Complexation-induced circular dichroism and circularly polarised luminescence of an aggregation-induced emission luminogen†
Received
19th August 2013
, Accepted 8th October 2013
First published on 9th October 2013
Abstract
We here report a molecule with chiral recognition capability by a mechanism of complexation-induced circularly polarised luminescence (CPL) in the solid thin film state. A molecule (1) containing the luminogenic unit silole and chiral phenylethanamine pendants is synthesized and characterized with standard spectroscopic methods. In a solution of 1, no circular dichroism (CD) or fluorescence emission are observed. When aggregated into nanoparticles in a poor solvent, fluorescence is turned on but CD is still silent. When complexed with chiral acids to form a thin film, molecule 1 becomes CD-active and strongly fluorescent, showing simultaneous complexation-induced CD (CICD) and aggregation-induced emission (AIE) effects. The assemblies of 1 with different enantiomers of mandelic acid emit distinctly handed circularly polarised light and display CPL dissymmetry factors with absolute value around 0.01. This work provides a new platform for creating molecular functional materials with solid-state chiral sensing ability through reading CPL output signals.
Introduction
Compared to standard photoluminescence spectroscopy, circularly polarised luminescence (CPL) spectroscopy, the emission analogue to circular dichroism (CD), is being less reported.1 From the spectroscopic CPL signals, we can obtain significant information about the chirality of materials in the excited states, from which stereochemical, conformational and three-dimensional structure can then be identified.2,3 A typical example is using CPL as a tool to study the electronic structure of radioactive actinides.1 CPL active materials with efficient performances are especially suitable for optoelectronic applications, such as making devices for stereoscopic optical processing, display and storage,4–7 chiral recognition in pharmaceutical industries and biological systems.8 In theory, luminophores arranged helically could produce CPL upon photoexcitation.5 With the selective emission of left- and right-handed circularly polarised (LCP and RCP) light from chiral molecular systems, CPL is characterized by differential spontaneous emission of LCP and RCP upon photo- or electro-excitation [ΔI(λ) = IL(λ) − IR(λ), where IL(λ) and IR(λ) denote the emission intensities of LCP and RCP components, respectively].2 The primary measurement of the degree of CPL, the emission dissymmetry factor gem, is defined as 2(IL − IR)/(IL + IR) which indicates the degree of either one type of circularly polarised light (LCP or RCP) is preferentially emitted over another. The value of gem is between −2 to +2 and its absolute value equals 2 for a pure single-handed material.5
Until now, there are two major chiral systems that have been reported with CPL properties: inorganic systems (lanthanide ion complexes,9,10 transition metal complexes,11–13etc.) and organic systems (small organic molecules,14–17 synthetic polymers18–22 and biomacromolecules4). To promote the real application of these materials, high emission efficiency and spectral stability in the condensed phase are necessary. However, most of the small organic candidates studied were measured in solution and found with only small gem values (∼10−5 to 10−2). The performance of the organic candidates is even worse in the condensed phase because of the aggregation-caused quenching (ACQ) effect.23 Aggregation of chiral luminophores normally makes the excited species dissipate through nonradiative pathways with the formation of excimers or exciplexes induced by π–π stacking interaction.23 Consequently, the emission efficiency, spectral stability and processability are lowered. Our group have previously observed an opposite phenomenon: a group of propeller-shaped π-conjugated molecules, for example, silole and tetraphenylethene, which are weakly or even non-emissive in dilute solutions, are induced to emit intensively by aggregation.24–26 The upside of adopting AIE active materials in CPL are to overcome the serious limitations of ACQ in condensed phase: they are highly emissive in the solid state and thus more practical for real applications; they have better processability—no intricate process is required to interfere with the aggregation process during the fabrication of the solid thin film. Decorating the AIE-active luminogens with chiral moieties may induce the ordered assembly and efficient CPL. Recently, we have proved this idea through attaching the AIE-active luminogen with chiral mannose-like moieties.27 The resultant molecules can self-assemble into right-handed helical nanostructures and give rise to simultaneous aggregation-induced CD (AICD) and a large dissymmetry factor with absolute values of 0.08–0.32 in the film state. The value is about two orders of magnitude higher than those of commonly reported organic materials. The mechanism of that system is mainly based on self-assembly of molecules into a one-handed preferred architecture upon solvent evaporation. The CPL signal obtained from the screw-shaped structure, however, cannot be changed or modified. To expand the system, in this work, we employ a new strategy to produce CPL active material with controllable signals. We synthesize an AIE luminogen with thiourea linkers and chiral phenylethanamine groups (1, Scheme 1). This molecule has no CD or fluorescence signals when molecularly dissolved. Upon aggregation in a poor solvent or fabricated as a thin film in the solid state, 1 can give strong green fluorescence upon photoexcitation, showing classical AIE characteristics but it is still CD-inactive. Due to the potential hydrogen-bonding interaction between the thiourea and carboxylic acid groups, complexation of 1 with specific chiral acids, herein mandelic acid, in the solid state can generate CD and CPL signals in the condensed phase, displaying a unique complexation-induced CD (CICD) characteristic. Interestingly, the predominant CPL can be RCP or LCP emission depending on the enantiomer of mandelic acid added.
