC6 onto gold nanorods: the mechanism for peptide assembly is probably triggered by electrostatic interactions between the deprotonated DPEG and the positively charged CTAB surfactant bilayer on the surface of gold nanorods, as a consequence of the improvement of AuNr stability. A better stability and evaluation of PEG-AuNr bioconjugation was confirmed by PM-IRRAS, which is particularly useful in the case of a very thin layer of modified materials. Planar gold surfaces were functionalized by a cysteamine self-assembled monolayer, which could strongly bound, under EDC/NHS chemistry activation, PC6 in one sample, and PC6-AuNr in another sample, for comparison purposes (see Scheme 2).
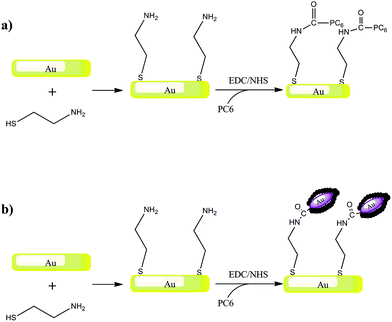 |
| Scheme 2 Schematic representation of the biosensor elaboration strategy: Au surface modification with cysteamine self-assembled monolayer and PC6 (a) or PC6-modified gold nanorods (b). | |
Fig. 3 shows a set of PM-IRRAS data taken after protein immobilization on the gold surface (Fig. 3 black line) and after PC6 conjugated PEG-AuNr immobilization on the gold surface (Fig. 4 redline). The PEG spectral pattern is confirmed by the vibration band of the (C–O–C) at 1020 cm—1; the C
O stretching mode of the carboxylic group expected at 1725 cm−1 is evidenced. The peaks at 1420 cm−1 were attributed to the asymmetric and symmetric C–H scissoring vibrations of CH3–N+ moieties and to the CH2 scissoring mode, respectively. In PM-IRRAS spectra, the peak at 1750 cm−1 is assigned to ester bonds, as a consequence of EDC/NHS activation, the peak at 1660 cm−1 is assigned to the amide II bonds and the one at 1530 cm−1 is assigned to the amide I bonds: in both cases all the peaks are comparable, except the peak at 1020 cm−1, characteristic of the C–O–C chain of PEG that confirms a good interaction between PC6 and PEG-AuNrs.
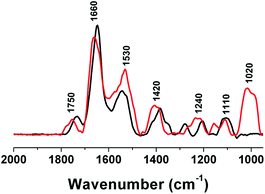 |
| Fig. 4 PM-IRRAS spectra of PC6-modified gold substrates (black line) and PC6-AuNR-modified gold substrates (red line). | |
3.3. Interaction of PC6-AuNRs with lead(II) ions
FT-SPR measurements were used in monitoring interactions between PC6-AuNRs and lead(II) ions. Fig. 5A shows the real-time monitoring of all Pb(II) solutions with different concentrations tested. At the instant t = 64 min, a solution of lead ions with 100 ppb concentration was injected into the test flow chamber, producing a shift of the FT-SPR peak absorption to 8650 cm−1; then an aqueous solution was flown in order to wash the SPR surface, and the FT-SPR signal was restored to its starting value at 9320 cm−1. At t = 93 min, a solution of lead ions at 50 ppb concentration was injected, producing a shift of FT-SPR up to 9050 cm−1; then aqueous solution was flown again to rinse the surface. At t = 115 min, a solution of lead ions at 25 ppb concentration was injected yielding a FT-SPR wavenumber value of about 9100 cm−1; finally aqueous solution was flown to rinse the surface. It is of particular interest that after rinsing the signal restores its initial value since this means that the interaction between nanocomplexes and metal ions is reversible. These wavenumber shifts indicate that lead(II) ions in solution significantly bind to phytochelatin modified AuNrs even if the hybrid probes are conjugated to the analysis surface of FT-SPR. Fig. 5B reports how the absolute position of the plasmon absorbance peak changes as a function of different concentrations of lead ion solution, while Fig. 5C shows data obtained from relative shifts of the plasmon peak during exposition to Pb(II) ion solutions. These data could be fitted using OriginLab Software™ by the following equation (Boltzmann model):
y = A2 + ((A1 − A2)/(1 + [e]^((x − x0)/dx))) |
where A1 is the initial value, A2 is the final value, x0 is the inflection point and dx is the lead concentration constant. The inflection point is useful for the evaluation of the affinity which quantifies how strong is the biomolecular interaction between PC6-AuNrs and lead(II) ions in aqueous solutions: in our case, the x0 value is 73.6 ± 0.9 cm−1 ppb−1 corresponding to 1.8 × 10−10 ± 2 × 10−12 cm−1 mol L−1.
