DOI:
10.1039/C3SC52816B
(Edge Article)
Chem. Sci., 2014,
5, 771-781
Pre-inverse-crowns: synthetic, structural and reactivity studies of alkali metal magnesiates primed for inverse crown formation†
Received
10th October 2013
, Accepted 25th November 2013
First published on 26th November 2013
Abstract
Two new alkali metal monoalkyl-bisamido magnesiates, the potassium compound [KMg(TMP)2nBu] and its sodium congener [NaMg(TMP)2nBu] have been synthesised in crystalline form (TMP = 2,2,6,6-tetramethylpiperidide). Devoid of solvating ligands and possessing excellent solubility in hydrocarbon solvents, these compounds open up a new gateway for the synthesis of inverse crowns. X-ray crystallography established that [KMg(TMP)2nBu] exists in three polymorphic forms, namely a helical polymer with an infinite KNMgN chain, a hexamer with a 24-atom (KNMgN)6 ring having endo-disposed alkyl substituents, and a tetramer with a 16-atom (KNMgN)4 ring also having endo-disposed alkyl substituents. Proving their validity as pre-inverse-crowns, both magnesiates react with benzene and toluene to generate known inverse crowns in syntheses much improved from the original, supporting the idea that the metallations take place via a template effect. [KMg(TMP)2nBu] reacts with naphthalene to generate the new inverse crown [KMg(TMP)2(2–C10H7)]6, the molecular structure of which shows a 24-atom (KNMgN)6 host ring with six naphthalene guest anions regioselectively magnesiated at the 2-position. An alternative unprecedented 1,4-dimagnesiation of naphthalene was accomplished via [NaMg(TMP)2nBu] and its NaTMP co-complex “[NaMg(TMP)2nBu]·NaTMP”, manifested in [{Na4Mg2(TMP)4(2,2,6-trimethyl-1,2,3,4-tetrahydropyridide)2}(1,4-C10H6)]. Adding to its novelty, this 12-atom (NaNNaNMgN)2 inverse crown structure contains two demethylated TMP ligands as well as four intact ones. Reactivity studies show that the naphthalen-ide and -di-ide inverse crowns can be regioselectively iodinated to 2-iodo and 1,4-diiodonaphthalene respectively.
Introduction
Alkali metal magnesiates have recently seen increasing utilisation in key organic transformations such as metal–hydrogen exchange, metal–halogen exchange and nucleophilic addition reactions.1–20 Compared to the organolithium reagents commonly employed in these reactions, magnesiates (as well as other types of ate, most importantly zincates1,21–45) can show advantages of superior functional group tolerance and application at ambient temperature and in ethereal solvents. Highlighting the synergistic reactivity inherent to these bimetallics, conspicuously homometallic magnesium compounds [dialkyls, R2Mg; Grignard (RMgX) or Hauser (R2NMgX) reagents] are often inert in these same reactions, especially in aromatic functionalisations. Through an inspiring series of papers Knochel has shown that adding stoichiometric lithium chloride creates synergistic-operative “turbo-charged” organomagnesium reagents (RMgX·LiCl or R2NMgX·LiCl) that can exhibit excellent reactivity and regio-control across a range of deprotonation and addition applications.6,46–84 Though deprotonation reactions of magnesiates can be viewed superficially as simple C–H to C–Mg(R) exchanges, the structural manoeuvrings to arrive there can be extraordinarily complex. A good early exemplar of this complexity was our report that an in situ 1
:
1
:
3 mixture of n-butylsodium, n,s-dibutylmagnesium and 2,2,6,6-tetramethylpiperidine [TMP(H)] in n-hexane deprotonates benzene or toluene, but that the C–H to C-metal exchanges are manifested in 12-atom macrocyclic ring complexes [Na4Mg2(TMP)6(arenedi-ide)] (1a or 1b, Scheme 1). This structural novelty was accompanied by novel reactivity as in each example the arene has been regiospecifically doubly deprotonated on its ring [1,4- for benzene leading to (C6H4)2− in 1a or 2,5- for toluene leading to (C6H3CH3)2− in 1b] and remarkably the most thermodynamically acidic CH3 hydrogen atoms of toluene in the latter complex are left untouched.85 Perhaps counter-intuitively, only monodeprotonation of the arenes is achieved when switching to “more reactive” potassium, manifested in larger 24-atom polymetallic host rings [K6Mg6(TMP)12(arene-ide)6], 2a and 2b.86 These macrocyclic compounds have been coined inverse crowns, due to their inverse topological relationship to conventional crown ethers.87–90 However the downsides of these reactions are that the arene has to be used in vast excess, the yields of the products are only modest at best, and as the reactions could only be performed in situ the active intermediates to the bimetallic macrocycles could not be identified nor structurally characterised meaning that inverse crown preparations could only be optimised by trial and error rather than by tailoring a posteriori.
 |
| Scheme 1 Synthesis of inverse crown complexes 1a/b and 2a/b (for a R = H; for b R = Me). | |
Here, for the first time, we report crystalline “pre-inverse-crowns”, that is pure, well-defined unsolvated forms of the ate (alkali metal, magnesium, base ligand) mixtures, and provide evidence that inverse crown formation may occur via a template mechanism. As these multicomponent compounds possess excellent solubility in hydrocarbon solvents, a new gateway to inverse crowns is established. Using the fused ring aromatic hydrocarbon naphthalene as a case study we demonstrate that new inverse crowns can be rationally designed via these pre-inverse-crowns. The resulting new naphthalene-ide and -di-ide inverse crowns have subsequently been utilised in iodination reactions to produce iodo-substituted naphthalenes, which are potentially of use as building blocks in medicinal chemistry.91
Results and discussion
Synthesis and characterisation of potassium pre-inverse-crowns
Focusing initially on potassium systems, we decided to study the equimolar reaction between freshly prepared KTMP and nBuMgTMP in a variety of hydrocarbon solvents (Scheme 2).
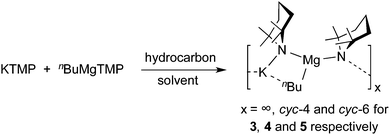 |
| Scheme 2 Synthesis of potassium pre-inverse crowns. | |
Pleasingly these reactions resulted in the isolation of several different oligomeric compounds. NMR spectroscopic studies of in situ mixtures of KTMP and nBuMgTMP in deuterated cyclohexane, cyc-C6D12, show a set of resonances that were not consistent with either starting material on their own (or potential monometallic metathesis products), indicating that co-complexation had occurred (see ESI)†. From a solid-state perspective, our first attempt to isolate a latent potassium-based pre-inverse-crown involved trying to grow crystals from a cyc-C6D12 solution of an equimolar mixture of KTMP and nBuMgTMP at room temperature. Crystallization occurred and the isolated product was a solvate of the polymer [KMg(TMP)2nBu]∞3 (Fig. 1). Structural determination by X-ray crystallography revealed an unusual helical potassium magnesiate. The backbone of the structure (Fig. 1b) can be considered to be the repeating KNMgN unit. The one dimensional helical polymers so formed lie parallel to the crystallographic a direction and have approximate (i.e. non-crystallographic) 31 screw symmetry. Each [KNMgN]∞ chain is supported by K⋯C⋯K cross-links (where C is from nBu) that thus form a series of four-atom, four-element (K–N–Mg–C) rings, fused together along the Mg–C edge to another ring of identical composition. Each potassium atom occupies a shared vertex that links neighbouring pairs of doubly-fused tetranuclear ring systems (Fig. 1). A total of twelve atoms within a K–N–Mg–C chain define both a single turn of the helix and the crystallographically unique segment of the polymer. The Mg centre forms short, strong bonds to the N atoms of both bridging TMP units [e.g., Mg2–N21, 2.102(4); Mg2–N31, 2.045(4) Å], and the C atom of the n-butyl anion [Mg2–C2A, 2.169(5) Å], thus placing Mg in a distorted trigonal planar environment [N21–Mg2–N31, 134.44(17); N21–Mg2–C2A, 111.97(17); and N31–Mg2–C2A, 113.45(18)°]. Each K atom’s coordination environment formally consists of a N2C2 ligand set. The K–N distances are approximately equal [K2–N31, 2.968(4); K2–N41, 2.943(4) Å], short in comparison to the more distended K⋯C contacts [K2–C2A 3.287(5) Å; K2–C3A 3.145(5) Å], which are primarily weak electrostatic interactions rather than formal covalent bonds. Interestingly, looking directly down the centre of the helix, the TMP ligands are arranged exo- whilst the n-butyl groups are arranged endo- with respect to the helical framework (Fig. 1c). Disordered solvent molecules occupy the gaps between polymeric chains – and thus the material is a channel solvate. This arrangement of a poly-amido outer scaffold with an inner alkyl unit follows the orientational pattern seen in the hexameric arene-encapsulated inverse crown species 2a/b.85