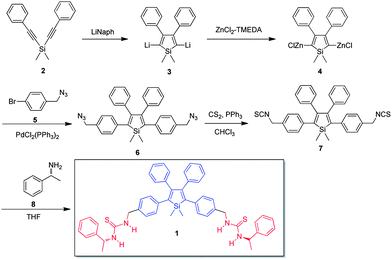 |
| Scheme 1 Synthetic route to chiral AIE-active molecule 1. | |
Results and discussion
Synthesis and structural characterisation
Scheme 1 shows the synthetic route to compound 1, which consists of the AIE luminophore, silole, and chiral peripheral blocks. In the presence of lithium naphthalenide (LiNaph), dimethylbis(phenylethynyl)silane (2) undergoes intramolecular reductive cyclization in an endo–endo mode to form 2,5-dilithiosilole (3), which is transformed to 2,5-dizinc silole (4) by the transmetalation with ZnCl2·TMEDA (TMEDA = N,N,N′,N′-tetramethylethylenediamine). The subsequent reaction of 4 with 4-bromobenzylazide (5) in the presence of palladium catalyst yields the 1,1-dimethyl-2,5-bis[4-(azidomethyl)phenyl]-3,4-diphenylsilole (6), which is further reacted with carbon disulphide to form 1,1-dimethyl-2,5-bis[4-(methylisothiocyanato)phenyl]-3,4-diphenylsilole (7).287 can readily react with chiral phenylethanamine to form a chiral AIE-active molecule (1) through thiourea linker. Compound 1 is characterized by standard spectroscopic techniques including 1H NMR, 13C NMR, and HRMS, from which satisfactory analysis data corresponding to their expected chemical structures are obtained (see Fig. S1–S3 in ESI† and Experimental section for detailed spectroscopic data).
Aggregation-induced emission (AIE)
The molecule 1 is soluble in polar organic solvents such as THF, chloroform, methanol and DMSO but insoluble in nonpolar solvents and water. The THF solution of 1, however, is faintly luminescent when excited at 370 nm (Fig. 1A). The fluorescence remains very weak when up to ∼50 vol% poor solvent, herein water, is added to the solution (Fig. 1B). Afterwards, the fluorescence intensity rises significantly with the increase of water fraction in the mixture. The fluorescence efficiency increases around 40-fold to its maximum intensity at 95 vol% water fraction. Since 1 is insoluble in water, aggregates must have been formed in the solvent mixture with high water fraction, which is further confirmed by particle size analysis (Fig. S4†). This result demonstrates that 1 is AIE-active: it is practically non-emissive when molecularly dissolved in good solvents but emits intensely in the aggregate state. The AIE effect has enabled 1 to emit efficiently in the solid state with a quantum efficiency of the thin film as high as 95%.
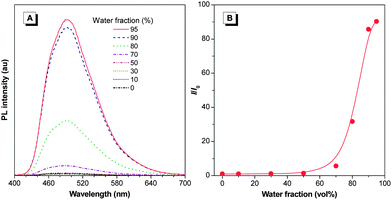 |
| Fig. 1 (A) PL spectra of compound 1 in THF–water mixtures with different water fractions. Excitation wavelength: 370 nm. (B) Plot of I/I0versus water content of a THF–H2O mixture of 1, where I0 denotes the emission intensity in pure THF solution. Concentration of 1: 10−5 M. Excitation wavelength: 370 nm. | |
Complexation-induced circular dichroism
CD spectroscopy measures the differential absorption of LCP and RCP light, [Δε(λ) = εL(λ) − εR(λ), where εL(λ) and εR(λ) denote the molar extinction coefficients of LCP and RCP light, respectively] and reflects the structural information of the ground electronic state of a system.2,3 Since the molecule 1 carries two chiral centres, we are curious about whether the chiral pendants can induce CD signals of the silole core. As shown in Fig. 2A, compound 1 itself, however, has no CD signal in solution at 370 nm, which is corresponding to the absorption band of the silole core (Fig. S5†). The silent CD signals indicate the silole moiety of 1 cannot inherit the chirality from its chiral phenylethanamine pendants, most probably due to the weak intermolecular interaction under such conditions. To enhance intermolecular interaction, we fabricated a thin film of 1 by casting and natural solvent evaporation. However, still no CD signals at the region of silole absorption band could be recorded, further confirming the weak self-assembly ability of 1 into chiral architecture.
