DOI:
10.1039/C4AN01433B
(Paper)
Analyst, 2015,
140, 353-357
Graphene oxide amplified fluorescence anisotropy for label-free detection of potassium ion†
Received
5th August 2014
, Accepted 18th October 2014
First published on 21st October 2014
Abstract
Fluorescence anisotropy (FA) has attracted considerable attention, but it has been rarely applied for the detection of small molecules and metal ions because they are too small to induce evident FA changes. Although some mass amplifying strategies have been developed, the recognition probes need to be covalently modified with the fluorescent dyes, which is complex and expensive. To overcome this limitation, a new simple, label-free and cost-effective method for the sensitive detection of potassium ion (K+), by using graphene oxide (GO) as FA enhancer, a G-rich single stranded DNA (ssDNA) as recognition probe and acridine orange (AO) as FA reporting fluorophore, was established in this paper. In the absence of K+, both ssDNA and AO are adsorbed on the surface of GO, and the FA of AO is enhanced greatly because the rotation of AO is coupled with the entire formation. After the addition of K+, the ssDNA self-associates into the G-quadruplex structure. Then, AO can bind with the formed G-quadruplex strongly, keeping away from the surface of GO, and the FA of AO decreases significantly because of the relatively small size of the complex of AO and G-quadruplex. Thus K+ can be detected sensitively in the range of 10 μM–2 mM based on the evidently decreased FA. This method is a further improvement of the previous reported mass amplifying strategies because it does not require any covalent labelling of the recognition probe, and it can be potentially applied for detection of a variety of other targets.
Introduction
Fluorescence anisotropy (FA) is a widespread and attractive method due to its homogeneous and reproducible properties.1 It is only sensitive to the rotational diffusion rate of the fluorophore, which depends on the physical properties and local environments of the fluorophore. Thus, it is sensitive to the mass and size of the rotating body.2 In principle, a small molecule has a smaller FA because of its faster rotation. In contrast, a larger molecule has a larger FA because it rotates slowly.3 As it is a ratiometric method, it is less sensitive to the photobleaching and the fluorescence fluctuation than other fluorescent techniques.4 Therefore, it is very suitable for detection of targets in complex samples. Up to now, FA has been widely applied in studying the protein–protein interactions,5,6 protein–DNA interactions,7–9 antibody–antigen interactions10,11 and molecule–membrane interactions,12,13 and it has also been utilized successfully in the detection of nucleic acids,14 proteins,15–18 and even cancer cells.19 However, FA can not be easily applied for the detection of small molecules and metal ions as they are too small to induce the evident FA changes.
To address the abovementioned limitations, numerous mass amplifying strategies have been developed. Particularly, Yang's group20 and Peyrin's group21 successfully used proteins as FA enhancers for the detection of ATP, cocaine and adenosine based on the specific recognition between the selected proteins and the fluorescent dyes labeled nucleic acid probes. Recently, a variety of nanomaterials, such as gold nanoparticles (AuNPs),3,22–24 SiO2 nanoparticles,25 metal–organic frameworks (MOFs),26,27 carbon nanoparticles,28 carbon nanotubes (CNTs)29,30 and graphene,31,32 have been smartly used to amplify FA for the sensitive detection due to their excellent properties, including large volumes and masses, high thermal stabilities and easy and controllable synthesis and modification. Although these methods have greatly enhanced FA and improved the sensitivity of the small molecules and metal ions detections, most of the recognition probes in these methods need to be covalently modified with the fluorescent dyes, which is complicated and expensive. Therefore, it is still necessary to develop simple and cost-effective FA methods for the small molecules and metal ions detection.