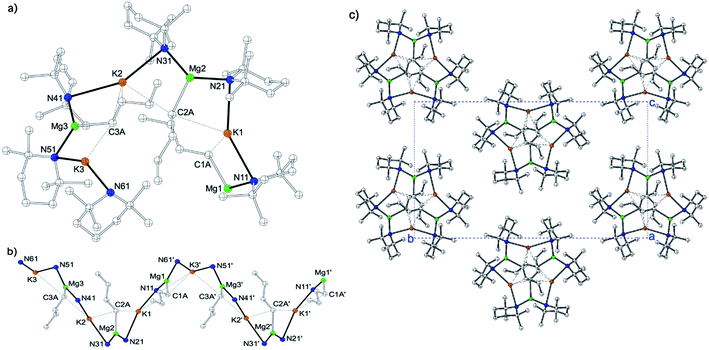 |
| Fig. 1 (a) Molecular structure of [KMg(TMP)2nBu]∞3, showing the contents of the asymmetric unit, which corresponds to a single turn of the helical chain. Hydrogen atoms are omitted for clarity. (b) Section of the extended framework structure showing atom connectivity between the metals, the n-butyl and connecting N atom of the TMP ligands. (c) Packing diagram of [KMg(TMP)2nBu]∞3 (viewed along a-axis). The dashed lines illustrate K⋯C contacts. Selected bond distances (Å) and angles (°): Mg1–C1A, 2.161(4); Mg1–N11, 2.033(4); Mg1–N61′, 2.069(4); K1–N11, 3.006(4); K1–N21, 2.939(4); K1–C1A, 3.321(5); K1–C2A, 3.142(5); Mg2–C2A, 2.169(5); Mg2–N21, 2.102(4), Mg2–N31, 2.045(4); 2.102(4); K2–N31, 2.968(4); K2–N41, 2.943(4); K2–C2A, 3.287(5); K2–C3A, 3.145(5); Mg3–N41, 2.098(4); Mg3–N51, 2.043(4); Mg3–C3A, 2.172(4); K3–N51, 2.985(4); K3–N61, 2.956(4); K3–C1A′′, 3.129(5); K3–C3A, 3.295(5); N11–Mg1–N61′, 132.76(16); N11–Mg1–C1A, 114.90(18); N61′–Mg1–C1A, 112.17(17); N21–K1–N11, 150.25(11); N21–K1–C2A, 71.13(11); N11–K1–C2A, 138.22(12); N21–K1–C1A, 127.55(11); N11–K1–C1A, 67.75(10); C2A–K1–C1A, 90.58(11); N31–Mg2–N21, 134.44(17); N31–Mg2–C2A, 113.45(18); N21–Mg2–C2A, 111.97(17); N41–K2–N31, 149.85(10); N41–K2–C3A, 71.13(10); N31–K2–C3A, 138.46(11); N41–K2–C2A, 127.06(11); N31–K2–C2A, 68.36(10); C3A-K2-C2A, 91.47(12); N51–Mg3–N41, 133.88(17); N51–Mg3–C3A, 13.74(17); N41–Mg3–C3A, 112.23(17); N61–K3–N51, 150.05(11); N61–K3–C1A′′, 70.41(10); N51–K3–C1A′′, 139.03(11); N61–K3–C3A, 127.50(11); N51–K3–C3A, 68.20(10); C1A′′–K3–C3A, 91.20(12). The symmetry operation used to generate the equivalent atoms labelled with ′ is x − 1, y, z; and ′′ is x + 1, y, z. | |
When the same reaction mixture is allowed to crystallise from methylcyclohexane at ambient temperature, the tetrameric oligomer [KMg(TMP)2nBu]44 is produced. High quality single crystals could not be formed – with all datasets examined showing multiple diffraction patterns. However, the X-ray crystallographic study does allow the atomic connectivity of 4 to be established (Fig. 2). The structure consists of a 16-atom polymetallic ring, of composition [K–N–Mg–N]4. Similarly to 3 above, the repeating KNMgN unit is supported by K⋯C⋯K cross links involving the n-butyl anion. Each K centre is thus four coordinate and the Mg atoms are three coordinate. As in the case of 3, the n-butyl groups all orientate towards the centre of the molecule. They have an anti arrangement as they alternately point above and below the plane of the [K–N–Mg–N]4 ring. Complex 4 exhibits similar structural features to the aforementioned inverse crowns 1 and 2 though its metal–nitrogen ring size (16-atom) differs (versus 12- and 24-atom, respectively85,86); but the salient feature is that instead of having a deprotonated aromatic solvent derived entity [e.g., (C6H4)2− or (C6H3CH3)2−] as its third ligand component, 4 has strongly Brønsted basic n-butyl anions seemingly primed for executing deprotonation reactions. It is this latter feature that drew our attention to 4 as a potential pre-inverse-crown.
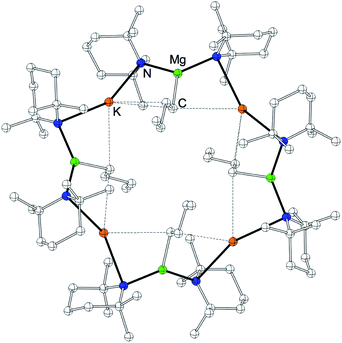 |
| Fig. 2 Atomic connectivity of [KMg(TMP)2nBu]44. The dashed lines illustrate K⋯C interactions. | |
By altering the solvent of crystallization from methylcyclohexane to cyclohexane solution and by cooling the solution to 8 °C, a third oligomeric form, a hexameric variant of the magnesiate [KMg(TMP)2nBu]65, was obtained (Fig. 3). Hexamer 5 features a 24-atom (KNMgN)6 centrosymmetric ring, with two and three coordinate K and Mg centres respectively, although the K is positioned in relatively close proximity to one of the α-carbon atoms of the neighbouring n-butyl groups [K1–C20, 3.143(5); K1–C20′, 3.243(5) Å]. The K atoms bridge between two TMP units, forming strong K–N contacts [K1–N1, 3.052(2); K1–N2, 3.053(2) Å], with a bridging N1–K1–N2 angle of 146.72(6)°. Mg occupies a distorted trigonal planar site [N1–Mg1–N2′′, 133.63(9); N1–Mg1–C20, 115.57(14); N2′′-Mg1–C20, 110.28(14)], and the inner n-butyl appendages point alternately above and below the ring plane, in a similar fashion to that observed in the tetrameric polymorph 4. Interestingly, continuing the pre-inverse-crown notion (i.e., 5 contains basic, reactive n-butyl ligands which are capable of inducing arene deprotonation), hexameric 5 appears to represent the perfect template to produce the arene-ide inverse crowns 2a and 2b, as it is directly comparable in terms of aggregation state and conformation with all its n-butyl appendages still intact to deprotonate benzene or toluene.86 When isolated crystals of both oligomeric forms (4 and 5) or polymeric 3 were dissolved in cyc-C6D12, their 1H NMR spectra were essentially identical to that obtained from a 1
:
1 mixture of KTMP and nBuMgTMP. This perhaps indicates that the energy difference between the different oligomers for this particular potassium magnesiate complex is small. DOSY (Diffusion-Ordered SpectroscopY) NMR studies92–112 of a 1
:
1 mixture of KTMP and nBuMgTMP in cyc-C6D12 was attempted but due to the highly reactive nature of the solution towards the employed standards, it was impossible to quantify the spectra.113 However, by performing DOSY with external calibration (see, ESI† for full details) we could study the oligomeric make up of the solution. Rather surprisingly, only a single oligomer could be observed in solution under these conditions (400 MHz, 27 °C in cyc-C6D12). The data suggest that this oligomer has an approximate molecular weight (Mw) of 2478.7 g mol−1, which is close to the predicted MW of hexameric 5 (Mw, 2406.1 g mol−1; −3.0% difference in MW). Therefore, our data suggest that no deaggregation equilibria exist for this species.
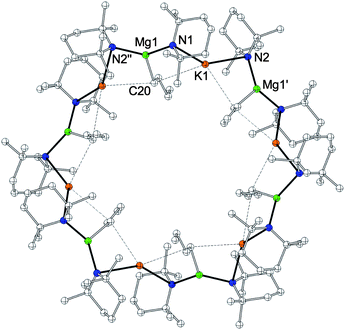 |
| Fig. 3 Molecular structure of [KMg(TMP)2nBu]65. Hydrogen atoms, solvent molecules of crystallization and one disordered component of the n-butyl ligand have been omitted for clarity. The dashed lines illustrate K⋯C contacts. Selected bond distances (Å) and angles (°): K1–N1, 3.052(2); K1–N2, 3.053(2); K1–C20, 3.143(5); K1–C20′, 3.243(5); Mg1–N1, 2.036(2); Mg1–N2′′, 2.043(2); Mg1–C20, 2.192(4); N1–K1–N2, 146.72(6); N1–K1–C20, 70.55(9); N2–K1–C20, 130.39(9); N1–K1–C20′, 140.65(8); N2–K1–C20′, 66.95(8); C20–K1–C20′, 103.28(18); N1–Mg1–N2′′, 133.63(9); N1–Mg1–C20, 115.57(14); N2′′–Mg1–C20, 110.28(14); Mg1–N1–K1, 89.43(7); Mg1′–N2–K1, 92.03(7); Mg1–C20–K1, 84.37(14); Mg1–C20–K1′′, 84.41(14); K1–C20–K1′′, 163.21(18). The symmetry operation used to generate the equivalent atoms labelled with ′ is y + 1, −x + y + 1,−z; and ′′ is x − y, x − 1, −z. | |
Next we decided to employ Density Functional Theory (DFT) calculations114 to estimate the relative gas-phase energy differences between the hypothetical monomeric, and dimeric oligomers of “KMg(TMP)2nBu” as well as the experimentally observed tetrameric and hexameric oligomers. By studying the association energies of each oligomer (Scheme 3) it was determined that the tetrameric and hexameric oligomers were equally as stable (ΔE, = −13.83 kcal mol−1 for each system, Fig. 4), thus mirroring the results of the solid-state data. As the oligomerisation state decreased from n = 3 to 1, the relative stabilities decreased (ΔE, = −10.42, −4.57 and 0 kcal mol−1 for trimer, dimer and monomer respectively).
