DOI:
10.1039/C3SC52451E
(Edge Article)
Chem. Sci., 2014,
5, 333-340
Application of diazene-directed fragment assembly to the total synthesis and stereochemical assignment of (+)-desmethyl-meso-chimonanthine and related heterodimeric alkaloids†
Received
31st August 2013
, Accepted 27th September 2013
First published on 27th September 2013
Abstract
We describe the first application of our methodology for heterodimerization via diazene fragmentation towards the total synthesis of (−)-calycanthidine, meso-chimonanthine, and (+)-desmethyl-meso-chimonanthine. Our syntheses of these alkaloids feature an improved route to C3a-aminocyclotryptamines, an enhanced method for sulfamide synthesis and oxidation, in addition to a late-stage diversification leading to the first enantioselective total synthesis of (+)-desmethyl-meso-chimonanthine and its unambiguous stereochemical assignment. This versatile strategy for directed assembly of heterodimeric cyclotryptamine alkaloids has broad implications for the controlled synthesis of higher order derivatives with related substructures.
Introduction
Alkaloids comprising multiple cyclotryptamine units adjoined at C3a and C7 junctures constitute a large family of structurally fascinating natural products, which display a wide range of biological activities1 and possess retrosynthetically challenging and sterically crowded quaternary linkages. Moreover, in certain cases, the synthetic challenge is exacerbated by the heterodimeric C3a–C3a′ connectivity (Fig. 1). Significant advances have been made in the assembly of Csp2–Csp3,2,3 Csp3–Csp3,4–6 and N–Csp37 linkages in cyclotryptamine-based alkaloids. Recently, we reported a general strategy for the selective late stage C–C bond construction at the C3a quaternary stereocenters of two dissimilar cyclotryptamine subunits.5e Herein, we report the first application of our diazene5e,8 based heterodimerization strategy for the total synthesis of (−)-calycanthidine (1),9meso-chimonanthine (2),10 and (+)-desmethyl-meso-chimonanthine (DMMC, 3), an alkaloid with previously undefined stereochemistry.11 We sought a unified approach for the selective synthesis of these three natural products as a critical demonstration of the broader applicability of our diazene-based fragment-coupling chemistry for the synthesis of more complex natural products such as (−)-idiospermuline (4)12 and (+)-caledonine (5).11 Our syntheses feature an improved route to C3a-aminocyclotryptamines, an enhanced method for sulfamide synthesis and oxidation, in addition to late-stage diversification allowing access to both enantiomers of DMMC (3) from a single heterodimeric intermediate, resulting in the first enantioselective total synthesis of (+)-(3) and its unambiguous stereochemical assignment.
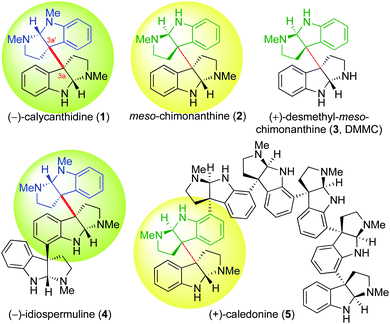 |
| Fig. 1 Representative cyclotryptamine-based natural products. | |
The dimeric, heterodimeric, and oligomeric cyclotryptamine alkaloids have been found to possess an assortment of impressive biological activities, ranging from analgesic, antiviral, antifungal, and antibacterial properties, to cytotoxicity against human cancer cell lines.11,13 (−)-Calycanthidine (1) was originally isolated in 1938 from Calycanthus floridus,9 with the structure being fully elucidated in 1962.14 Overman and co-workers' concise enantioselective synthesis of (−)-1via diastereoselective dialkylation allowed for the assignment of its absolute stereochemistry.2a,bMeso-chimonanthine (2), originally isolated in 1961 from Chimonanthus fragrans10a but not fully identified as a natural isolate until 1964,4d has been synthesized previously via the unselective oxidative dimerization of tryptamine derivatives.2g,4a,c–f,m–o Overman and co-workers have reported the selective synthesis of meso-chimonanthine (2) via a reductive dialkylation or a double Heck cyclization to secure the vicinal quaternary stereocenters.4i,k,l (+)-Desmethyl-meso-chimonanthine (3) was initially isolated in 1999 from the Rubiaceae Psychotria lyciiflora.11,15 However, the absolute stereochemistry was not assigned in the isolation report.