In this study, we developed a new label-free FA method by using graphene oxide (GO) as FA amplifier and potassium ion (K+) as a proof-of-concept target. K+ is an important cation in biology, for example, it can maintain the extracellular osmolarity and regulate the concentration of other ions in the living cells.33 However, it is a challenge to detect K+ selectively because the concentration of K+ and Na+ in blood stream is 3.5–5.3 and 135–145 mM, respectively.34 Here, we use a well-known guanine-rich single stranded DNA (ssDNA), with a sequence of 5′-GGT TGG TGT GGT TGG-3′, as recognition probe, which can fold into a G-quadruplex structure in the presence of K+ specifically.33 It has been proved that the stabilizing ability of K+ to the G-quadruplexes is considerably higher than that of Na+.35,36 Then, acridine orange (AO), a classical fluorescent molecule with a conjugate planar structure, which can bind with G-quadruplex by coordinating the guanosine ligands from outside of the cavity,37 was selected as FA reporting fluorophore. As shown in Scheme 1, in the absence of K+, both the ssDNA and AO were adsorbed on the surface of GO through the electrostatic and π–π stacking interactions.38–40 Thus, high FA can be obtained because the rotation of AO is coupled with the entire formation. In the presence of K+, the ssDNA self-associates into the G-quadruplex structure and binds with AO. Owing to the stronger interaction of AO with G-quadruplex than that with GO and the decreased π–π stacking between the honeycomb surface of GO and the rings of the nucleotide bases of G-quadruplex, the complex of AO and G-quadruplex will keep away from the surface of GO.38,40–42 Therefore, the FA of AO will decrease significantly because of the relatively small size of the complex of AO and G-quadruplex. Thus K+ can be detected sensitively based on the evidently decreased FA.
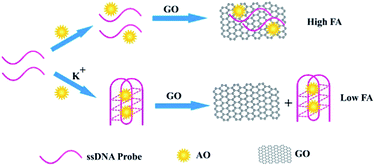 |
| Scheme 1 The concept and principle of GO amplified fluorescence anisotropy for the label-free detection of K+. | |
Experiment section
Materials and instruments
The sequence of the ssDNA probe for K+ recognition is 5′-GGT TGG TGT GGT TGG-3′, which was synthesized and purified through HPLC by Songon Inc (Shanghai, China). GO was purchased from Nanjing XFNANO Materials Tech Inc (Nanjing, China) and suspended in water via sonication. Multi-walled carbon nanotubes (MWCNTs) were purchased from Chengdu Organic Reagent Co. Ltd (Chengdu, China). Acridine orange (AO) was purchased from Sinopharm Chemical Reagent Co., Ltd, China. MOFs (MIL-101) were prepared according to the method discussed in a previously reported study.43 Other metal ions were of analytical reagent grade. Ultrapure water was obtained from a Millipore water (18.0 MΩ cm−1) purification system. Tris–HCl buffer solution (pH 7.4) was used to control the acidity of the reaction solution.
FA was measured using a F-2500 fluorescence spectrophotometer equipped with a polarization filter (Hitachi, Tokyo, Japan). Dynamic light scattering (DLS) measurements were performed using a Zetasizer Nano-ZS90 instrument (Malvern Inc). A vortex mixer QL-901 (Haimen, China) was employed to blend the solution. Circular dichroism (CD) spectra were obtained using a J-810 spectropholarimeter (Jasco, Tokyo, Japan). A constant-temperature water-base boiler (Jiangsu, China) was employed to control the temperature.
Fluorescence anisotropy measurements
Briefly, 10 μL KCl solution with a certain concentration was added to the mixture of 10 μL 2 μM ssDNA probe and 20 μL pH 7.4 Tris–HCl buffer. The mixture was incubated at 95 °C for 5 min, and cooled to room temperature for 30 min. Then AO solution with the final concentration of 1 μM was added and reacted for 2 min. Finally, the GO solution with the final concentration of 12.0 μg mL−1 was added, followed by adding certain amount of ultrapure water to make the final volume of 500 μL, and was incubated for another 10 min at room temperature. Fluorescence anisotropy measurements were carried out on the F-2500 fluorescence spectrophotometer with an excitation wavelength of 485 nm and emission was detected at 530 nm.
The anisotropy, r, of the test solution was calculated by
and
where
I represents the intensity of the fluorescence signal and the subscripts define the orientation H for horizontal and V for vertical of the excitation and emission polarizers,
G is the grating factor of the fluorescence spectrophotometer, which is used to correct the wavelength response to polarization of the emission optics and detectors.