 |
| Scheme 3 Modelled energy of oligomerisation reactions for KMg(TMP)2nBu. | |
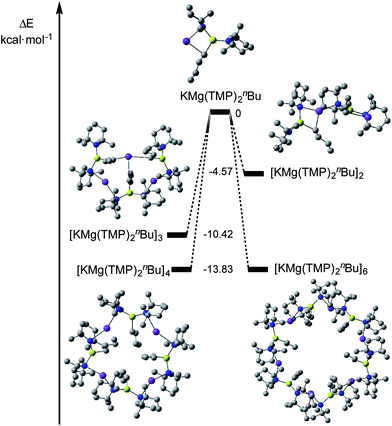 |
| Fig. 4 Computed relative electronic energies of association for KMg(TMP)2nBu. Hydrogen atoms omitted for clarity. | |
Application of potassium pre-inverse-crowns in the synthesis of inverse crowns
As already mentioned the original synthesis of the inverse crowns 1a/b and 2a/b relied on the employment of vast excesses of arene.85,86 In order to assess the reactivity of 3 as a potential pre-inverse-crown, we decided to investigate its reaction with equimolar quantities of benzene and toluene and to study both the solution and solid-state products. Could 3 (in the form of an in situ mixture of KTMP and nBuMgTMP) replicate or even outperform the originally used in situ (nBuK, n,sBu2Mg, 3 TMPH) mixtures? In answer, we found that at ambient temperature, the previously prepared inverse crown molecules could be isolated in good to excellent isolated yields (44% and 93% for 2a and 2b, respectively). The aforementioned DOSY NMR spectroscopy study appears to rule out equilibria allowing the deaggregation of the large cyclic oligomers to smaller dimers/trimers etc. (and hence creating different deprotonating species). There are also two other pieces of evidence which provide support that the larger oligomers are involved in the deprotonative chemistry: (i) the aforementioned gas phase experiments (Fig. 4) show that the tetramer and hexamer are considerably more stable than their smaller aggregates, and as the deprotonative reactions are performed in the absence of donor media, there is no driving force to induce deaggregation; and (ii) the solid-state structure of previously reported inverse crowns (having similar macrocyclic structures to 5) have been shown to be retained in hydrocarbon solution by a series of spectroscopic analyses.115,116 Therefore it appears that the in situ mixture of 3 in hydrocarbon solution is an excellent forerunner for the rational synthesis of these inverse crown macrocyclic complexes. This led on to another question, “could we utilise this in situ mixture to synthesise new inverse crown molecules with other “guest” anions encapsulated within the cationic ring?”
To answer this question, we turned our attention to the fused ring aromatic hydrocarbon system, naphthalene, as a case study. Naphthalene has been studied previously in metallation chemistry, both in terms of its reactivity with traditional alkyllithium reagents117,118 and more complex synergic bimetallic metallators.119–121 However to the best of our knowledge it has never been magnesiated directly. The naphthalene skeleton represents a popular unit in chemical and pharmaceutical industries with interesting optical, electronic and biological properties.122–131 In recent years, the development of new and efficient methodologies for the synthesis of substituted naphthalene derivatives has attracted attention in organic synthesis. Directed metallation of activated naphthalene derivatives with alkyllithium reagents akin to the Directed ortho Metallation (DoM)118,132–146 of activated benzene derivatives has been shown to provide access to functionalized naphthalene compounds.147,148 The more challenging metallation of non-substituted naphthalene has also been accomplished by the Lochmann–Schlosser superbase (nBuLi·KOtBu, LICKOR) which can di-deprotonate naphthalene at cryogenic temperatures, but not in a regioselective manner as twelve different mono/di-substituted isomers are formed in a collectively poor yield.121 Synergically-operative metallators such as sodium zincate (TMEDA)·Na(μ-TMP)(μ-tBu)Zn(tBu)149–155 or sodium manganate (TMEDA)·Na(μ-TMP)(μ-CH2SiMe3)Mn(TMP)119,156–159 offer an enhanced regioselectivity. Metallation (zincation120 or manganation119) at the 2-position of naphthalene occurs at room temperature via these respective reagents. Also a 2,6-di-zincated naphthalene derivative can be isolated upon heating a 2
:
1 mixture of the sodium zincate and naphthalene.120 However, although these last metallations have been carried out via alkali-metal-mediated metallations, none of them have produced an inverse crown product.
On reacting naphthalene with our new metallating agent 3 in methylcyclohexane/heptane solution we produced the first naphthalene-based inverse crown [KMg(TMP)2(2-C10H7)]66 following recrystallization from toluene. The yield of the isolated crystalline solid was 41%; but 1H NMR analysis of the crude reaction mixture showed that the reaction was essentially quantitative. The metallation of naphthalene was found to be highly regioselective at the 2-position, both in the isolated crystals and the crude reaction mixture. X-ray crystallographic analysis reveals that as with 5, 6 crystallises as a hexamer with
symmetry (Fig. 5). Its cationic ring is essentially isostructural to those of 2a/b,85,86 but its core contains six monodeprotonated naphthalenide ligands. This macrocycle features a 24-atom (KNMgN)6 ring, with significant interactions between K and the π-system of the naphthalenide ring resulting in a series of smaller doubly fused four-atom ring appendages, mirroring the situation witnessed in the polymer 3 and hexamer 5. The faces of each naphthalenide unit are orientated to maximize the number of contributing π interactions with the nearest K atom but the naphthalenide ring inclines slightly to favour one face. As a consequence, the equivalent K atoms engage in two distinct π-interactions, firstly binding to a naphthalenide anion in a classical η3 mode via the 1, 2, and 3-positions. The second interaction is to a second naphthalenide anion; however, this is considerably weaker and is tending towards η1 hapticity [for η3, K1′′′–C30, 3.064(4); K1′′′–C31, 3.318(4); K1′′–C35, 3.282(4); for η1, K1–C30, 3.128(4); K1⋯C31, 3.568(4); K1⋯C35, 3.525(5) Å]. These K–C (naphthalenide) interactions also describe two elegant internal structures, a 12-atom hexagonal arrangement propagating through the 2-position of the naphthalenide, and a complementary 12-atom, six-pointed star structure consisting exclusively of K π-interactions160–162 to C3-position of the naphthalenide group. The significant K-(naphthalenide) π-interactions make these smaller secondary rings essentially planar, revealing a doubly sided “paddle wheel” motif. When viewed side on, the (KNMgN)6 ring is extremely puckered, in an identical fashion found for hexamer 5 (Fig. 5b). The Mg centres are trigonal planar as in all previous inverse crown complexes, and are σ-bound to the 2-position of the naphthalenide ring system and also to two bridging TMP N atoms [N1–Mg1–N2, 136.08(12), N1–Mg1–C30, 110.30(14); N2–Mg1–C30, 113.46(14)°]. The short Mg–C [Mg1–C30, 2.248(2) Å], and Mg–N bonds [Mg1–N1, 2.028(3); Mg1–N2, 2.029(3) Å], reveal that the metal is strongly bound to the naphthalenide and TMP ligands. Within the host metal-amido ring system, each metal points inwards towards the centre of the molecule, exhibiting obtuse exocyclic bridging angles [N1–Mg1–N2, 136.08(12); N2–K1–N1′, 140.92(8)°], with concomitant projection of the N atoms outwards away from the centre, with these endocyclic bond angles being nearly acute (mean angle = 90.33°).