Retrosynthetic analysis
A central objective of our retrosynthetic approach to (−)-calycanthidine (1), meso-chimonanthine (2), (+)- and (−)-desmethyl-meso-chimonanthine (DMMC, 3) was to establish a general strategy reliant on the late-stage directed assembly of versatile precursors with potential for application to more complex cyclotryptamine-containing alkaloids. We envisioned accessing these four alkaloids from only two heterodimers 6 and 7 that would in turn be prepared from enantiomerically enriched C3a-aminocyclotryptamines 10–12 (Scheme 1). Whereas site specific methyl amine synthesis on the “chiral”-heterodimer 6 would allow access to (−)-calycanthidine (1), a similar strategic introduction of methyl units onto “meso”-heterodimer 7 would readily afford meso-chimonanthine (2) as well as both enantiomers of DMMC (3). The vicinal quaternary stereocenters bearing the C3a–C3a′ linkage of the key heterodimers 6 and 7 would be secured from diazenes 8 and 9, respectively, via implementation of our diazene-directed heterodimerization strategy using C3a-aminocyclotryptamines 10–12.5e,16 Owing to our knowledge of the relatively weak nature of the benzylic C3a–H bond of related cyclotryptamines,1c,5a,e we saw an opportunity for examination of the latest advances in intermolecular C–H amination for the synthesis of amines 10–12.17 We envision that the optimization and implementation of our planned strategy for the total synthesis of these heterodimeric cyclotryptamine natural products will serve as the foundation for further application to related complex natural products.
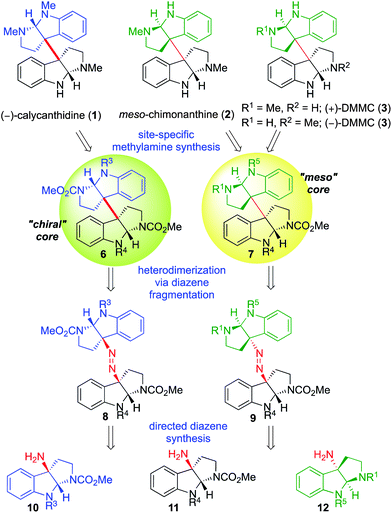 |
| Scheme 1 Retrosynthetic analysis of (−)-calycanthidine (1), meso-chimonanthine (2), (+)- and (−)-desmethyl-meso-chimonanthine (3, DMMC). | |
Synthesis of C3a-aminocyclotryptamines via catalytic intermolecular C–H amination
Given the importance of the C3a-aminocyclotryptamines 10–12 to the success of our planned synthesis, we sought improved methods to access the desired amines.5e,18 With this in mind, we turned our attention to rhodium-catalyzed C–H amination for the introduction of the required C3a-amine functional group. Based on our previous success in selective benzylic bromination of similar C3a–H cyclotryptamines,5a,e we hypothesized that the benzylic C3a–H would serve as an ideal substrate for rhodium-catalyzed C–H amination.19–21 Moreover, we believed that the resulting sulfamate ester obtained from the C–H amination might provide an opportunity for rapid synthesis of the desired mixed sulfamides by removing the need to prepare the more sensitive sulfamoyl chlorides22 used in our previous report.5e Indeed, as a separate part of our studies directed at efficient synthesis of diazenes, 2-hydroxyphenyl23 and 4-nitrophenyl24 sulfamate esters had proven effective coupling partners for entry to various sulfamide derivatives.25 In order to test these hypotheses, cyclotryptamine (−)-14 was synthesized from the cyclotryptophan derivative (+)-13 in an 86% yield via hydrolysis of the ester, Barton ester formation, and subsequent photodecarboxylation (Scheme 2A).26