Results and discussion
GO as effective FA amplifier
Firstly, to confirm that GO can be used as FA enhancer for K+ detection, the FA responses in the absence and presence of K+ with/without subsequent incubation with GO was measured. As shown in Fig. 1, in the absence of GO, the change of FA was so small that it would be impossible to detect K+ directly. Although Wang's group has successfully utilized this G-rich ssDNA for the detection of K+ based on the reduction in FA response, the ssDNA needs to be internally labelled with the fluorescent dye.44 Therefore, to avoid the complex and expensive covalent labelling, GO was introduced in this work. It was found that in the presence of GO, the background FA was significantly enhanced to 0.33, which was about 11-fold higher than that without GO. This was due to the electrostatic attraction and the π–π stacking between the negatively charged GO and positively charged AO. After the addition of K+, the conformation of ssDNA was changed to G-quadruplex structure, and AO can bind with the formed G-quadruplex through the coordination of acridine with the guanosine of the K+–G-quadruplex complex from outside,37 keeping away from the surface of GO because of the decreased π–π stacking between the honeycomb surface of GO and the rings of the nucleotide bases of G-quadruplex. Thus the FA was reduced. These experimental results clearly indicate that GO can greatly amplify the reporting signal Δr (Δr = r0 − r, where r0 and r are the FA values in the absence and presence of K+, respectively) by 39 times as it significantly enhances the background FA. To confirm the interactions in the presence of GO, DLS measurements were carried out. The experimental results (Fig. S1†) show that there is only one size distribution of the complex in the absence of K+, which means that both AO and ssDNA are adsorbed on the surface of GO. In the presence of K+, however, there are two size distributions. One is 287.3 nm, which is about 93.1% and corresponds to the large hydrodynamic diameter of GO. The other one is 27.63 nm, about 6.9%, and corresponds to the small hydrodynamic diameter of the complex of G-quadruplex and AO. This result confirms that the decreased FA in the presence of K+ is indeed attributed to the smaller size of the complex of G-quadruplex and AO compared with that of GO, ssDNA and AO.
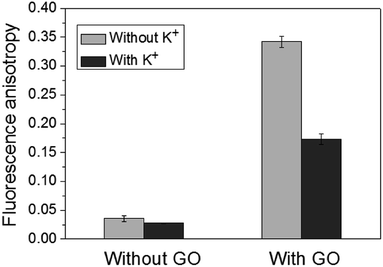 |
| Fig. 1 Fluorescence anisotropy in the absence (light gray columns) and presence (dark gray columns) of K+ with/without the subsequent addition of GO. Concentrations: ssDNA, 40 nM; GO, 12.0 μg mL−1; AO, 1 μM; K+, 2 mM. All data were collected from three measurements, and the error bars indicate the standard deviation. | |
To test the generality of this amplification strategy, other nanomaterials, including MWCNTs and MOFs (MIL-101), were employed. The experimental results (Fig. S2 & S3†) indicated that MWCNTs and MIL-101 with negative charges played a role similar to GO and could adsorb ssDNA and AO through π–π stacking and electrostatic attraction to enhance the FA. After the addition of K+, AO preferred to bind with the formed G-quadruplex and kept away from the surface of MWCNTs and MIL-101, and the measured FA decreased. These results confirmed the versatility of the present method.
Circular dichroism (CD) spectra analysis
To further confirm the reaction mechanism, we investigated the CD spectra of the ssDNA before and after the addition of different concentrations of K+. The experimental results (Fig. 2) showed that after the addition of K+, a positive band near 293 nm and a negative band near 263 nm were formed, which were the characteristics of antiparallel G-quadruplex.45 Furthermore, the intensity of the positive band at 293 nm increased with the increase of the concentration of K+, indicating that more and more G-quadruplex structures were formed. All of these results confirm that K+ can induce the ssDNA to form the G-quadruplex structure in our experimental conditions. Then, AO prefers to interact with the formed G-quadruplex. As a result, AO keeps away from the surface of GO due to the reduced interaction between G-quadruplex and GO, and the FA of AO reduced significantly.
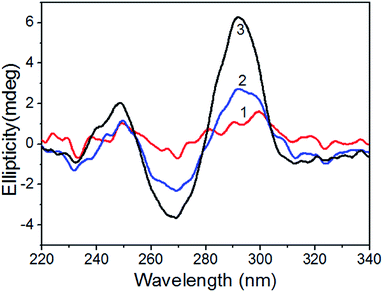 |
| Fig. 2 The CD spectra of ssDNA probe in the absence (1) and presence (2) and (3) of K+. Concentration of ssDNA is 40 nM, and concentrations of K+ is: (1), 0 μM; (2), 1.25 μM; (3), 5 μM. | |
Optimization of the concentration of GO
After confirming the reaction mechanism, we next investigated the influence of the amount of GO on the detection signals. As shown in Fig. 3, when the amount of GO is small, the ssDNA and AO can not be sufficiently installed on the surface of GO, leading to the low background. When the amount of GO is too high, the ssDNA and AO could not be easily released from the surface of GO, giving poor reporting signal. It was found that Δr firstly enhanced with the increased amount of GO and then reached to a maximum when 10.0–12.0 μg mL−1 GO was added. When the amount of GO was higher than 12.0 μg mL−1, Δr decreased evidently. Therefore, 12.0 μg mL−1 was selected as the optimal concentration of GO.