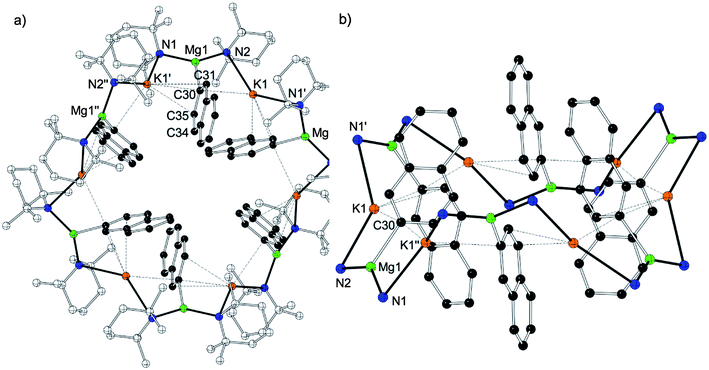 |
| Fig. 5 (a) Molecular structure of [KMg(TMP)2(2-C10H7)]66 (naphthalenide moiety in black), with hydrogen atoms, solvent molecule of crystallization and one disordered component of the naphthalenide ligand omitted for clarity; (b) core view (TMP C atoms and K–C interactions omitted for simplicity). The dashed lines illustrate K⋯C contacts. Key bond distances (Å) and angles (°): K1–C30, 3.128(4); K1–C31, 3.568(4); K1–C35, 3.525(5); K1–N2, 3.072(3); K1–C30′, 3.064(4); K1–N1′, 3.174(3); K1–C31′, 3.318(12); Mg1–N1, 2.028(3); Mg1–N2, 2.029(3); Mg1–C30, 2.248(2); N1–K1′′, 3.174(3); C30–K1′′, 3.064(4); C31–K1′′, 3.318(4); C35–K1′′, 3.282(4); C30′–K1–N2, 141.24(7); C30′-K1-C30, 117.75(11); N2–K1–C30, 70.50(7); C30′–K1–N1′, 68.49(7); N2–K1–N1′, 140.92(8); C30–K1–N1′, 125.30(7); N2–K1–C35′, 138.89(18); N1′-K1-C35′, 79.53(18); N2–K1–C31′, 117.52(17); N1′–K1–C31′, 84.4(2); N2–K1–C35, 84.54(7); N1′–K1–C35, 124.33(7); N1–Mg1–N2, 136.08(12); N1–Mg1–C30, 110.30(14); N2–Mg1–C30, 113.46(14); N1–Mg1–K1, 163.39(9); N2–Mg1–K1, 56.44(8); C30–Mg1–K1, 57.68(11); N1–Mg1–K1′′, 57.09(8); N2–Mg1–K1′′, 166.22(9); C30–Mg1–K1′′, 54.13(11); K1–Mg1–K1′′, 111.79(3); Mg1–N1–K1′′, 90.48(10); Mg1–N2–K1, 90.17(9); Mg1–C30–K1′′, 89.38(12); Mg1–C30–K1, 84.91(10). The symmetry operation used to generate the equivalent atoms labelled with ′ is y, −x + y, −z + 1; and ′′ is x − y, x, −z + 1. | |
The poly-naphthalene-ide inverse crown 6 exhibits low solubility in common non-polar deuterated hydrocarbon solvents; so NMR spectroscopic characterisation in the more polar (coordinating) d8-THF has been used at the potential cost of changing the nature of the structure by deaggregating the hexamer. Consistent with the 2-magnesiation seen in the solid-state structure, the 1H NMR spectrum for 6 shows seven spectroscopically unique and inequivalent aromatic resonances. The downfield singlet and doublet at 8.37 ppm and 8.20 ppm, respectively, are attributed to C31–H and C35–H, adjacent to the Mg–C bond. The doublet at 7.31 ppm is attributed to C34–H and the non-deprotonated second ring of the naphthalenide ligand gives rise to four distinct resonances between 7.01 and 7.57 ppm. The 13C NMR spectrum mirrors that observed above, with seven distinct resonances in the aromatic region representative of the seven unique C–H environments.
Previous examples of potassium-mediated magnesiations163 with dinuclear Lewis base stabilized potassium alkyl-amido-magnesiates reveal that they react kinetically with arenes via their TMP anion [to afford a new organometallic as well as TMP(H)]. Ultimately, in turn these two compounds react together to produce the thermodynamic organometallic amide product and alkane co-product. This two-step mechanism has only been demonstrated with relatively simple dinuclear Lewis base stabilized motifs such as (PMDETA).K(TMP)(CH2SiMe3)Mg(TMP) (PMDETA is N,N,N′,N′′,N′′-pentamethyldiethylenetriamine) which critically do not generate inverse crowns but retain their dinuclearity post metallation of the arene. In contrast the systems herein are Lewis base free having high polynuclear structures that appear set up to be able to deprotonate arenes thermodynamically in a single step through alkyl basicity.
Extension to sodium
Next it was decided to investigate a sodium base system with naphthalene. Following the precedent set by the potassium pre-inverse-crown system, by treating equimolar quantities of freshly prepared NaTMP, and nBuMgTMP in hydrocarbon solvents it was envisaged that these reagents could co-complex resulting in a potential precursor to 1a/b.85 This strategy was successfully employed in methylcyclohexane solution to generate “NaMg(TMP)2nBu” 7 (Scheme 4). Sodium magnesiate 7 could be crystallised and isolated in a 61% yield from a methylcyclohexane solution at 8 °C. Unfortunately, attempts to obtain high quality crystallographic data for crystalline 7 were unsuccessful due to the high disorder found in both TMP and n-butyl ligands present within the structure. However, 1H NMR spectroscopic data (obtained from a cyc-C6D12 solution) are consistent with the 2
:
1 TMP:nBu composition expected for 7. When an equimolar mixture of NaTMP and nBuMgTMP is dissolved in cyc-C6D12, its 1H NMR spectrum is identical to that obtained from a solution of isolated crystals of 7 in the same solvent.
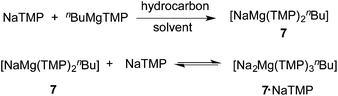 |
| Scheme 4 Synthesis of sodium pre-inverse crowns. | |
It is immediately apparent that the composition of the sodium magnesiate 7 (Na:Mg ratio, 1
:
1) is different from the polyamide ring required to form inverse crowns 1a/b (Na:Mg ratio, 2
:
1).85 Therefore it was decided to assess whether a hydrocarbon solution of 7 could be a precursor to 1a/b or whether a different species was active in the preparation of the known inverse crown complexes.85 Thus it was discovered that on treating 7 with an equimolar quantity of benzene or toluene, the respective inverse crowns 1a/b could be isolated from the reaction solutions albeit in moderate yields (63% and 48%, for C6H6 and C6H5CH3, respectively). Significantly, however, when 7 is pre-treated with an additional equivalent of NaTMP (to match the observed 2
:
1 Na:Mg stoichiometry within the metal-amido rings in 1a/b85) the isolated yields of the inverse crowns were improved (to 73% and 88%, for C6H6 and C6H5CH3, respectively). It is well known that NaTMP is insoluble in hydrocarbon solvents at ambient temperature;164 however, on adding NaTMP to a hydrocarbon solution of 7 at ambient temperature, homogeneity was achieved, most likely indicating that the amide has co-complexed with the pre-existing sodium magnesiate species 7. Thus far we have been unable to isolate crystalline/solid material from 7·NaTMP, but 1H NMR spectroscopic studies of the reaction mixture in cyc-C6D12 reveal that the equilibrium shown in Scheme 4 could be involved as a new species appears to form (broad singlet at −0.43 ppm attributed to a α-CH2 from a new n-butyl from Na2MgTMP3nBu) and a reduced quantity of free NaTMP is observed (see, ESI†).
In order to probe the deprotonative capability of the bimetallic complexes, 7 and 7·NaTMP, each was reacted with an equimolar amount of naphthalene in methylcyclohexane solution. To the best of our knowledge (vide supra), using conventional alkyllithium or existing organo-bimetallic bases, naphthalene has only been regioselectively monometalated at the 2-position or di-metallated at 2,6-positions.119,120 On utilising 7, we have established a new unprecedented metallation pattern for naphthalene, namely selective 1,4-di-metallation. Along with this novel regioselectivity, the reaction produces a novel new inverse crown in [{Na4Mg2(TMP)4(TTHP)2(1,4-C10H6)] 8 (Fig. 6) (where TTHP is 2,2,6-trimethyl-1,2,3,4-tetrahydropyridide). The 12-atom Na4Mg2N6 ring is slightly bent and the naphthalenedi-ide group lies in an orthogonal manner to the mean plane of the poly-amido-metallic ring. The di-cationic ring forms a six-pointed star with those corresponding outer N atoms and inner Mg and Na atoms. In 8, the C–H missing bonds from the naphthalene are replaced by strong C–Mg σ-bonds (mean distance, 2.220 Å) and are comparable to those in 1a/b (2.196 Å). The Mg atoms adopt distorted trigonal-planar geometries and the N–Na–N units are coplanar with the magnesiated carbons to maximize the C–Na π-interactions. The sodium atoms in 8 lie above and below the metallated aromatic ring faces, and engage π-interactions with the C atoms in 1, 2- and 3, 4-positions (Na–C, 2.674–3.265 Å). The Na–N TMP bonds within the inverse crown structure (mean distance, 2.534 Å) are 0.493 Å longer than those strong N–Mg TMP (mean 2.041 Å) bonds.