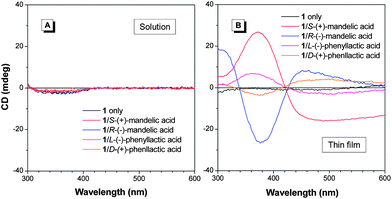 |
| Fig. 2 CD spectra of 1 in the absence and presence of chiral hydroxyl acids in (A) THF solution and (B) solid thin film states. [1] = 1 mM; [acid] = 40 mM. | |
The thiourea bridges of 1, though not favourable for self-assembly, may serve as proton donors in the hydrogen bonding interactions with hydroxy acids. Therefore, we examine whether a chiral hydroxy acid, e.g. mandelic acid, can trigger the chirality of the silole core. As shown in Fig. 2A, in the THF solution, no CD signal of 1 can be recorded at 370 nm even in the presence of enantiopure mandelic acid. Intriguingly, in the condensed phase (i.e. solid thin film state), the addition of a large amount of mandelic acid to 1 (molar ratio 40
:
1) leads to the emergence of the Cotton effects at 370 nm (Fig. 2B). Note that a lower amount of mandelic acid can hardly induce the same effect (Fig. S6†). This new peak, corresponding to the silole absorption band, is associated with chirality transfer from the chiral acids to 1 through the complexation probably between the thiourea groups with the chiral hydroxy acids. Such interactions can induce the silole cores to be helically arranged accordingly. We thus name this phenomenon as a complexation-induced CD (CICD) effect. The CD signals produced by the addition of mandelic acid enantiomers show opposite Cotton effects (Fig. 2B).
In order to understand the structural role of mandelic acids in the CICD effect of 1, we choose a number of chiral acids for comparison (Chart 1). In the solution state, none of these acids can induce the CD signals of 1 (Fig. 2A and S6A†). The peak at the short wavelength (<300 nm region) is referred to the chirality of the acid. For chiral aliphatic acids, such as malic and tartaric acids, there is no new peak found at 370 nm either in solution or solid states, indicating the importance of the phenyl group of mandelic acids in the CICD effect (Fig. 2 and S7†). The silent CD spectrum of 1 with (R)-(−)-1-phenyl-1,2-ethanediol in both solution and solid states suggests the involvement of the carbonyl group of mandelic acids in the CICD effect. In addition to the phenyl and carbonyl groups, control experiments with phenylbutyric acid and dibenzoyl-tartaric acids reveal that both hydroxyl groups in mandelic acid are essential for generating the CICD effect. With a phenyl ring and the two hydroxyl groups, phenyllactic acid is able to induce the CD signals of 1 in the condensed phase (Fig. 2B). However, the presence of one more methylene spacer in its aliphatic chain compared to mandelic acid, greatly reduce the molar ellipticity of 1 at 370 nm. Thus, among the tested chiral acids, mandelic acids are the only acids that can generate the greatest CICD effect of 1 owing to their structural features.