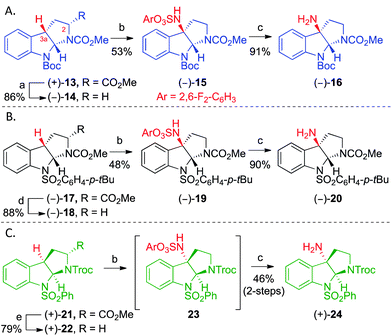 |
| Scheme 2 C–H amination, synthesis of amines (−)-16, (−)-20 and (+)-24. Conditions: (a) (i) 5 N KOH (aq.), MeOH, 23 °C; (ii) TCFH, thiopyridine N-oxide, DMAP, Et3N, THF, then t-BuSH, hν, 23 °C, 86%; (b) Rh2(esp)2, H2NSO3Ar, PhI(OAc)2, Ph(CH3)2CCO2H, MgO, 5 Å MS, i-PrOAc, 23 °C, for 14 → 15, 53%, 18 → 19, 48%; (c) pyridine, MeCN, H2O, 70 °C, for 15 → 16, 91%, 19 → 20, 90%, 22 → 24, 46% (2 steps); (d) (i) 5 N KOH (aq.), MeOH, 23 °C; (ii) (COCl)2, DMF, CH2Cl2, 23 °C; (iii) (Me3Si)3SiH, AIBN, PhMe, 80 °C, 88%; (e) (i) Me3SnOH, DCE, 80 °C; (ii) TCFH, thiopyridine N-oxide, DMAP, Et3N, THF, then t-BuSH, hν, 23 °C (79%, 2 steps); DMF = dimethylformamide, AIBN = azobisisobutyronitrile, TCFH = N,N,N′,N′-tetramethylchloroformamidinium hexafluorophosphate, DMAP = 4-(dimethylamino)pyridine, THF = tetrahydrofuran, DCE = 1,2-dichloroethane. | |
With cyclotryptamine (−)-14 in hand we were poised to examine the desired C–H amination. Utilizing the latest conditions and reagents generously provided to us by Du Bois and co-workers for selective intermolecular tertiary C–H amination19,27 we were able to obtain the desired arylsulfamate ester (−)-15 in a 53% yield. Interestingly, we observed the undesired C–H amination at C2 to be the major byproduct of the rhodium-catalyzed C–H amination reaction (10–15%).28,29 We postulate that the increased steric demands of the C3a–H cyclotryptamine (−)-14 lead to the observed competitive C2 amination.30 Hydrolysis of the sulfamate ester (−)-15 efficiently gave the desired C3a amine (−)-16 in a 91% yield. The differentially functionalized C3a-aminocyclotryptamine (−)-20 was synthesized in an analogous fashion from the tricycle (−)-17 in an overall yield of 38% over 4 steps (Scheme 2B).31 We subsequently discovered that the desired amine (+)-24 could be directly obtained from the corresponding cyclotryptamine in a two-step sequence without purification of the sulfamate ester. In the event, rhodium-catalyzed C–H amination of cyclotryptamine (+)-22 followed by treatment of the crude sulfamate ester 23 with pyridine in an acetonitrile–water mixture at 70 °C directly afforded the C3a-aminocyclotryptamine (+)-24 in a 46% yield over two-steps (Scheme 2C).
The application of selective C–H amination allows for direct access to the desired C3a-aminocyclotryptamine in only two steps from the corresponding C3a–H cyclotryptamines, eliminates the need for the preparation of azides, and precludes the requirement for prior activation as the benzylic bromide.5e Furthermore, access to sulfamate ester (−)-19 has allowed examination of its use as a coupling partner in place of our previously utilized sulfamoyl chlorides for the synthesis of mixed sulfamides (vide infra).