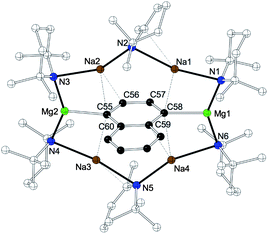 |
| Fig. 6 Molecular structure of [{Na4Mg2(TMP)4(2,2,6-trimethyl-1,2,3,4-tetrahydropyridide)2}(1,4-C10H6)] 8. Hydrogen atoms and one disordered component of the TTHP ligand omitted for clarity. The dashed lines illustrate Na⋯C interactions. Key bond distances (Å) and angles (°): Mg1–N6, 2.043(5); Mg1–N1, 2.048(5); Mg1–C58, 2.220(5); Mg1–Na1, 3.168(3); Mg1–Na4, 3.199(3); Mg2–N3, 2.032(5); Mg2–N4, 2.040(5); Mg2–C55, 2.219(5); Mg2–Na3, 3.164(3); Mg2–Na2, 3.212(3); Na1–N1, 2.531(5); Na1–N2, 2.585(12); Na1–C58, 2.683(6); Na1–Na2, 3.860(3); Na2–N2, 2.185(15); Na2–N3, 2.539(5); Na2–C55, 2.675(6); Na2–C56, 3.012(5); Na3–N5, 2.479(5); Na3–N4, 2.534(5); Na3–C55, 2.697(6); Na3–Na4, 3.899(3); Na4–N5, 2.288(5); Na4–N6, 2.529(5); Na4–C58, 2.693(6); Na4–C59, 3.017(6); N6–C50, 1.481(8); N6–Mg1–N1, 142.67(19); N6–Mg1–C58, 107.9(2); N1–Mg1–C58, 109.4(2); N6–Mg1–Na1, 164.37(14); N1–Mg1–Na1, 52.92(13); C58–Mg1–Na1, 56.48(16); N6–Mg1–Na4, 52.20(13); N1–Mg1–Na4, 164.85(15); C58–Mg1–Na4, 56.17(16); Na1–Mg1–Na4, 112.35(7); N3–Mg2–N4, 142.5(2); N3–Mg2–C55, 107.7(2); N4–Mg2–C55, 109.7(2); N3–Mg2–Na3, 164.44(15); N4–Mg2–Na3, 53.10(14); C55–Mg2–Na3, 56.94(15); N3–Mg2–Na2, 52.21(14); N4–Mg2–Na2, 164.98(14); C55–Mg2–Na2, 55.44(15); Na3–Mg2–Na2, 112.32(7); N1–Na1–N2, 166.7(4); N1–Na1–C58, 83.79(17); N2–Na1–C58, 108.9(4); C58–Na1–Na2, 76.44(12); N2–Na2–C55, 123.5(4); N3–Na2–C55, 82.33(16); N5–Na3–N4, 166.38(18); N5–Na3–C55, 109.45(16); N4–Na3–C55, 83.49(16); N4–Na3–Na4, 157.49(14); N5–Na4–N6, 156.68(19); N5–Na4–C58, 119.89(17); N6–Na4–C58, 82.57(16); Mg1–N1–Na1, 86.89(17); Mg2–N3–Na2, 88.55(17); Mg2–N4–Na3, 86.82(17); Na4–N5–Na3, 109.71(19); Mg1–N6–Na4, 88.14(16); Na2–N2–Na1, 107.8(6); Mg2–C55–Na2, 81.46(17); Mg2–C55–Na3, 79.46(17); Na2–C55–Na3, 160.7(2); Mg1–C58–Na1, 79.90(18); Mg1–C58–Na4, 80.62(17); Na1–C58–Na4, 159.4(2). | |
This compound was isolated in a 51% yield. Matching previous results, 8 was isolated in higher yield (76%) by reacting equimolar quantities of 7·NaTMP and naphthalene. At first glance, the X-ray determined structure of 8 displays a 12-atom Na4Mg2N6 cationic metal-amido ring which appears identical to that in 1a/b;85 however, two of the six amide ligands appear to have formally lost methane to produce TTHP anions. It should be stressed that this reaction leading to 8 is completely reproducible and that an inverse crown with six all intact TMP ligands has not been isolated when naphthalene has been utilized as the arene substrate. This perhaps suggests that the arene's increased steric bulk (with respect to benzene or toluene in 1a and b, respectively) has forced a sterically-driven demethylation of the amide. To the best of our knowledge this is the first time that a complex which contains a demethylated TMP ligand has been characterized; however, Shiner and co-workers have previously shown some indirect evidence for a demethylation process involving LiTMP.165 Returning to 8, its 1H NMR spectrum obtained from a cyc-C6D12 solution appears to show that the complex remains intact in solution. Most indicative of this fact is that a sharp resonance for the two Me groups present on the α-C(sp3) of the 2,2,6-trimethyl-1,2,3,4-tetrahydropyridide ligand are situated over the π-face of the arene rendering them highly shielded causing an upfield shift to 0.06 ppm (see ESI). As expected, the spectrum also shows a singlet and two multiplets (7.92, 7.95 and 7.29 ppm, respectively) in the aromatic region in a 1
:
1
:
1 ratio assignable to a 1,4-dideprotonated naphthalene consistent with the 1,4-dimagnesiation of the arene observed in the crystal structure. The 13C NMR spectrum replicate the situation indicated by the appearance of the 1H NMR spectrum. An intriguing question to ask is “why does the sodium magnesiate induce a two-fold deprotonation whilst the assumed more reactive potassium magnesiate solution only mono-metallates naphthalene?” One plausible explanation is that the larger rings formed in the potassium case allow the heavier, softer alkali metal to maximise its stabilisation via π-arene interactions, especially as the reaction medium is free of donor atoms. Fig. 5a implies that each K centre receives a double helping of π-interactions, a situation that would not be possible if Na was simply replaced by K in 8 (Fig. 6). Moreover in comparing 6 with 8 and speculating on the nature of their pre-inverse-crowns (presumably 5 for K) it must be stressed that we are not comparing like with like as the K case has a 1
:
1, K:Mg stoichiometry; whereas in the Na case, the corresponding ratio is 2
:
1.
Reactivity studies of the new inverse crowns
Turning to the utility of the potassium system 6 in synthesis (Scheme 5), when an in situ methylcyclohexane suspension of 6 is reacted with iodine in THF solution, 2-iodonaphthalene119,120,166–1699 was isolated in 67% yield. When the reaction was repeated using isolated 6 suspended in methylcyclohexane, 9 was obtained in a significantly higher yield (84%).
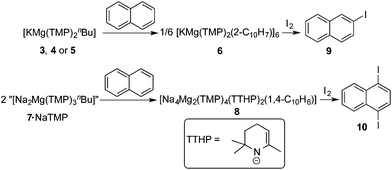 |
| Scheme 5 Optimised syntheses of iodonaphthalenes via novel inverse crown intermediates. | |
To assess the potential synthetic utility of the sodium system 7, an in situ solution mixture of 7·NaTMP in methylcyclohexane and naphthalene were reacted and subsequently treated with iodine in THF. After work-up, the formation of 1,4-diiodonaphthalene 10 in an 86% yield was accomplished.170 When isolated 8 suspended in methylcyclohexane was reacted with iodine in THF solution, a marginally improved conversion to 10 was observed (to 89% yield).
Conclusions
In summary, by synthesising and managing to isolate crystalline alkali metal magnesiates containing a combination of two TMP ligands and one n-butyl ligand but no donor solvent ligands, we have gained access to valuable precursors to inverse crown macrocyclic complexes. The idea that these unsolvated bimetallic compounds could function as pre-inverse-crowns came from the remarkable structural chemistry of [KMg(TMP)2nBu], with three oligomeric states revealed (tetrameric, hexameric and polymeric), with architectural features closely akin to existing inverse crowns but with the deprotonated entity substituted by an active alkyl base ligand. Confirmation of their pre-inverse-crown status was established through reactions with naphthalene that in executing regioselective mono- and di-deprotonation of the arene produced novel new types of inverse crown. With these new pre-inverse-crowns in hand, and others likely to follow, the floodgates for broadening the scope of inverse crown chemistry have been opened wide.†
Acknowledgements
This research was supported by the UK Engineering and Physical Science Research Council (EP/J001872/1 and EP/L001497/1); (Career Acceleration Fellowship to CTOH), the Royal Society (Wolfson research merit award to REM) and the Royal Society of Edinburgh/BP Trust, (Fellowship to SDR). We also thank Prof. E. Hevia for many helpful insightful comments.
References
- F. Mongin and A. Harrison-Marchand, Chem. Rev., 2013, 113, 7470 CrossRef PubMed.
- C. Lichtenberg, T. P. Spaniol, I. Peckermann, T. P. Hanusa and J. Okuda, J. Am. Chem. Soc., 2013, 135, 811 CrossRef CAS PubMed.
- M. I. Antczak and J. M. Ready, Chem. Sci., 2012, 3, 1450 RSC.
- B. J. Fleming, P. García-Álvarez, E. Keating, A. R. Kennedy and C. T. O'Hara, Inorg. Chim. Acta, 2012, 384, 154 CrossRef CAS.
- S. E. Baillie, W. Clegg, P. Garcia-Alvarez, E. Hevia, A. R. Kennedy, J. Klett and L. Russo, Organometallics, 2012, 31, 5131 CrossRef CAS.
- B. Haag, M. Mosrin, H. Ila, V. Malakhov and P. Knochel, Angew. Chem., Int. Ed., 2011, 50, 9794 CrossRef CAS PubMed.
-
C. T. O'Hara, in Specialist Periodical Reports: Organometallic Chemistry, ed. I. J. S. Fairlamb and J. M. Lynam, The Royal Society of Chemistry, Cambridge, UK, 2011, vol. 37, p. 1 Search PubMed.
- P. García-Álvarez, A. R. Kennedy, C. T. O'Hara, K. Reilly and G. M. Robertson, Dalton Trans., 2011, 40, 5332 RSC.
- R. Campbell, B. Conway, G. S. Fairweather, P. García-Álvarez, A. R. Kennedy, J. Klett, R. E. Mulvey, C. T. O'Hara and G. M. Robertson, Dalton Trans., 2010, 511 RSC.
- A. R. Kennedy and C. T. O'Hara, Dalton Trans., 2008, 4975 RSC.
- R. E. Mulvey, F. Mongin, M. Uchiyama and Y. Kondo, Angew. Chem., Int. Ed., 2007, 46, 3802 CrossRef CAS PubMed.
- A. D. Pajerski, D. M. Kushlan, M. Parvez and H. G. Richey, Organometallics, 2006, 25, 1206 CrossRef CAS.
- A. D. Pajerski, E. P. Squiller, M. Parvez, R. R. Whittle and H. G. Richey, Organometallics, 2005, 24, 809 CrossRef CAS.
- A. Deffieux, L. Shcheglova, A. Barabanova, J. M. Marechal and S. Carlotti, Macromol. Symp., 2004, 215, 17 CrossRef CAS.
- K. W. Henderson, G. W. Honeyman, A. R. Kennedy, R. E. Mulvey, J. A. Parkinson and D. C. Sherrington, Dalton Trans., 2003, 1365 RSC.