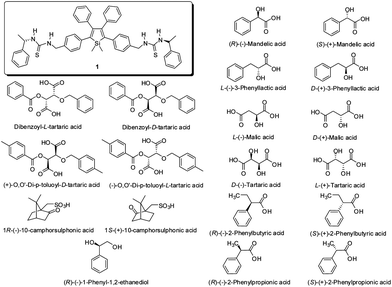 |
| Chart 1 Structures of compound 1 and enantiomeric acids used in this study. | |
Circularly polarised luminescence
The AIE-active molecule 1 exhibits efficient fluorescence and CICD effect in the condensed phase, which prompts us to investigate its CPL behaviour. The home-built epi-illumination (reflection mode) optical system is applied to evaluate the CPL activity through recording the differential spontaneous emission, ΔI(λ) = IL(λ) − IR(λ). The details of the setup have been described in our previous publication.27 This setup is well suited for measuring the chirality of transparent solutions as well as opaque or nontransparent samples which have strong light scattering and low transmittance. Fig. 3 shows the dependence of ΔI and emission dissymmetry factor gemversus the wavelength. Since 1 is AIE-active, it emits weak fluorescence in the solution state and thus we only measure the CPL in its condensed phase. As shown in Fig. 3A, the solid film of 1 alone produces almost no CPL signals. The result, in line with the CD measurement, indicates the conformation of 1 is randomly arranged, together with weak or even no intermolecular interaction to introduce chiral architecture, therefore it shows no CD or CPL signals. In the presence of enantiopure mandelic acid, the ΔI signals become either negative or positive depending on the chirality of the guest acids (Fig. 3B and C). When complexed with R-(−)-mandelic acid, the RCP emission of 1 is dominant over the LCP emission in the whole monitored spectral region, which suggests that R-(−)-mandelic acid can induce the molecules of 1 to align as one-handed helical structures in the solid state. Interestingly, such an arrangement can be altered to the opposite orientation upon complexation with the enantiomeric S-(+)-mandelic acid. The gem values are clearly different, indicative of the distinct packing order in their respective helical assemblies. The gem values of R-(−)-mandelic acid and S-(+)-mandelic acid are about −0.01 and +0.01 on average, respectively, in the detected spectral window of 450–600 nm with little dependence on the emission wavelength.
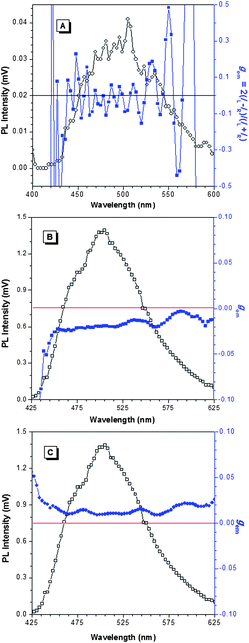 |
| Fig. 3 Plots of PL intensity and CPL dissymmetry factor (gem) versus wavelength for 1 in the (A) absence and (B and C) presence of R-(−) or S-(+)-mandelic acid ([1]/[acid] = 1 : 40 by mole) in the solid film state, respectively. gem = 2(IL − IR)/(IL + IR) and PL intensity = IL + IR, where IL and IR denote the left- and right-handed emission intensity, respectively. Excitation wavelength: 325 nm (0.5 mW). | |
To gain more information on the morphology of the complexes, we perform transmission electron microscopy (TEM) and powder X-ray diffraction (XRD) analysis (Fig. S9 and S10†). The results show that upon complexation with mandelic acids, the morphology of the aggregates of 1 is changed with the emergence of new peaks in the XRD patterns. This implies a higher ordered packing mode has been adopted, mostly induced by the hydrogen-bonding interaction between the thiourea and carboxylic acid groups. The large amount of mandelic acid (40 times higher than 1 in molar ratio) in the solid thin film may crystallize, which generates a chiral field fitted with 1 and induces the silole core to arrange in a helical manner. The presence of 1, however, may in turn cause the non-uniform lattice distortions of the mandelic acid crystals, which is reflected in the broad patterns of XRD.
Conclusions
In this work, a small organic molecule 1 with complexation-induced chirality and CPL behavior has been reported. 1 inherits the AIE characteristics of the silole core: it is nonemissive in the solution state and highly luminescent in the condensed phase. The fluorescence quantum efficiency of 1 in solid state reaches as high as 95%. Although equipped with chiral phenylethanamine moieties, compound 1 can hardly self-assemble, resulting in negligible CD signals in either solution or solid states. Enantio-pure mandelic acid is able to complex with 1 and induces the CD signal of 1 at the silole absorption region, i.e. 370 nm, only in the condensed phase, showing a complexation-induced CD (CICD) effect. This complexation is structurally specific in that other hydroxy and carboxylic acids cannot induce the CD signals of 1 as efficiently as mandelic acids. Combining the AIE and CICD effects, we have then further investigated the CPL performance of the 1/mandelic acid complex in the condensed phase. Under these conditions, the complex generates a CPL dissymmetry factor at an appreciable value. The gem values of R-(−)-mandelic acid and S-(+)-mandelic acid are about −0.01 and +0.01 on average, respectively. These absolute values are much higher than most of the reported organic CPL emitters, which suffer from the aggregation-caused quenching effect. The AIE-active fluorogens thus represent a class of desirable molecules for generating efficient CPL in the solid state. Moreover, whether the predominant CP emission is RCP or LCP emission can be designated upon complexation of 1 with either enantiomer of mandelic acid. Further study on the rational design of the AIE emitters to generate tunable CPL is ongoing in our laboratory.