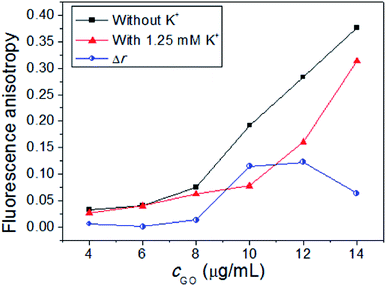 |
| Fig. 3 Fluorescence anisotropy in the absence and presence of the K+ with different concentrations of GO. Concentrations: ssDNA, 40 nM; K+, 1.25 mM; AO, 1 μM. | |
High sensitivity of the K+ detection
To determine the sensitivity of this method, different concentrations of K+ were tested at the optimal conditions and the typical response curve is shown in Fig. 4. There was a good linear relationship between Δr and the concentration of K+ over a wide range of 10 μM–2 mM, and the linear regression equation (cK+, μM) was Δr = 0.030 + 6.142 × 10−5 × cK+ (the regression coefficient R2 = 0.981). The limit of detection (LOD) was estimated to be as low as 1 μM, which was lower than that of the previous reports.45,46 All of these results confirmed that the designed strategy could be applied for the sensitive detection of K+.
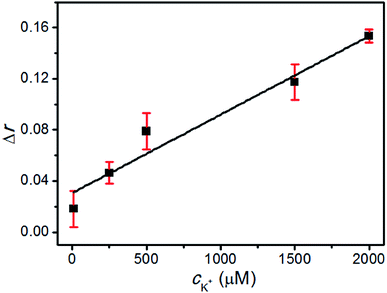 |
| Fig. 4 The linear relationship between Δr and the concentration of K+. Concentrations: ssDNA, 40 nM; GO, 12.0 μg mL−1; AO, 1 μM. All data were collected from three measurements, and the error bars indicate the standard deviation. | |
High selectivity of the K+ detection
Finally, the selectivity of this method was examined by comparing the FA changes of AO brought by K+ with that brought by a variety of monovalent and divalent cations, including Li+, Na+, NH4+, Ni2+, Mn2+ and Co2+ under the same experimental conditions, and the results are shown in Fig. 5. It could be seen that only K+ can induce a significant FA change. The other cations with the same concentrations could not cause the evident FA changes, indicating that these cations were not able to induce the formation of G-quadruplex. Therefore, this method is selective for K+ detection.
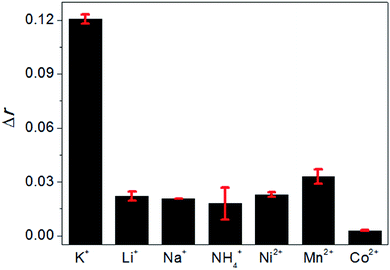 |
| Fig. 5 Selectivity of K+ detection over other cations. Concentrations: ssDNA, 40 nM; GO, 12.0 μg mL−1; AO, 1 μM; cations, 1.5 mM. All data were collected from three measurements, and the error bars indicate the standard deviation. | |
Conclusions
In conclusion, a new label-free method was established by using GO as FA amplifier, a G-rich ssDNA as recognition probe, and AO as FA reporting fluorophore for the sensitive and selective detection of K+. This FA method is a further improvement of the previous reported mass amplifying strategies because it does not require any covalent labelling of the recognition probe. Therefore, it is highly simple and cost-effective. In addition, this method is general. On one hand, other nanomaterials, such as MWCNTs and MOFs, can be used in this system to enhance the FA. On the other hand, it can be easily used to detect a lot of other targets just by substituting the G-rich ssDNA probe for the oligonucleotide that can selectively bind with other targets. Further efforts are currently being made in such directions.
Acknowledgements
This work was supported by the National Natural Science Foundation of China (NSFC, no. 21305113) and the Research Fund for the Doctoral Program of Higher Education of China (young scholars) (RFDP, no. 20120182120017).