- J. E. Chubb and H. G. Richey, Organometallics, 2003, 22, 5141 CrossRef CAS.
- H. Tang, M. Parvez and H. G. Richey, Organometallics, 2000, 19, 4810 CrossRef CAS.
- H. Viebrock, U. Behrens and E. Weiss, Angew. Chem., Int. Ed., Engl., 1994, 33, 1257 CrossRef.
- W. Clegg, K. W. Henderson, R. E. Mulvey and P. A. O'Neil, J. Chem. Soc., Chem. Commun., 1993, 969 RSC.
- K. M. Waggoner and P. P. Power, Organometallics, 1992, 11, 3209 CrossRef CAS.
- C. I. Stathakis, S. M. Manolikakes and P. Knochel, Org. Lett., 2013, 15, 1302 CrossRef CAS PubMed.
- D. R. Armstrong, J. A. Garden, A. R. Kennedy, R. E. Mulvey and S. D. Robertson, Angew. Chem., Int. Ed., 2013, 52, 7190 CrossRef CAS PubMed.
- D. Tilly, K. Snegaroff, G. Dayaker, F. Chevallier, P. C. Gros and F. Mongin, Tetrahedron, 2012, 68, 8761 CrossRef CAS.
- L. C. McCann, H. N. Hunter, J. A. C. Clyburne and M. G. Organ, Angew. Chem., Int. Ed., 2012, 51, 7024 CrossRef CAS PubMed.
- M. Isobe, C.-T. Chiang, K.-W. Tsao, C.-Y. Cheng and R. Bruening, Eur. J. Org. Chem., 2012, 2109 CrossRef CAS.
- E. Hevia, A. R. Kennedy and M. D. McCall, Dalton Trans., 2012, 41, 98 RSC.
- J. A. Garden, A. R. Kennedy, R. E. Mulvey and S. D. Robertson, Chem. Commun., 2012, 48, 5265 RSC.
- M. Uchiyama, Y. Matsumoto, D. Nobuto, T. Furuyama, K. Yamaguchi and K. Morokuma, J. Am. Chem. Soc., 2006, 128, 8748 CrossRef CAS PubMed.
- E. Erdik and E. Z. Serdar, J. Organomet. Chem., 2012, 703, 1 CrossRef CAS.
- G. Dayaker, D. Tilly, F. Chevallier, G. Hilmersson, P. C. Gros and F. Mongin, Eur. J. Inorg. Chem., 2012, 6051 CrossRef CAS.
- S. E. Baillie, V. L. Blair, D. C. Blakemore, D. Hay, A. R. Kennedy, D. C. Pryde and E. Hevia, Chem. Commun., 2012, 48, 1985 RSC.
- D. R. Armstrong, L. Balloch, J. J. Crawford, B. J. Fleming, L. M. Hogg, A. R. Kennedy, J. Klett, R. E. Mulvey, C. T. O'Hara, S. A. Orr and S. D. Robertson, Chem. Commun., 2012, 48, 1541 RSC.
- H. N. Hunter, N. Hadei, V. Blagojevic, P. Patschinski, G. T. Achonduh, S. Avola, D. K. Bohme and M. G. Organ, Chem.–Eur. J., 2011, 17, 7845 CrossRef CAS PubMed.
- J. A. Garden, A. R. Kennedy, R. E. Mulvey and S. D. Robertson, Dalton Trans., 2011, 40, 11945 RSC.
- P. García-Álvarez, R. E. Mulvey and J. A. Parkinson, Angew. Chem., Int. Ed., 2011, 50, 9668 CrossRef PubMed.
- A. Deagostino, C. Prandi, S. Tabasso and P. Venturello, Curr. Org. Chem., 2011, 15, 2390 CrossRef CAS.
- J. J. Crawford, B. J. Fleming, A. R. Kennedy, J. Klett, C. T. O'Hara and S. A. Orr, Chem. Commun., 2011, 47, 3772 RSC.
- V. L. Blair, D. C. Blakemore, D. Hay, E. Hevia and D. C. Pryde, Tetrahedron Lett., 2011, 52, 4590 CrossRef CAS.
- L. Balloch, A. R. Kennedy, R. E. Mulvey, T. Rantanen, S. D. Robertson and V. Snieckus, Organometallics, 2011, 30, 145 CrossRef CAS.
- D. R. Armstrong, L. Balloch, E. Hevia, A. R. Kennedy, R. E. Mulvey, C. T. O'Hara and S. D. Robertson, Beilstein J. Org. Chem., 2011, 7, 1234 CrossRef CAS PubMed.
- C. Y. Liu, X. Wang, T. Furuyama, S. Yasuike, A. Muranaka, K. Morokuma and M. Uchiyama, Chem.–Eur. J., 2010, 16, 1780 CrossRef CAS PubMed.
- L. Balloch, A. R. Kennedy, J. Klett, R. E. Mulvey and C. T. O'Hara, Chem. Commun., 2010, 46, 2319 RSC.
- D. R. Armstrong, V. L. Blair, W. Clegg, S. H. Dale, J. García-Álvarez, G. W. Honeyman, E. Hevia, R. E. Mulvey and L. Russo, J. Am. Chem. Soc., 2010, 132, 9480 CrossRef CAS PubMed.
- S. Nakamura, C. Y. Liu, A. Muranaka and M. Uchiyama, Chem.–Eur. J., 2009, 15, 5686 CrossRef CAS PubMed.
- D. R. Armstrong, W. Clegg, S. H. Dale, J. García-Álvarez, R. W. Harrington, E. Hevia, G. W. Honeyman, A. R. Kennedy, R. E. Mulvey and C. T. O'Hara, Chem. Commun., 2008, 187 RSC.
- S. Yamada, A. Gavryushin and P. Knochel, Angew. Chem., Int. Ed., 2010, 49, 2215 CrossRef CAS PubMed.
- S. H. Wunderlich, C. J. Rohbogner, A. Unsinn and P. Knochel, Org. Process Res. Dev., 2010, 14, 339 CrossRef CAS.
- C. J. Rohbogner, S. Wirth and P. Knochel, Org. Lett., 2010, 12, 1984 CrossRef CAS PubMed.
- G. Monzon and P. Knochel, Synlett, 2010, 304 CAS.
- L. Shi, Y. Y. Chu, P. Knochel and H. Mayr, J. Org. Chem., 2009, 74, 2760 CrossRef CAS PubMed.
- C. J. Rohbogner, S. H. Wunderlich, G. C. Clososki and P. Knochel, Eur. J. Org. Chem., 2009, 1781 CrossRef CAS.
- C. B. Rauhat, C. Cervino, A. Krasovskiy and P. Knochel, Synlett, 2009, 67 Search PubMed.
- F. M. Piller, A. Metzger, M. A. Schade, B. A. Haag, A. Gavryushin and P. Knochel, Chem.–Eur. J., 2009, 15, 7192 CrossRef CAS PubMed.
- F. M. Piller and P. Knochel, Org. Lett., 2009, 11, 445 CrossRef CAS PubMed.
- M. Mosrin and P. Knochel, Chem.–Eur. J., 2009, 15, 1468 CrossRef CAS PubMed.
- L. Melzig, C. B. Rauhat and P. Knochel, Synthesis, 2009, 1041 CAS.
- L. Melzig, C. B. Rauhat and P. Knochel, Chem. Commun., 2009, 3536 RSC.
- G. Manolikakes and P. Knochel, Angew. Chem., Int. Ed., 2009, 48, 205 CrossRef CAS PubMed.
- C. Despotopoulou, L. Klier and P. Knochel, Org. Lett., 2009, 11, 3326 CrossRef CAS PubMed.
- A. H. Stoll and P. Knochel, Org. Lett., 2008, 10, 113 CrossRef CAS PubMed.
- L. Shi, Y. Chu, P. Knochel and H. Mayr, Angew. Chem., Int. Ed., 2008, 47, 202 CrossRef CAS PubMed.
- C. J. Rohbogner, G. C. Clososki and P. Knochel, Angew. Chem., Int. Ed., 2008, 47, 1503 CrossRef CAS PubMed.
- C. B. Rauhat, V. A. Vu, F. F. Fleming and P. Knochel, Org. Lett., 2008, 10, 1187 CrossRef PubMed.
- F. M. Piller, P. Appukkuttan, A. Gavryushin, M. Helm and P. Knochel, Angew. Chem., Int. Ed., 2008, 47, 6802 CrossRef CAS PubMed.
- M. Mosrin, M. Petrera and P. Knochel, Synthesis, 2008, 3697 CAS.
- M. Mosrin and P. Knochel, Org. Lett., 2008, 10, 2497 CrossRef CAS PubMed.
- C. Despotopoulou, R. C. Bauer, A. Krasovskiy, P. Mayer, J. M. Stryker and P. Knochel, Chem.–Eur. J., 2008, 14, 2499 CrossRef CAS PubMed.
- N. Boudet, S. R. Dubbaka and P. Knochel, Org. Lett., 2008, 10, 1715 CrossRef CAS PubMed.
- S. H. Wunderlich and P. Knochel, Angew. Chem., Int. Ed., 2007, 46, 7685 CrossRef CAS PubMed.
- A. H. Stoll, P. Mayer and P. Knochel, Organometallics, 2007, 26, 6694 CrossRef CAS.
- F. Kopp, S. Wunderlich and P. Knochel, Chem. Commun., 2007, 2075 RSC.
- F. Kopp and P. Knochel, Synlett, 2007, 980 CAS.