Experimental section
General information
Tetrahydrofuran (THF) was distilled from sodium benzophenone ketyl immediately prior to use. Dichloromethane (DCM) was distilled over calcium hydride. Lithium wire, naphthalene, dichlorobis(triphenylphosphine)palladium(II), ZnCl2·TMEDA, copper(I) iodide, triphenylphosphine, and other chemicals and solvents were all purchased from Aldrich and used as received without further purification.
1H and 13C NMR spectra were measured on a Bruker ARX 400 NMR spectrometer using methanol-d4 or chloroform-d as solvent with tetramethylsilane (TMS) as internal reference. UV absorption spectra were taken on a Milton Ray Spectronic 3000 array spectrophotometer. CD spectra were recorded on a JASCO J-810 spectropolarimeter in a 1 mm quartz cuvette using a step resolution of 0.1 nm, a scan speed of 200 nm min−1, a sensitivity of 0.5 nm, and a response time of 1 s. Photoluminescence (PL) spectra were recorded on a Perkin-Elmer LS 55 spectrofluorometer. Emission efficiencies of cast thin films of the molecule 1 were measured by a calibrated integrating sphere. High-resolution mass spectra (HRMS) were recorded on a Finnigan MAT TSQ 7000 Mass Spectrometer System operating in a MALDI-TOF mode. Morphologies and structures of the aggregates were investigated by high-resolution JEOL 2010F transmission electron microscopy (TEM). Circular photoluminescence spectra were measured with a home-made CPL spectroscopy system which has been published in a previous paper by our group.26
Material preparation
Preparation of 4-bromobenzylazide (5)28.
Into a flask equipped with a magnetic stirrer were added 4-bromobenzyl bromide (7.5 g, 30 mmol), sodium azide (7.8 g, 120 mmol), and 40 mL of DMSO. After stirring at 70 °C for 12 h, the solution was poured into 150 mL of water and extracted with CH2Cl2. The crude product was purified by silica-gel chromatography using hexane as eluent to give a colorless viscous liquid in 96.2% yield (6.12 g). 1H NMR (CDCl3, 400 MHz), δ (TMS, ppm): 7.47 (d, J = 8.2 Hz, 2H), 7.15 (d, J = 8.2 Hz, 2H), 4.26 (s, 2H). 13C NMR (CDCl3, 100 MHz), δ (TMS, ppm): 134.3, 131.8, 129.6, 122.1, 53.9. HRMS (MALDI-TOF): m/z 210.9640 (M+, calcd 210.9745).
Preparation of 1,1-dimethyl-2,5-bis[4-(azidomethyl)phenyl]-3,4-diphenylsilole (6)28,30,31.
The preparation of 2 was described in ref. 29. A mixture of lithium (0.056 g, 8 mmol) and naphthalene (1.04 g, 8 mmol) in 8 mL of THF was stirred at room temperature under nitrogen for 3 h to form a dark green solution of LiNaph. A solution of 2 (0.52 g, 2 mmol) in 5 mL of THF was then added dropwise to LiNaph solution over 4 min at room temperature. After stirring for 1 h, the mixture was cooled to 0 °C and then diluted with 25 mL THF. A black suspension was formed upon addition of ZnCl2·TMEDA (2 g, 8 mmol). After stirring for an additional hour at room temperature, a solution of 5 (0.89 g, 4.2 mmol) and PdCl2(PPh3)2 (0.08 g, 0.1 mmol) in 25 mL of THF was added. The mixture was refluxed overnight. After cooling to room temperature, 100 mL of 1 M HCl solution was added and the mixture was extracted with DCM. The combined organic layer was washed with brine and water and then dried over magnesium sulfate. After solvent evaporation under reduced pressure, the residue was purified by a silica-gel column using hexane as eluent. The product was obtained as a yellow solid in 57.3% yield. 1H NMR (400 MHz, CDCl3), δ (TMS, ppm): 7.06 (d, J = 8.1 Hz, 4H), 7.01 (m, 6H), 6.92 (d, J = 8.1 Hz, 4H), 6.78 (m, 4H), 4.24 (s, 4H), 0.47 (s, 6H). 13C NMR (100 MHz, CDCl3), δ (TMS, ppm): 154.3, 141.3, 139.9, 138.5, 132.4, 129.9, 129.1, 127.9, 127.5, 126.3, 54.6, −3.9. HRMS (MALDI-TOF): m/z 524.2200 (M+, calcd 524.2145).