Notes and references
- D. M. Jameson and J. A. Ross, Chem. Rev., 2010, 110, 2685–2708 CrossRef CAS PubMed.
- M. E. McCarroll, F. H. Billiot and I. M. Warner, J. Am. Chem. Soc., 2001, 123, 3173–3174 CrossRef CAS.
- B.-C. Yin, P. Zuo, H. Huo, X. Zhong and B.-C. Ye, Anal. Biochem., 2010, 401, 47–52 CrossRef CAS PubMed.
- J. Liu, Z. Cao and Y. Lu, Chem. Rev., 2009, 109, 1948–1998 CrossRef CAS PubMed.
- E. M. Souza-Fagundes, A. O. Frank, M. D. Feldkamp, D. C. Dorset, W. J. Chazin, O. W. Rossanese, E. T. Olejniczak and S. W. Fesik, Anal. Biochem., 2012, 421, 742–749 CrossRef CAS PubMed.
- J.-W. Choi, D.-K. Kang, H. Park, A. J. deMello and S.-I. Chang, Anal. Chem., 2012, 84, 3849–3854 CrossRef CAS PubMed.
- V. Letilly and C. A. Royer, Biochemistry, 1993, 32, 7753–7758 CrossRef CAS.
- M. S. Ozers, J. J. Hill, K. Ervin, J. R. Wood, A. M. Nardulli, C. A. Royer and J. Gorski, J. Biol. Chem., 1997, 272, 30405–30411 CrossRef CAS PubMed.
- K. Wojtuszewski and I. Mukerji, Biochemistry, 2003, 42, 3096–3104 CrossRef CAS PubMed.
- H. Szmacinski, E. Terpetschnig and J. R. Lakowicz, Biophys. Chem., 1996, 62, 109–120 CrossRef CAS.
- S. A. Eremin, I. A. Ryabova, J. N. Yakovleva, E. V. Yazynina, A. V. Zherdev and B. B. Dzantiev, Anal. Chim. Acta, 2002, 468, 229–236 CrossRef CAS.
- F. Jahnig, Proc. Natl. Acad. Sci. U. S. A., 1979, 76, 6361–6365 CrossRef CAS.
- N. Ehrich, A. L. Christensen and D. Stamou, Anal. Chem., 2011, 83, 8169–8176 CrossRef PubMed.
- M. Zhang, Y.-M. Guan and B.-C. Ye, Chem. Commun., 2011, 47, 3478–3480 RSC.
- W. Li, K. Wang, W. Tan, C. Ma and X. Yang, Analyst, 2007, 132, 107–113 RSC.
- G. Gokulrangan, J. R. Unruh, D. F. Holub, B. Ingram, C. K. Johnson and G. S. Wilson, Anal. Chem., 2005, 77, 1963–1970 CrossRef CAS PubMed.
- M. Zou, Y. Chen, X. Xu, H. Huang, F. Liu and N. Li, Biosens. Bioelectron., 2012, 32, 148–154 CrossRef CAS PubMed.
- X. H. Fang, Z. H. Cao, T. Beck and W. H. Tan, Anal. Chem., 2001, 73, 5752–5757 CrossRef CAS.
- T. Deng, J. Li, L.-L. Zhang, J.-H. Jiang, J.-N. Chen, G.-L. Shen and R.-Q. Yu, Biosens. Bioelectron., 2010, 25, 1587–1591 CrossRef CAS PubMed.
- L. Cui, Y. Zou, N. Lin, Z. Zhu, G. Jenkins and C. J. Yang, Anal. Chem., 2012, 84, 5535–5541 CrossRef CAS PubMed.
- Z. Zhu, C. Ravelet, S. Perrier, V. Guieu, E. Fiore and E. Peyrin, Anal. Chem., 2012, 84, 7203–7211 CrossRef CAS PubMed.
- B.-C. Ye and B.-C. Yin, Angew. Chem., Int. Ed., 2008, 47, 8386–8389 CrossRef CAS PubMed.
- Y. Huang, S. Zhao, Z.-F. Chen, Y.-C. Liu and H. Liang, Chem. Commun., 2011, 47, 4763–4765 RSC.
- X. Wang, M. Zou, H. Huang, Y. Ren, L. Li, X. Yang and N. Li, Biosens. Bioelectron., 2013, 41, 569–575 CrossRef CAS PubMed.