- M. Kienle, S. R. Dubbaka, V. del Amo and P. Knochel, Synthesis, 2007, 1272 CAS.
- G. C. Clososki, C. J. Rohbogner and P. Knochel, Angew. Chem., Int. Ed., 2007, 46, 7681 CrossRef CAS PubMed.
- N. Boudet, J. R. Lachs and P. Knochel, Org. Lett., 2007, 9, 5525 CrossRef CAS PubMed.
- W. Lin, O. Baron and P. Knochel, Org. Lett., 2006, 8, 5673 CrossRef CAS PubMed.
- A. Krasovskiy, B. F. Straub and P. Knochel, Angew. Chem., Int. Ed., 2006, 45, 159 CrossRef CAS PubMed.
- A. Krasovskiy, V. Krasovskaya and P. Knochel, Angew. Chem., Int. Ed., 2006, 45, 2958 CrossRef CAS PubMed.
- H. Ila, O. Baron, A. J. Wagner and P. Knochel, Chem. Commun., 2006, 583 RSC.
- H. Ila, O. Baron, A. J. Wagner and P. Knochel, Chem. Lett., 2006, 35, 2 CrossRef CAS.
- V. del Amo, S. R. Dubbaka, A. Krasovskiy and P. Knochel, Angew. Chem., Int. Ed., 2006, 45, 7838 CrossRef CAS PubMed.
- C.-Y. Liu and P. Knochel, Org. Lett., 2005, 7, 2543 CrossRef CAS PubMed.
- A. Krasovskiy and P. Knochel, Angew. Chem., Int. Ed., 2004, 43, 3333 CrossRef CAS PubMed.
- F. Kopp, A. Krasovskiy and P. Knochel, Chem. Commun., 2004, 2288 RSC.
- D. R. Armstrong, A. R. Kennedy, R. E. Mulvey and R. B. Rowlings, Angew. Chem., Int. Ed., 1999, 38, 131 CrossRef CAS.
- P. C. Andrews, A. R. Kennedy, R. E. Mulvey, C. L. Raston, B. A. Roberts and R. B. Rowlings, Angew. Chem., Int. Ed., 2000, 39, 1960 CrossRef CAS.
- A. R. Kennedy, J. Klett, R. E. Mulvey, S. Newton and D. S. Wright, Chem. Commun., 2008, 308 RSC.
- R. E. Mulvey, Organometallics, 2006, 25, 1060 CrossRef CAS.
- R. E. Mulvey, Chem. Commun., 2001, 1049 RSC.
- A. R. Kennedy, R. E. Mulvey and R. B. Rowlings, J. Am. Chem. Soc., 1998, 120, 7816 CrossRef CAS.
- R. S. Upadhayaya, J. K. Vandavasi, R. A. Kardile, S. V. Lahore, S. S. Dixit, H. S. Deokar, P. D. Shinde, M. P. Sarmah and J. Chattopadyaya, Eur. J. Med. Chem., 2010, 45, 1854 CrossRef CAS PubMed.
- C. C. Su, R. Hopson and P. G. Williard, Eur. J. Inorg. Chem., 2013, 4136 CrossRef CAS.
- D. R. Armstrong, A. R. Kennedy, R. E. Mulvey, J. A. Parkinson and S. D. Robertson, Chem. Sci., 2012, 3, 2700 RSC.
- W. B. Li, G. Kagan, R. Hopson and P. G. Williard, Arkivoc, 2011, 180 CAS.
- W. B. Li, G. Kagan, R. Hopson and P. G. Williard, J. Chem. Educ., 2011, 88, 1331 CrossRef CAS.
- T. Tatic, S. Hermann, M. John, A. Loquet, A. Lange and D. Stalke, Angew. Chem., Int. Ed., 2011, 50, 6666 CrossRef CAS PubMed.
- E. Hevia, A. R. Kennedy, R. E. Mulvey, D. L. Ramsey and S. D. Robertson, Chem.–Eur. J., 2013, 19, 14069 CrossRef CAS PubMed.
- D. R. Armstrong, P. García-Álvarez, A. R. Kennedy, R. E. Mulvey and S. D. Robertson, Chem.–Eur. J., 2011, 17, 6725 CrossRef CAS PubMed.
- A. M. Socha, G. Kagan, W. B. Li, R. Hopson, J. K. Sello and P. G. Williard, Energy Fuels, 2010, 24, 4518 CrossRef CAS.
- W. B. Li, G. Kagan, H. A. Yang, C. Cai, R. Hopson, D. A. Sweigart and P. G. Williard, Org. Lett., 2010, 12, 2698 CrossRef CAS PubMed.
- D. R. Armstrong, P. Garcia-Alvarez, A. R. Kennedy, R. E. Mulvey and J. A. Parkinson, Angew. Chem., Int. Ed., 2010, 49, 3185 CrossRef CAS PubMed.
- T. Tatic, K. Meindl, J. Henn, S. K. Pandey and D. Stalke, Chem. Commun., 2010, 46, 4562 RSC.
- D. Y. Li, I. Keresztes, R. Hopson and P. G. Williard, Acc. Chem. Res., 2009, 42, 270 CrossRef CAS PubMed.
- D. Y. Li, G. Kagan, R. Hopson and P. G. Williard, J. Am. Chem. Soc., 2009, 131, 5627 CrossRef CAS PubMed.
- B. Lecachey, N. Duguet, H. Oulyadi, C. Fressigné, A. Harrison-Marchand, Y. Yamamoto, K. Tomioka and J. Maddaluno, Org. Lett., 2009, 11, 1907 CrossRef CAS PubMed.
- J. Liu, D. Y. Li, C. Z. Sun and P. G. Williard, J. Org. Chem., 2008, 73, 4045 CrossRef CAS PubMed.
- D. Y. Li, C. Z. Sun, J. Liu, R. Hopson, W. B. Li and P. G. Williard, J. Org. Chem., 2008, 73, 2373 CrossRef CAS PubMed.
- A. Macchioni, G. Ciancaleoni, C. Zuccaccia and D. Zuccaccia, Chem. Soc. Rev., 2008, 37, 479 RSC.
- X. He, J. J. Morris, B. C. Noll, S. N. Brown and K. W. Henderson, J. Am. Chem. Soc., 2006, 128, 13599 CrossRef CAS PubMed.
- T. Megyes, H. Jude, T. Grósz, I. Bakó, T. Radnai, G. Tárkányi, G. Pálinkás and P. J. Stang, J. Am. Chem. Soc., 2005, 127, 10731 CrossRef CAS PubMed.
- I. Keresztes and P. G. Williard, J. Am. Chem. Soc., 2000, 122, 10228 CrossRef CAS.
- A. Pichota, P. S. Pregosin, M. Valentini, M. Wörle and D. Seebach, Angew. Chem., Int. Ed., 2000, 39, 153 CrossRef CAS.
- The standards used for DOSY were: tetramethylsilane, 1-phenylnaphthalene and 1,2,3,4-tetraphenylnaphthalene.
-
M. J. Frisch, G. W. Trucks, H. B. Schlegel, G. E. Scuseria, M. A. Robb, J. R. Cheeseman, J. A. Montgomery Jr, T. Vreven, K. N. Kudin, J. C. Burant, J. M. Millam, S. S. Iyengar, J. Tomasi, V. Barone, B. Mennucci, M. Cossi, G. Scalmani, N. Rega, G. A. Petersson, H. Nakatsuji, M. Hada, M. Ehara, K. Toyota, R. Fukuda, J. Hasegawa, M. Ishida, T. Nakajima, Y. Honda, O. Kitao, H. Nakai, M. Klene, X. Li, J. E. Knox, H. P. Hratchian, J. B. Cross, V. Bakken, C. Adamo, J. Jaramillo, R. Gomperts, R. E. Stratmann, O. Yazyev, A. J. Austin, R. Cammi, C. Pomelli, J. W. Ochterski, P. Y. Ayala, K. Morokuma, G. A. Voth, P. Salvador, J. J. Dannenberg, V. G. Zakrzewski, S. Dapprich, A. D. Daniels, M. C. Strain, O. Farkas, D. K. Malick, A. D. Rabuck, K. Raghavachari, J. B. Foresman, J. V. Ortiz, Q. Cui, A. G. Baboul, S. Clifford, J. Cioslowski, B. B. Stefanov, G. Liu, A. Liashenko, P. Piskorz, I. Komaromi, R. L. Martin, D. J. Fox, T. Keith, M. A. Al-Laham, C. Y. Peng, A. Nanayakkara, M. Challacombe, P. M. W. Gill, B. Johnson, W. Chen, M. W. Wong, C. Gonzalez and J. A. Pople, in Gaussian 03, Revision C.02, Gaussian, Inc., Wallingford, CT, 2004 Search PubMed.
- P. C. Andrikopoulos, D. R. Armstrong, W. Clegg, C. J. Gilfillan, E. Hevia, A. R. Kennedy, R. E. Mulvey, C. T. O'Hara, J. A. Parkinson and D. M. Tooke, J. Am. Chem. Soc., 2004, 126, 11612 CrossRef CAS PubMed.
- R. E. Mulvey, V. L. Blair, W. Clegg, A. R. Kennedy, J. Klett and L. Russo, Nat. Chem., 2010, 2, 588 CrossRef CAS PubMed.
- A. A. Morton, J. B. Davidson and T. R. P. Gibb, J. Am. Chem. Soc., 1942, 64, 2250 CrossRef CAS.