Preparation of 1,1-dimethyl-2,5-bis[4-(isothiocyanatomethyl)phenyl]-3,4-diphenylsilole (7)28.
To a solution of 6 (131 mg, 0.25 mmol) and CS2 (0.24 mL, 4.0 mmol) in CHCl3 (1.0 mL) was added PPh3 (131.1 mg, 0.5 mmol) at room temperature. The mixture was stirred for 1.5 h. After solvent evaporation under reduced pressure, the crude product was purified by a silica-gel column using ethyl acetate/hexane (1
:
10 v/v) as eluent. The product was obtained as a yellow solid in 58.6% yield. 1H NMR (400 MHz, CDCl3), δ (TMS, ppm): 7.04 (d, J = 6.4 Hz, 4H, Ar), 7.00 (m, 6H, Ar), 6.93 (d, J = 8.4 Hz, 4H, Ar), 6.79 (m, 4H, Ar), 4.62 (s, 4H, CH2), 0.47 (s, 6H, CH3). 13C NMR (100 MHz, CDCl3), δ (TMS, ppm): 154.5, 141.2, 139.9, 138.4, 131.2, 129.9, 129.2, 127.5, 126.5, 126.4, 48.4, −3.9. HRMS (MALDI-TOF): m/z 556.1462 (M+, calcd 556.1463).
Preparation of 1.
Into a Schlenk tube equipped with a magnetic stirrer were added 0.14 g (0.25 mmol) of 7 and 0.73 mL (0.553 mmol) of 8 in 6 mL of distilled THF protected under nitrogen, the setup was stirred at room temperature for 30 hours. After solvent evaporation under reduced pressure, the residue was purified by a silica-gel column using gradient DCM/hexane (80/20 to 100/0, v/v) mixture as eluent. A yellow solid was obtained in 97.6% yield. 1H NMR (400 MHz, MeOD), δ (TMS, ppm): 7.29–7.27 (m, 4H), 7.22–7.18 (m, 1H), 7.02–6.88 (m, 5H), 6.85–6.83 (d, 2H), 6.78–6.76 (m, 2H), 5.48 (s, 1H), 4.46 (s, 1H), 4.50–4.47 (d, 1H), 1.46–1.44 (d, 3H), 0.40 (t, 3H). 13C NMR (100 MHz, MeOD), δ (TMS, ppm): 183.1, 155.8, 145.3, 142.9, 140.6, 140.2, 137.4, 131.4, 130.3, 129.9, 128.9, 128.4, 127.7, 127.5, 54.7, 31.5, 23.1, −3.50. HRMS (MALDI-TOF): m/z 798.32 (M+, calcd 798.32). The optical rotation of 1 in THF at room temperature is −51.9° (concentration of 1: 0.4 g L−1).
Thin film preparation.
The thin films for CD and CPL measurement were prepared by dissolving 1 and enantiopure chiral acid (1
:
40 molar ratio) in THF. The solution was cast on a quartz plate and solvent was then naturally evaporated.
Acknowledgements
This work was partially supported by the National Basic Research Program of China (973 Program; 2013CB834701), Research Grants Council of Hong Kong (HKUST2/CRF/10 and N_HKUST620/11), and the University Grants Committee of Hong Kong (AoE/P-03/08). B. Z. T. is grateful for the support from Guangdong Innovative Research Team Program of China (201101C0105067115).
Notes and references
- G.-L. Law, C. M. Andolina, J. Xu, V. Luu, P. X. Rutkowski, G. Muller, D. K. Shuh, J. K. Gibson and K. N. Raymond, J. Am. Chem. Soc., 2012, 134, 15545–15549 CrossRef CAS PubMed.
-
(a) F. S. Richardson and J. P. Riehl, Chem. Rev., 1977, 77, 773 CrossRef CAS;
(b) J. P. Riehl and F. S. Richardson, Chem. Rev., 1986, 86, 1 CrossRef CAS.
-
(a) F. C. Spano, Z. Zhao and S. C. J. Meskers, J. Chem. Phys., 2004, 120, 10594 CrossRef CAS PubMed;
(b) F. C. Spano, S. C. J. Meskers, E. Hennebicq and D. Beljonne, J. Chem. Phys., 2007, 129, 7044 CAS.
-
E. Gussakovsky, Circularly polarised luminescence (CPL) of proteins and protein complexes, in Reviews in Fluorescence 2008, ed. C. D. Geddes, Springer, New York, 2010, vol. 2008, pp. 425–459 Search PubMed.