Sulfamide formation, oxidation and diazene fragmentation
With the desired amines in hand we were ready to access the heterodimers (−)-27 and (+)-30via directed sulfamide formation, mild oxidation, and localized diazene fragmentation (Scheme 3). In our previous report, we carried out sulfamide formation by first selectively converting one of the amines to the sulfamoyl chloride followed by treatment with the other amine to afford the desired mixed sulfamide.5e We hypothesized that arylsulfamate (−)-19, the direct product of C–H amination, could be utilized in place of the sulfamoyl chloride. We were delighted to find that exposure of sulfamate ester (−)-19 to amine (−)-16 in the presence of triethylamine in tetrahydrofuran afforded the desired sulfamide (−)-25 in an 85% yield. Notably, only a slight excess (1.2 equiv.) of sulfamate (−)-19 is required to efficiently obtain the desired product, despite the severe steric constraints inherent in these systems. This represents a significant streamlining of sulfamide synthesis as the heterodimeric sulfamide can now be directly generated from the C–H amination product without the need for formation of the more sensitive sulfamoyl chloride. We expect that this process should provide a general route to a wide range of sulfamide derivatives and the corresponding diazenes.
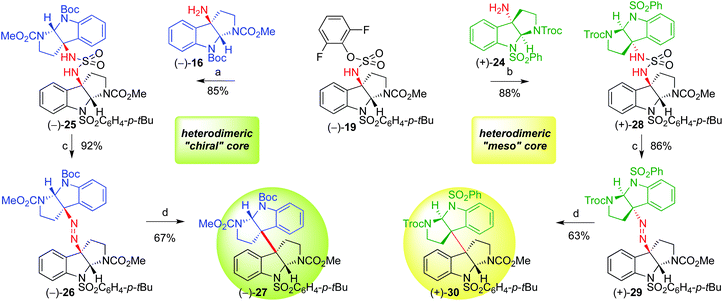 |
| Scheme 3 Synthesis of heterodimers (−)-27 and (+)-30via diazene fragmentation. Conditions: (a) Et3N, (−)-16, THF, 23 °C, 85%; (b) Et3N, (+)-24, THF, 23 °C, 88%; (c) 1,3-dichloro-5,5-dimethylhydantoin, DBU, MeOH, 23 °C, for 25 → 26, 92%, 28 → 29, 86%; (d) hν (380 nm), 23 °C, for 26 → 27, 67%, 29 → 30, 63%; DBU = 1,8-diazabicyclo[5.4.0]undec-7-ene. | |
Next, oxidation of sulfamide (−)-25 to the diazene was required.32 Our previous conditions for oxidation necessitated a large excess of oxidant and base to ensure complete conversion to the desired diazene.5e Upon further optimization of the reaction conditions we found that protic solvents gave high yields of the desired product while requiring fewer equivalents of both base and oxidant. Notably, the more electrophilic chlorinating agent 1,3-dichloro-5,5-dimethylhydantoin (DCDMH) proved ideal. Under our new and optimized conditions, treatment of sulfamide (−)-25 with 1,8-diazabicyclo[5.4.0]undec-7-ene (DBU, 5 equiv.) and DCDMH (2.5 equiv.) in methanol provided the diazene (−)-26 in a 92% isolated yield. Most likely, the alcoholic solvent increases the rate of proton transfer, leading to an increase in the rate of oxidation of the sulfamide nitrogen, thereby decreasing the undesired consumption of the oxidant by the amine base.
Initially, we sought to exploit the solvent-cage effect we observed previously by conducting the photolysis of unsymmetrical diazene (−)-26 in tert-butanol.5e However, diazene (−)-26 was found to be sparingly soluble in tert-butanol. Therefore, we explored conducting the photolysis in the solid state in the hope of increasing the localization effect and favoring the heterodimerization33 while being cognizant that an increase in the formation of disproportionation products was conceivable.33a,b We found that photolysis of the diazene (−)-26 as a thin film in the absence of solvent afforded the “chiral” heterodimer (−)-27 in a 67% yield, with minimal formation of disproportionation products, and in the absence of related cross-over products.