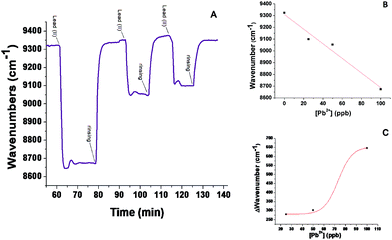 |
| Fig. 5 (A) FT-SPR response as a function of lead(II) solution concentrations (respectively 100, 50 and 25 ppb) during 3 cycles of binding followed by rinsing; (B) SPR transmittance peak position by increasing concentrations of lead solutions; (C) SPR transmittance peak shift as a function of lead(II) solution concentrations. | |
Conclusions
In this work, we have characterized by optical, label free techniques the interaction between small peptides, namely PC6, and Pb(II) in aqueous solutions based on peptide adsorbed gold nanorods. These hybrid nanocomplexes are stable and biologically active: even if linked by adsorbed-gold interactions on the nanorod surface, the peptides are able to strongly bind the heavy metal ions with an affinity constant in the range of picomolars. The signal changes, i.e. variation of the FT-SPR peak position, are important (more than 200 cm−1) even at a very low concentration (25 ppb) of metal ions: this result is very promising for the development of sensitive and effective nanoparticle-based biosensors for quantifying the Pb(II) ion concentration in water.
Author Contributions
The manuscript was written through contributions from all authors. All authors have given approval to the final version of the manuscript.
Funding Sources
The present work is supported by the Italian National Operative Program PON01_01525 “MONitoraggio Innovativo per le Coste e l'Ambiente marino”.
References
- M. S. Yavuz, Y. Cheng, J. Chen, C. M. Cobley, Q. Zhang, M. Rycenga, J. Xie, C. Kim, K. H. Song, A. G. Schwartz, L. V. Wang and Y. Xia, Gold nanocages covered by smart polymers for controlled release with near-infrared light, Nat. Mater., 2009, 8, 935–939 CrossRef CAS PubMed.
- D. A. Giljohann, D. S. Seferos, W. L. Daniel, M. D. Massich, P. C. Patel and C. A. Mirkin, Gold nanoparticle in Biologie und Medizin, Angew. Chem., 2010, 122, 3352–3366 CrossRef.
- E. E. Bedford, J. Spadavecchia, C.-M. Pradier and F. X. Gu, Surface Plasmon Resonance Biosensors Incorporating Gold Nanoparticles, Macromol. Biosci., 2012, 12, 724–739 CrossRef CAS PubMed.
- E. T. Castellana, R. C. Gamez and D. H. J. Russell, Label-Free Biosensing with Lipid-Functionalized Gold Nanorods, J. Am. Chem. Soc., 2011, 133(12), 4182–4185 CrossRef CAS PubMed.
- N. Xia, Y. Shi, R. Zhang, F. Zhao, F. Liu and L. Liu, Simple, rapid and label-free colorimetric assay for arsenic based on unmodified gold nanoparticles and a phytochelatin-like peptide, Anal. Methods, 2012, 4, 3937–3941 RSC.
- L. Rodríguez-Lorenzo, R. De la Rica, R. A. Álvarez-Puebla, L. M. Liz-Marzán and M. M. Stevens, Plasmonic nanosensors with inverse sensitivity by means of enzyme-guided crystal growth, Nat. Mater., 2012, 11, 604–607 CrossRef PubMed.