- S. H. Chen, D. Katsis, A. W. Schmid, J. C. Mastrangelo, T. Tsutsui and T. N. Blanton, Nature, 1999, 397, 506 CrossRef CAS PubMed.
- A. Montali, C. Bastiaansen, P. Smith and C. Weder, Nature, 1998, 392, 261 CrossRef CAS PubMed.
- E. Peeters, M. P. T. Christiaans, R. A. J. Janssen, H. F. M. Schoo, H. P. J. M. Dekkers and E. W. Meijer, J. Am. Chem. Soc., 1997, 119, 9909 CrossRef CAS.
-
(a) N. Liu, S. Song, D. Li and Y. S. Zheng, Chem. Commun., 2012, 48, 4908–4910 RSC;
(b) J. Heo and C. A. Mirkin, Angew. Chem., 2006, 118, 955–958 (
Angew. Chem., Int. Ed.
, 2006
, 45
, 941–944
) CrossRef;
(c) D. Yang, X. Li, Y. F. fan and D. W. Zhang, J. Am. Chem. Soc., 2005, 127, 7996–7997 CrossRef CAS PubMed;
(d) L. N. Chi, J. Z. Zhao and T. D. James, J. Org. Chem., 2008, 73, 4684–4687 CrossRef CAS PubMed;
(e) S. Shirakawa, A. Moriyama and S. Shimizu, Org. Lett., 2007, 9, 3117–3119 CrossRef CAS PubMed;
(f) J. Lin, Q. S. Hu, M. H. Xu and L. Pu, J. Am. Chem. Soc., 2002, 124, 2088–2089 CrossRef CAS PubMed;
(g) M. H. Xu, J. Lin, Q. S. Hu and L. Pu, J. Am. Chem. Soc., 2002, 124, 14239–14246 CrossRef CAS PubMed;
(h) Z. B. Li, J. Lin, M. Sabat, M. Hyacinth and L. Pu, J. Org. Chem., 2007, 72, 4905–4916 CrossRef CAS PubMed.
-
(a) J. Yuasa, T. Ohno, K. Miyata, H. Tsumatori, Y. Hasegawa and T. Kawai, J. Am. Chem. Soc., 2011, 133, 9892 CrossRef CAS PubMed;
(b) T. Harada, Y. Nakano, M. Fujiki, M. Naito, T. Kawai and Y. Hasegawa, Inorg. Chem., 2009, 48, 11242 CrossRef CAS PubMed.
-
(a) J. A. Kitchen, D. E. Barry, L. Mercs, M. Albrecht, R. D. Peacock and T. Gunnlaugsson, Angew. Chem., Int. Ed., 2012, 51, 704 CrossRef CAS PubMed;
(b) A. P. S. Samuel, J. L. Lunkley, G. Muller and K. N. Raymond, Eur. J. Inorg. Chem., 2010, 3343 CrossRef CAS PubMed;
(c) M. Seitz, K. Do, A. J. Ingram, E. G. Moore, G. Muller and K. N. Raymond, Inorg. Chem., 2009, 48, 8469 CrossRef CAS PubMed.
- K. D. Oyler, F. J. Coughlin and S. Bernhard, J. Am. Chem. Soc., 2007, 129, 210 CrossRef CAS PubMed.
- S. C. J. Meskers, H. P. J. M. Dekkers, G. Rapenne and J.-P. Sauvage, Chem.–Eur. J., 2000, 6, 2129 CrossRef CAS.
- C. Schaffner-Hamann, A. von Zelewsky, A. Barbieri, F. Barigelletti, G. Muller, J. P. Riehl and A. Neels, J. Am. Chem. Soc., 2004, 126, 9339 CrossRef CAS PubMed.
-
(a) T. Kaseyama, S. Furumi, X. Zhang, K. Tanaka and M. Takeuchi, Angew. Chem., Int. Ed., 2011, 50, 3684 CrossRef CAS PubMed;
(b) J. E. Field, G. Muller, J. P. Riehl and D. Venkataraman, J. Am. Chem. Soc., 2003, 125, 11808 CrossRef CAS PubMed.
- K. E. S. Phillips, T. J. Katz, S. Jockusch, A. J. Lovinger and N. J. Turro, J. Am. Chem. Soc., 2001, 123, 11899 CrossRef CAS PubMed.
-
(a) H. Maeda, Y. Bando, K. Shimomura, I. Yamada, M. Naito, K. Nobusawa, H. Tsumatori and T. Kawai, J. Am. Chem. Soc., 2011, 133, 9266 CrossRef CAS PubMed;
(b) H. Tsumatori, T. Nakashima and T. Kawai, Org. Lett., 2010, 12, 2362 CrossRef CAS PubMed.