Applying a similar strategy, we were able to access the desired “meso” heterodimer (+)-30. Treatment of the sulfamate ester (−)-19 with triethylamine in the presence of tricyclic amine (+)-24 afforded the new mixed sulfamide (+)-28 in an 88% yield. Subsequent oxidation under our newly-optimized conditions for diazene formation provided the unsymmetrical diazene (+)-29 in an 86% yield. Photolysis of (+)-29 as a thin film selectively provided the “meso“ heterodimer (+)-30 in a 63% yield without any detectable cross-over products. Importantly, the application of our diazene-based strategy to heterodimer assembly has allowed the rapid and selective synthesis of two strategically functional heterodimers [(−)-27 and (+)-30] for selective late-stage methylamine synthesis, from two distinct amines [(−)-16 and (+)-24] and a single versatile sulfamate ester (19).
Total syntheses of (−)-calycanthidine (1), meso-chimonanthine (2), (+)- and (−)-desmethyl-meso-chimonanthine (3)
With access to both desired heterodimers, we were poised to access (−)-calycanthidine (1, Scheme 4) via selective functionalization of “chiral” heterodimer (−)-27. Treatment of heterodimer 27 with trifluoroacetic acid selectively removed the tert-butyl carbamate (90% yield), allowing the ensuing reductive methylation of the resultant indoline (−)-31 to afford the desired N-methyl indoline (−)-32 in a 90% yield. Exposure of (−)-32 to sodium amalgam in methanol, followed by reduction of the methylcarbamate functional groups of indoline (−)-33, provided synthetic (−)-calycanthidine (1) in a 65% yield over the final two steps. All 1H and 13C NMR data, as well as optical rotation (observed [α]24D = −289.6, c = 1.54, MeOH; literature [α]20D = −285.1, c = 1.992, MeOH), for synthetic (−)-1 were in agreement with literature data.2a,b,9
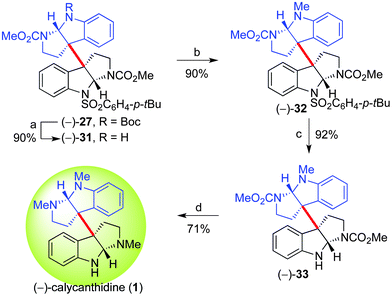 |
| Scheme 4 Total synthesis of (−)-calycanthidine (1). Conditions: (a) CH2Cl2, TFA, 90%; (b) formalin, NaBH3CN, AcOH, MeCN, 23 °C, 90%; (c) Na(Hg), NaH2PO4, MeOH, 23 °C, 92%; (d) Red-Al, PhMe, 110 °C, 71%; TFA = trifluoroacetic acid, Red-Al = sodium bis(2-methoxyethoxy)aluminium hydride. | |
Having successfully employed “chiral” heterodimer (−)-27en route to (−)-calycanthidine (1), we hoped to exploit a similar strategy utilizing the “meso” heterodimer (+)-30 for the synthesis of meso-chimonanthine (2, Scheme 5) in addition to (+)-DMMC (3) and (−)-DMMC (3). While attempts to reduce both carbamate functional groups of heterodimer (+)-30 to the corresponding methyl amines resulted in removal of the trichloroethyl carbamate, treatment of heterodimer (+)-30 with lithium triethylborohydride led exclusively to the formation of ethyl carbamate (+)-34 in an 84% yield.34 Although unexpected, the formation of intermediate (+)-34 proved critically beneficial as it permitted the selective removal of the arenesulfonyl groups to afford the free indoline (−)-35 in an 80% yield. A final reduction of the two carbamate functional groups selectively gave meso-chimonanthine (2) in a 91% yield. All 1H and 13C NMR data for our synthetic 2 were identical in all respects to the literature values for meso-chimonanthine (2).4 Intrigued by the presence of related reorganized natural products in the chiral chimonanthine series,4k,5a,35 we investigated the rearrangement of meso-chimonanthine (2) to the corresponding meso-calycanthine (36, Scheme 5). In the event, the acid-mediated rearrangement of meso-chimonanthine (2) was explored by heating a solution of 2 in deuterium oxide and acetic acid-d6 to 95 °C and monitoring the progress using in situ1H NMR.36 After 24 h, >90% consumption of meso-chimonanthine was observed, affording a mixture of meso-calycanthine (36) as well as products related to fragmentation of the fragile C3a–C3a′ bond. Subsequent basic work-up and purification by flash column chromatography provided meso-calycanthine (36) in a 36% yield. Resubmission of isolated meso-calycanthine (36) to the acidic conditions resulted in no change, suggesting that in contrast to the equilibrium observed between chimonanthine and calycanthine (15
:
85),5a the thermodynamic equilibrium between 2 and 36 strongly favors meso-calycanthine (36).