- Y. Huang, S. Zhao, Z.-F. Chen, M. Shi and H. Liang, Chem. Commun., 2012, 48, 7480–7482 RSC.
- J. F. Guo, C. M. Li, X. L. Hu, C. Z. Huang and Y. F. Li, RSC Adv., 2014, 4, 9379–9382 RSC.
- J. M. Fang, P. F. Gao, X. L. Hu and Y. F. Li, RSC Adv., 2014, 4, 37349–37352 RSC.
- J. Liu, J. Yu, J. Chen, R. Yang and K. Shih, Mater. Sci. Eng., C, 2014, 38, 206–211 CrossRef CAS PubMed.
- Y. Huang, M. Shi, L. Zhao, S. Zhao, K. Hu, Z.-F. Chen, J. Chen and H. Liang, Biosens. Bioelectron., 2014, 54, 285–291 CrossRef CAS PubMed.
- Y. Huang, K. Hu, S. Zhao, M. Li, Z.-F. Chen, Q. Lv and H. Liang, Chem.–Asian J., 2014, 9, 87–92 CrossRef CAS PubMed.
- Y. Yu, Y. Liu, S. J. Zhen and C. Z. Huang, Chem. Commun., 2013, 49, 1942–1944 RSC.
- J. Liu, C. Wang, Y. Jiang, Y. Hu, J. Li, S. Yang, Y. Li, R. Yang, W. Tan and C. Z. Huang, Anal. Chem., 2013, 85, 1424–1430 CrossRef CAS PubMed.
- F. He, Y. L. Tang, S. Wang, Y. L. Li and D. B. Zhu, J. Am. Chem. Soc., 2005, 127, 12343–12346 CrossRef CAS PubMed.
- J. Lee, H.-J. Kim and J. Kim, J. Am. Chem. Soc., 2008, 130, 5010–5011 CrossRef CAS PubMed.
- D. M. Kong, Y. E. Ma, J. H. Guo, W. Yang and H. X. Shen, Anal. Chem., 2009, 81, 2678–2684 CrossRef CAS PubMed.
- A. De Cian, L. Guittat, M. Kaiser, B. Sacca, S. Amrane, A. Bourdoncle, P. Alberti, M.-P. Teulade-Fichou, L. Lacroix and J.-L. Mergny, Methods, 2007, 42, 183–195 CrossRef CAS PubMed.
- N. Nagesh and A. Krishnaiah, J. Biochem. Biophys. Methods, 2003, 57, 65–74 CrossRef CAS.
- Y. Shi, W. T. Huang, H. Q. Luo and N. B. Li, Chem. Commun., 2011, 47, 4676–4678 RSC.
- L. Liu, W. Liu, T. Hong, X. Weng, Q. Zhai and X. Zhou, Anal. Methods, 2012, 4, 1935–1939 RSC.
- W. T. Huang, J. R. Zhang, W. Y. Xie, Y. Shi, H. Q. Luo and N. B. Li, Biosens. Bioelectron., 2014, 57, 117–124 CrossRef CAS PubMed.
- H. Wang, T. Chen, S. Wu, X. Chu and R. Yu, Biosens. Bioelectron., 2012, 34, 88–93 CrossRef CAS PubMed.
- X.-J. Xing, Y. Zhou, X.-G. Liu, H.-W. Tang and D.-W. Pang, Analyst, 2013, 138, 6301–6304 RSC.
- J. M. Fang, F. Leng, X. J. Zhao, X. L. Hu and Y. F. Li, Analyst, 2014, 139, 801–806 RSC.
- D. Zhang, H. Shen, G. Li, B. Zhao, A. Yu, Q. Zhao and H. Wang, Anal. Chem., 2012, 84, 8088–8094 CrossRef CAS PubMed.
- T. Li, E. Wang and S. Dong, Anal. Chem., 2010, 82, 7576–7580 CrossRef CAS PubMed.
- X. Zhu, J. Zhao, Y. Wu, Z. Shen and G. Li, Anal. Chem., 2011, 83, 4085–4089 CrossRef CAS PubMed.
Footnote |
† Electronic supplementary information (ESI) available. See DOI: 10.1039/c4an01433b |
|
This journal is © The Royal Society of Chemistry 2015 |
Click here to see how this site uses Cookies. View our privacy policy here.