-
(a) Y. Imai, K. Kawano, Y. Nakano, K. Kawaguchi, T. Harada, T. Sato, M. Fujiki, R. Kuroda and Y. Matsubara, New J. Chem., 2008, 32, 1110 RSC;
(b) N. Nishiguchi, T. Kinuta, Y. Nakano, T. Harada, N. Tajima, T. Sato, M. Fujiki, R. Kuroda, Y. Matsubara and Y. Imai, Chem.–Asian J., 2011, 6, 1092 CrossRef CAS PubMed;
(c) N. Nishiguchi, T. Kinuta, T. Sato, Y. Nakano, H. Tokutome, N. Tajima, M. Fujiki, R. Kuroda, Y. Matsubara and Y. Imai, Chem.–Asian J., 2012, 7, 360 CrossRef CAS PubMed.
- K. Watanabe, T. Sakamoto, M. Taguchi, M. Fujiki and T. Nakano, Chem. Commun., 2011, 47, 10996 RSC.
-
(a) H. Hayasaka, T. Miyashita, K. Tamura and K. Akagi, Adv. Funct. Mater., 2010, 20, 1243 CrossRef CAS;
(b) H. Goto and K. Akagi, Angew. Chem., Int. Ed., 2005, 44, 4322 CrossRef CAS PubMed.
- S. Fukao and M. Fujiki, Macromolecules, 2009, 42, 8062 CrossRef CAS.
- Y. Geng, A. Trajkovska, S. W. Culligan, J. J. Ou, H. M. P. Chen, D. Katsis and S. H. Chen, J. Am. Chem. Soc., 2003, 125, 14032 CrossRef CAS PubMed.
- A. Satrijo, S. C. J. Meskers and T. M. Swager, J. Am. Chem. Soc., 2006, 128, 9030 CrossRef CAS PubMed.
-
J. B. Birks, Photophysics of Aromatic Molecules, Wiley, London, 1970 Search PubMed.
- Y. Hong, J. W. Y. Lam and B. Z. Tang, Chem. Soc. Rev., 2011, 40, 5361 RSC.
- Y. Hong, J. W. Y. Lam and B. Z. Tang, Chem. Commun., 2009, 4332 RSC.
- J. Luo, Z. Xie, J. W. Y. Lam, L. Cheng, H. Chen, C. Qiu, H. S. Kwok, X. Zhan, Y. Liu, D. Zhu and B. Z. Tang, Chem. Commun., 2001, 1740 RSC.
- J. Liu, H. Su, L. Meng, Y. Zhao, C. Deng, J. C. Y. Ng, P. Lu, M. Faisal, J. W. Y. Lam, X. Huang, H. Wu, K. S. Wong and B. Z. Tang, Chem. Sci., 2012, 3, 2737–2747 RSC.
- Y. Yu, J. Liu, Z. Zhao, K. M. Ng, K. Q. Luo and B. Z. Tang, Chem. Commun., 2012, 48, 6360–6362 RSC.
- J. Liu, Y. Zhong, J. W. Y. Lam, P. Lu, Y. Hong, Y. Yu, Y. Yue, M. Faisal, H. H. Y. Sung, I. D. Williams, K. S. Wong and B. Z. Tang, Macromolecules, 2010, 43, 4921 CrossRef CAS.
- J. Liu, J. W. Y. Lam and B. Z. Tang, J. Inorg. Organomet. Polym. Mater., 2009, 19, 249 CrossRef CAS.
-
(a) K. Tamao, S. Yamaguchi, M. Shiozaki, Y. Nakagawa and Y. Ito, J. Am. Chem. Soc., 1992, 114, 5867 CrossRef CAS;
(b) K. Tamao, S. Yamaguchi and M. Shiro, J. Am. Chem. Soc., 1994, 116, 11715 CrossRef CAS.
Footnote |
† Electronic supplementary information (ESI) available: 1H and 13C NMR spectra of 1 (Fig. S1 and S2), MS and particle size distribution of 1 (Fig. S3 and S4), UV and CD spectra of 1 in the absence and presence of chiral hydroxy acids (Fig. S5–S7). TEM and powder XRD of 1, pure R-(−)-mandelic acid, S-(+)-mandelic acid and the corresponding complexes (Fig. S7–S9).See DOI: 10.1039/c3tc31633e |
|
This journal is © The Royal Society of Chemistry 2014 |