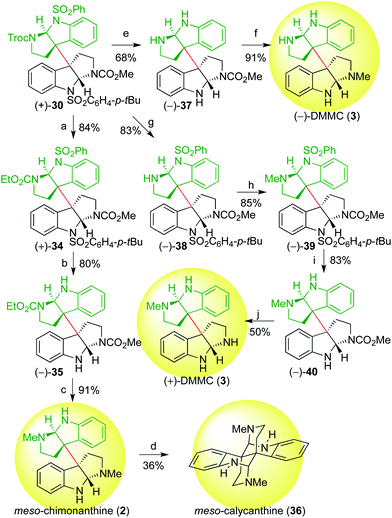 |
| Scheme 5 Total synthesis of meso-chimonanthine (2) and (+)- and (−)-desmethyl-meso-chimonanthine (DMMC, 3). Conditions: (a) LiEt3BH, THF, 65 °C, 84%; (b) Na(Hg), NaH2PO4, MeOH, 23 °C, 80%; (c) Red-Al, PhMe, 110 °C, 91%; (d) D2O, CD3CO2D, 95 °C, 24 h, 36%; (e) Na(Hg), NaH2PO4, MeOH, 23 °C, 68%; (f) Red-Al, PhMe, 110 °C, 91%; (g) Zn, AcOH, MeOH, 23 °C, 83%; (h) formalin, NaCNBH3, AcOH, MeCN, 23 °C, 85%; (i) Na(Hg), NaH2PO4, MeOH, 23 °C, 83%; (j) 5 N NaOH (aq.), MeOH, 65 °C, 50%. | |
We next turned our focus to the synthesis and assignment of absolute stereochemistry of (+)-DMMC (3) from the same versatile “meso” heterodimer (+)-30. Our ability to unequivocally assign the absolute stereochemistry of (+)-DMMC (3) centered on our capacity to access both enantiomers of this previously unassigned natural alkaloid. We found that exposure of heterodimer (+)-30 to sodium amalgam in methanol resulted in removal of the trichloroethyl carbamate along with the sulfonyl groups in a single step, to afford triamine (−)-37 in a 68% yield (Scheme 5). Subsequent reduction of the methyl carbamate group with Red-Al afforded the desired methyl amine 3 in a 91% yield. All 1H and 13C NMR data for synthetic 3 were identical in all respects to the literature values for (+)-DMMC (3).11 However, the observed optical rotation of our synthetic 3 was opposite in sign to that of the natural product (observed [α]24D = −1.8, c = 0.20, EtOH; literature [α]25D = +0.5, c = 1, EtOH)11 and therefore we assigned the depicted structure as (−)-DMMC (3). Importantly, careful and repeated analysis of the same sample over 2 h indicated a steady decrease in the magnitude of the observed rotation with eventual inversion of the sign. Both 1H NMR and TLC analysis of the sample indicated minor decomposition (<5%) of the natural product during this process, which is likely to be responsible for the gradual drift of the optical rotation value.37 We hypothesize that this observed variation in optical rotation over time is responsible for the discrepancy between the absolute value of the optical rotation of our sample and that of the natural isolate.11 Nevertheless, we are fully confident in the high quality of our sample, its optical rotation, and its absolute stereochemistry as depicted in Scheme 5.