- G. Zhenhai, J. Jianhui, Z. Ting and W. J. Daocheng, Preparation of Rhodamine B Fluorescent Poly(methacrylic acid) Coated Gelatin Nanoparticles, Nanomaterials, 2011, 1, 753705 Search PubMed.
- R. J. Nikhil, L. Gearheart and C. J. Murphy, Seed-Mediated Growth Approach for Shape-Controlled Synthesis of Spheroidal and Rod-like Gold Nanoparticles Using a Surfactant Template, Adv. Mater., 2001, 13(18), 1389–1393 CrossRef.
- H. J. Parab, J-H. Huang, T-C. Lai, Y-H. Jan, R-S. Liu, J-L. Wang, M. Hsiao, C-H. Chen, Y-K. Hwu, D. P. Tsai, S-Y. Chuang and J-H. S. Pang, Biocompatible transferrin-conjugated sodium hexametaphosphate-stabilized gold nanoparticles: synthesis, characterization, cytotoxicity and cellular uptake, Nanotechnology, 2011, 22, 395706 CrossRef PubMed.
- T. Biver, N. Eltugral, A. Pucci, G. Ruggeri, A. Schena, F. Secco and M. Venturini, Synthesis, characterization, DNA interaction and potential applications of gold nanoparticles functionalized with Acridine Orange fluorophores, Dalton Trans., 2011, 40, 4190 RSC.
- J. Manson, D. Kumar, B. Meenan and D. Dixon, Polyethylene glycol functionalized gold nanoparticles: the influence of capping density on stability in various media, Gold Bull., 2011, 44, 99 CrossRef CAS PubMed.
- H. Zhao, S. Q. Wu, Q.-H. Yao, G. Xu and Q. Xu, Nanocomposites containing gold nanorods and porphyrin-doped mesoporous silica with dual capability of two-photon imaging and photosensitization, Langmuir, 2010, 26, 14937 CrossRef PubMed.
- C.-J. L. Chang-HaiWang, C.-L. Wang, T.-E. Hua, K. H. L. J. M. Obliosca, Y. Hwu, C.-S. Yang, H.-M. L. Ru-Shi Liu, J.-H. Je and G. Margaritondo, J. Phys. D: Appl. Phys., 2008, 41, 195301 CrossRef.
- Q. Shen, Z. Nie, M. Guo, C. Zhong, B. Lin, W. Li and S. Yao, Simple and rapid colorimetric sensing of enzymatic cleavage and oxidative damage of single-stranded DNA with unmodified gold nanoparticles as indicator, Chem. Commun., 2009, 929–931 RSC.
- Y. L. Tang, F. D. Feng, S. F. He, Y. L. Wang Li and D. B. Zhu, Direct Visualization of Enzymatic Cleavage and Oxidative Damage by Hydroxyl Radicals of Single-Stranded DNA with a Cationic
Polythiophene Derivative, J. Am. Chem. Soc., 2006, 128, 14972–14976 CrossRef CAS PubMed.
- W. Li, Z. Nie, K. He, X. Xu, Y. Li, Y. Huang and S. Yao, Simple, rapid and label-free colorimetric assay for Zn2+ based on unmodified gold nanoparticles and specific Zn2+ binding peptide, Chem. Commun., 2011, 47, 4412–4414 RSC.
- Y. Guo, Z. Wang, W. Qu, H. Shao and X. Jiang, Colorimetric detection of mercury, lead and copper ions simultaneously using protein-functionalized gold nanoparticles, Biosens. Bioelectron., 2011, 26, 4064–4069 CrossRef CAS PubMed.
- M. Zhang, Y.-Q. Liu and B.-C. Ye, Attomolar ultrasensitive microRNA detection by DNA-scaffolded silver-nanocluster probe based on isothermal amplification, Analyst, 2012, 137, 601–607 RSC.
- S. Tong, Lead exposure and cognitive development: Persistence and a dynamic pattern, J. Paediatr. Child Health, 1998, 34, 114–118 CAS.