- H. Gilman and R. L. Bebb, J. Am. Chem. Soc., 1939, 31, 109 CrossRef.
- V. L. Blair, W. Clegg, R. E. Mulvey and L. Russo, Inorg. Chem., 2009, 48, 8863 CrossRef CAS PubMed.
- W. Clegg, S. H. Dale, E. Hevia, L. M. Hogg, G. W. Honeyman, R. E. Mulvey and C. T. O'Hara, Angew. Chem., Int. Ed., 2006, 45, 6548 CrossRef CAS PubMed.
- M. Schlosser, J. H. Choi and S. Takagishi, Tetrahedron, 1990, 46, 5633 CrossRef CAS.
- F. Zeng, Y. S. Su and C. F. Chen, Sci. China, Ser. B: Chem., 2012, 10, 2069 CrossRef.
- P. E. Georghiou, Z. Li, M. Ashram, S. Chowdhury, S. Mizyed, A. H. Tran, H. Al-Saraierh and D. O. Miller, Synlett, 2005, 879 CrossRef CAS.
- M. Medarde, A. B. S. Maya and C. J. Pérez-Melero, J. Enzyme Inhib. Med. Chem., 2004, 19, 521 CrossRef CAS PubMed.
- S. Armstrong and A. R. Merril, Anal. Biochem., 2001, 292, 26 CrossRef CAS PubMed.
- X. Xie and M. C. Kozlowski, Org. Lett., 2001, 3, 2661 CrossRef CAS PubMed.
- M. D. Watson, A. Fechtenkötter and K. Müllen, Chem. Rev., 2001, 101, 1267 CrossRef CAS PubMed.
- T. Ukita, Y. Nakamura, A. Kubo, Y. Yamamoto, M. Takahashi, J. Kotera and T. Ikeo, J. Med. Chem., 1999, 42, 1293 CrossRef CAS PubMed.
- R. S. Ward, Nat. Prod. Rep., 1995, 12, 183 RSC.
- J. M. Trujillo, R. E. Jorge, E. Navarro and J. Boada, Phytochemistry, 1990, 29, 2991 CrossRef CAS.
- B. L. Seong and M. H. Han, Chem. Lett., 1982, 627 CrossRef CAS.
- G. Wittig and F. Fuhrman, Chem. Ber., 1940, 73, 1139 Search PubMed.
- C. A. James, A. L. Coelho, M. Gevaert, P. Forgione and V. Snieckus, J. Org. Chem., 2009, 74, 4094 CrossRef CAS PubMed.
-
C. G. Hartung and V. Snieckus, in Modern Arene Chemistry, ed. D. Astruc, Wiley-VCH, New York, 2002, p. 330 Search PubMed.
- M. C. Whisler, S. MacNeil, V. Snieckus and P. Beak, Angew. Chem., Int. Ed., 2004, 43, 2206 CrossRef CAS PubMed.
- V. Snieckus, Chem. Rev., 1990, 90, 879 CrossRef CAS.
- E. J.-G. Anctil and V. Snieckus, J. Organomet. Chem., 2002, 653, 150 CrossRef CAS.
- P. Beak and R. A. Brown, J. Org. Chem., 1977, 42, 1823 CrossRef CAS.
- P. Beak and V. Snieckus, Acc. Chem. Res., 1982, 15, 306 CrossRef CAS.
- M. Reuman and A. I. Meyers, Tetrahedron, 1985, 51, 837 CrossRef.
- M. P. Sibi and V. Snieckus, J. Org. Chem., 1983, 48, 1935 CrossRef CAS.
- W. Führer and H. W. Gschwend, J. Org. Chem., 1979, 44, 1133 CrossRef.
-
J. Clayden, Organolithiums: Selectivity for Synthesis, Pergamon, Oxford, 2002 Search PubMed.
-
J. Clayden, in The Chemistry of Organolithium Compounds, ed. Z. Rappoport and I. Marek, Wiley, New York, 2004 Search PubMed.
- D. Tilly, J. Magolan and J. Mortier, Chem.–Eur. J., 2012, 18, 3804 CrossRef CAS PubMed.
- C. Schneider, E. David, A. A. Toutov and V. Snieckus, Angew. Chem., Int. Ed., 2012, 51, 2722 CrossRef CAS PubMed.
- J. Clayden, C. S. Frampton, C. McCarthy and N. Westlund, Tetrahedron, 1999, 55, 14161 CrossRef CAS.
- P. Bowles, J. Clayden, M. Helliwell, C. McCarthy, M. Tomkinson, N. Westlund and S. A. Yasin, J. Chem. Soc., Perkin Trans. 1, 1997, 2607 RSC.
- D. R. Armstrong, J. García-Álvarez, D. V. Graham, G. W. Honeyman, E. Hevia, A. R. Kennedy and R. E. Mulvey, Chem.–Eur. J., 2009, 15, 3800 CrossRef CAS PubMed.
- W. Clegg, S. H. Dale, E. Hevia, L. M. Hogg, G. W. Honeyman, R. E. Mulvey, C. T. O'Hara and L. Russo, Angew. Chem., Int. Ed., 2008, 47, 731 CrossRef CAS PubMed.
- D. R. Armstrong, W. Clegg, S. Dale, D. V. Graham, E. Hevia, L. M. Hogg, G. W. Honeyman, A. R. Kennedy and R. E. Mulvey, Chem. Commun., 2007, 598 RSC.
- W. Clegg, S. H. Dale, R. W. Harrington, E. Hevia, G. W. Honeyman and R. E. Mulvey, Angew. Chem., Int. Ed., 2006, 45, 2374 CrossRef CAS PubMed.
- W. Clegg, S. H. Dale, A. M. Drummond, E. Hevia, G. W. Honeyman and R. E. Mulvey, J. Am. Chem. Soc., 2006, 128, 7434 CrossRef CAS PubMed.
- D. R. Armstrong, W. Clegg, S. H. Dale, E. Hevia, L. M. Hogg, G. W. Honeyman and R. E. Mulvey, Angew. Chem., Int. Ed., 2006, 45, 3775 CrossRef CAS PubMed.
- P. C. Andrikopoulos, D. R. Armstrong, H. R. L. Barley, W. Clegg, S. H. Dale, E. Hevia, G. W. Honeyman, A. R. Kennedy and R. E. Mulvey, J. Am. Chem. Soc., 2005, 127, 6184 CrossRef CAS PubMed.
- V. L. Blair, L. M. Carrella, W. Clegg, J. Klett, R. E. Mulvey, E. Rentschler and L. Russo, Chem.–Eur. J., 2009, 15, 856 CrossRef CAS PubMed.
- V. L. Blair, W. Clegg, B. Conway, E. Hevia, A. Kennedy, J. Klett, R. E. Mulvey and L. Russo, Chem.–Eur. J., 2008, 14, 65 CrossRef CAS PubMed.
- J. García-Álvarez, A. R. Kennedy, J. Klett and R. E. Mulvey, Angew. Chem., Int. Ed., 2007, 46, 1105 CrossRef PubMed.
- L. M. Carrella, W. Clegg, D. V. Graham, L. M. Hogg, A. R. Kennedy, J. Klett, R. E. Mulvey, E. Rentschler and L. Russo, Angew. Chem., Int. Ed., 2007, 46, 4662 CrossRef CAS PubMed.
- L. M. Salonen, M. Ellermann and F. Diederich, Angew. Chem., Int. Ed., 2011, 50, 4808 CrossRef CAS PubMed.
- J. C. Ma and D. A. Dougherty, Chem. Rev., 1997, 97, 1303 CrossRef CAS PubMed.
- D. A. Dougherty, Science, 1996, 271, 163 CAS.
- W. Clegg, B. Conway, P. García-Álvarez, A. R. Kennedy, R. E. Mulvey, L. Russo, J. Sassmannshausen and T. Tuttle, Chem.–Eur. J., 2009, 15, 10702 CrossRef CAS PubMed.
- B. Gehrhus, P. H. Hitchcock, A. R. Kennedy, M. F. Lappert, R. E. Mulvey and P. J. A. Rodger, J. Organomet. Chem., 1999, 587, 88 CrossRef CAS.
- C. S. Shiner, A. H. Berks and A. M. Fisher, J. Am. Chem. Soc., 1988, 110, 957 CrossRef CAS.
- H. Yang, Y. Li, M. Jiang, J. Wang and H. Fu, Chem.–Eur. J., 2011, 17, 5652 CrossRef CAS PubMed.
- M. Jean, J. Renault, P. Uriac, M. Capet and P. van de Weghe, Org. Lett., 2007, 9, 3623 CrossRef CAS PubMed.
- H.-C. Shen, S. Pal, J.-J. Lian and R.-S. Liu, J. Am. Chem. Soc., 2003, 125, 15762 CrossRef CAS PubMed.
- L. Di Bari, G. Pescitelli, F. Marchetti and P. Salvadori, J. Am. Chem. Soc., 2000, 122, 6395 CrossRef CAS.
- The preparation of 1,4-diiodonaphtahlene is reported in this paper but no characterization is provided: J. Barluenga, M. Trincado, E. Rubio and J. M. González, Angew. Chem., Int. Ed., 2006, 45, 3140 CrossRef CAS PubMed.
Footnote |
† Electronic supplementary information (ESI) available. CCDC [964188–964192]. For ESI and crystallographic data in CIF or other electronic format see DOI: 10.1039/c3sc52816b |
|
This journal is © The Royal Society of Chemistry 2014 |