To further clarify the absolute stereochemistry of (+)- and (−)-DMMC (3), and to confirm our observations regarding sample sensitivity as described above, we sought to access (+)-DMMC (3) by exploiting the versatility of the “meso” heterodimer (+)-30 (Scheme 5). Enantioselective total synthesis of both (+)- and (−)-DMMC (3) from heterodimer (+)-30, would allow unambiguous confirmation of the absolute stereochemistry of DMMC (3) and also highlight the utility of heterodimer (+)-30 as a synthetic intermediate for the preparation of these cyclotryptamine alkaloids. Selective trichloroethyl carbamate removal with zinc in a mixture of acetic acid and methanol provided the free amine (−)-38 in an 83% yield. Subsequent reductive methylation with formalin and sodium cyanoborohydride (85% yield), followed by removal of the arenesulfonyl groups to afford the free indoline (−)-40 (83% yield) and hydrolysis of the methyl carbamate group provided the first synthetic sample of (+)-DMMC (3).11 All 1H and 13C NMR data for synthetic 3 were identical to the literature values for (+)-DMMC (3) and the optical rotation of our synthetic (+)-3 was consistent with that reported in the literature (observed [α]24D = +2.7, c = 0.13 EtOH). Importantly, the same observations regarding the critical purity of the sample in optical activity determination were observed once again. Having accessed both enantiomers of the natural product from known chiral feedstock we can confidently assign the absolute configuration of (+)- and (−)-desmethyl-meso-chimonanthine (3) as shown in Scheme 5.38
Conclusions
Application of our diazene-based method for heterodimerization has allowed the total syntheses of (−)-calycanthidine (1), meso-chimonanthine (2), and (+)- and (−)-desmethyl-meso-chimonanthine (3). Our synthetic route takes advantage of the inherent symmetry found in this group of natural products and allows us to selectively access these four unique compounds from only two distinct heterodimers (27 and 30), two unique amines (16 and 24) and a sulfamate (19). Furthermore, access to (+)- and (−)-desmethyl-meso-chimonanthine (3) has allowed for its unambiguous stereochemical assignment. The successful implementation of our diazene-based heterodimerization for the synthesis of the three heterodimeric cyclotryptamine alkaloids will serve as the foundation for further application to the controlled synthesis of more complex cyclotryptamine-containing natural products such as (−)-idiospermuline (4) and (+)-caledonine (5).
Acknowledgements
We acknowledge financial support by NIH-NIGMS (GM089732). S. P. L. is grateful to the NIH for a Ruth L. Kirschstein NRSA postdoctoral fellowship (F32GM097776). We acknowledge the NSF under CCI Center for selective C–H functionalization (CHE-1205646) for support related to C–H amination chemistry. We are grateful for advice, catalysts, and reagents provided by professors J. Du Bois, H. M. L. Davies, H. Lebel, and J. L. Roizen.
Notes and references
-
(a)
G. A. Cordell and J. E Saxton, in The Alkaloids: Chemistry and Physiology, ed. R. H. F. Manske and R. G. A. Rodrigo, Academic Press, New York, 1981, vol. 20, pp. 3–294 Search PubMed;
(b)
T. Hino and M. Nakagawa, in The Alkaloids: Chemistry and Pharmacology, ed. A. Brossi, Academic Press, New York, 1989, vol. 34, pp. 1–75 Search PubMed;
(c) D. Crich and A. Banerjee, Acc. Chem. Res., 2007, 40, 151 CrossRef CAS PubMed;
(d) A. Steven and L. E. Overman, Angew. Chem., Int. Ed., 2007, 46, 5488 CrossRef CAS PubMed.