- H. Lilienthal, G. Winneke and T. Ewert, Effects of lead on neurophysiological and performance measures: animal and human data, Environ. Health Perspect., 1990, 89, 21–25 CrossRef CAS.
-
CDC, Surveillance for elevated blood lead levels among children-United States, 1997–2001, Centers for Disease Control and Prevention MMWR Morb Mortal Wkly Rep., 2003, vol. 53, pp. 1–21 Search PubMed.
- S. J. Rothenberg, A. Poblano and L. Schnaas, Neurodegenerative Diseases and Metal Ions, Met. Ions Life Sci., 2000, 22, 503–510 CAS.
- M. Orita, H. Iwahana, H. Kanazawa, K. Hayashi and T. Sekiya, Detection of polymorphisms of human DNA by gel electrophoresis as single-strand conformation polymorphisms., Proc. Natl. Acad. Sci. U. S. A., 1989, 86, 2766–2770 CrossRef CAS.
- C. S. Cobbett, Phytochelatin biosynthesis and function in heavy-metal detoxification, Curr. Opin. Plant Biol., 2000, 3, 211–216 CrossRef CAS.
- J. Manson, D. Kumar, B. J. Meenan and D. Dixon, Polyethylene glycol functionalized gold nanoparticles: the influence of capping density on stability in various media, Gold Bull., 2011, 44, 99–105 CrossRef CAS PubMed.
- N. R. Jana, L. Gearheart and C. J. Murphy, Seed-Mediated Growth Approach for Shape-Controlled Synthesis of Spheroidal and Rod-like Gold Nanoparticles Using a Surfactant Template, Adv. Mater., 2001, 13, 1389 CrossRef CAS.
- H. J. Parab, J.-H. Huang, T.-C. Lai, Y.-H. Jan, R.-S. Liu, J.-L. Wang, M. Hsiao, C.-H. Chen, Y.-K. Hwu, D. P. Tsai, S.-Y. Chuang and J.-H. S. Pang, Biocompatible transferrin-conjugated sodium hexametaphosphate-stabilized gold nanoparticles: synthesis, characterization, cytotoxicity and cellular uptake, Nanotechnology, 2011, 22, 395706 CrossRef PubMed.
- C. Li, D. Li, G. Wan, J. Xu and W. Hou, Facile synthesis of concentrated gold nanoparticles with low size-distribution in water: temperature and pH controls, Nanoscale Res. Lett., 2011, 6, 440 CrossRef PubMed.
- J. Spadavecchia, J. Moreau, J. Hottin and M. Canva, New cysteamine based functionalization for biochip applications, Sens. Actuators, B, 2009, 143, 139 CrossRef PubMed.
- K. G. Neoh and E. T. Kang, Functionalization of inorganic nanoparticles with polymers for stealth biomedical applications, Polym. Chem., 2011, 2, 747 RSC.
- A. Gole and C. J. Murphy, Seed-Mediated Synthesis of Gold Nanorods:
Role of the Size and Nature of the Seed, Chem. Mater., 2004, 16, 3633 CrossRef CAS.
- B. D. Busbee, S. O. Obare and C. J. Murphy, An Improved Synthesis of High-Aspect-Ratio Gold Nanorods, Adv. Mater., 2003, 15, 414 CrossRef CAS.
- J. Pérez-Juste, I. Pastoriza-Santos, L. M. Liz-Marzan and P. Mul-vaney, Gold nanorods: synthesis, characterization and applications Coord, Chem. Rev., 2005, 249, 1870 Search PubMed.
- C. Li, F. Fan, B. Yin, L. Chen, T. Ganguly and Z. Tian, Au+-cetyltrimethylammonium bromide solution: A novel precursor for seed-mediated growth of gold nanoparticles in aqueous solution, Nano Res., 2013, 6, 29–37 CrossRef CAS.
- J. Mattay, Photochemistry in Microheterogeneous Systems. Von K. Kalyanasundaram, Angew. Chem., 1988, 100, 744 CrossRef.
Footnote |
† All authors contributed equally to this work. |
|
This journal is © The Royal Society of Chemistry 2015 |