-
(a) L. E. Overman and E. A. Peterson, Angew. Chem., Int. Ed., 2003, 42, 2525 CrossRef CAS PubMed;
(b) L. E. Overman and E. A. Peterson, Tetrahedron, 2003, 59, 6905 CrossRef CAS;
(c) S. P. Govek and L. E. Overman, Tetrahedron, 2007, 63, 8499 CrossRef CAS PubMed;
(d) L. E. Overman and Y. Shin, Org. Lett., 2007, 9, 339 CrossRef CAS PubMed;
(e) J. J. Kodanko, S. Hiebert, E. A. Peterson, L. Sung, L. E. Overman, V. de Moura Linck, G. C. Goerck, T. A. Amador, M. B. Leal and E. Elisabetsky, J. Org. Chem., 2007, 72, 7909 CrossRef CAS PubMed;
(f) A. W. Schammel, B. W. Boal, L. Zu, T. Mesganaw and N. K. Garg, Tetrahedron, 2010, 66, 4687 CrossRef CAS PubMed;
(g) R. H. Snell, R. L. Woodward and M. C. Willis, Angew. Chem., Int. Ed., 2011, 50, 9116 CrossRef CAS PubMed;
(h) J. E. DeLorbe, S. Y. Jabri, S. M. Mennen, L. E. Overman and F.-L. Zhang, J. Am. Chem. Soc., 2011, 133, 6549 CrossRef CAS PubMed;
(i) L. Furst, J. M. R. Narayanam and C. R. J. Sephenson, Angew. Chem., Int. Ed., 2011, 50, 9655 CrossRef CAS PubMed;
(j) M. E. Kieffer, K. V. Chuang and S. E. Reisman, J. Am. Chem. Soc., 2013, 135, 5557 CrossRef CAS PubMed.
-
(a) J. Kim and M. Movassaghi, J. Am. Chem. Soc., 2011, 133, 14940 CrossRef CAS PubMed;
(b) N. Boyer and M. Movassaghi, Chem. Sci., 2012, 3, 1798 RSC;
(c) A. Coste, J. Kim, T. C. Adams and M. Movassaghi, Chem. Sci., 2013, 4, 3191 RSC.
-
(a) J. B. Hendrickson, R. Rees and R. Göschke, Proc. Chem. Soc., London, 1962, 383 CAS;
(b) T. Hino and S.-I. Yamada, Tetrahedron Lett., 1963, 4, 1757 CrossRef;
(c) J. B. Hendrickson, R. Göschke and R. Rees, Tetrahedron, 1964, 20, 565 CrossRef CAS;
(d) A. I. Scott, F. McCapra and E. S. Hall, J. Am. Chem. Soc., 1964, 86, 302 CrossRef CAS;
(e) E. S. Hall, F. McCapra and A. J. Scott, Tetrahedron, 1967, 23, 4131 CrossRef CAS;
(f) T. Hino, S. Kodato, K. Takahashi, H. Yamaguchi and M. Nakagawa, Tetrahedron Lett., 1978, 19, 4913 CrossRef;
(g) M. Nakagawa, H. Sugumi, S. Kodato and T. Hino, Tetrahedron Lett., 1981, 22, 5323 CrossRef CAS;
(h) C.-L. Fang, S. Horne, N. Taylor and R. Rodrigo, J. Am. Chem. Soc., 1994, 116, 9480 CrossRef CAS;
(i) J. T. Link and L. E. Overman, J. Am. Chem. Soc., 1996, 118, 8166 CrossRef CAS;
(j) M. Somei, N. Oshikiri, M. Hasegawa and F. Yamada, Heterocycles, 1999, 51, 1237 CrossRef CAS;
(k) L. E. Overman, D. V. Paone and B. A. Sterns, J. Am. Chem. Soc., 1999, 121, 7702 CrossRef CAS;
(l) L. E. Overman, J. F. Larrow, B. A. Sterns and J. M. Vance, Angew. Chem., Int. Ed., 2000, 39, 213 CrossRef CAS;
(m) L. Verotta, F. Orsini, M. Sbacchi, M. A. Scheildler, T. A. Amador and E. Elisabetsky, Bioorg. Med. Chem., 2002, 10, 2133 CrossRef CAS;
(n) H. Ishikawa, H. Takayama and N. Aimi, Tetrahedron Lett., 2002, 43, 5637 CrossRef CAS;
(o) Y. Matsuda, M. Kitajima and H. Takayama, Heterocycles, 2005, 65, 1031 CrossRef CAS PubMed;
(p) S. Tadano, Y. Mukaeda and H. Ishidawa, Angew. Chem., Int. Ed., 2013, 52, 7990 CrossRef CAS PubMed.
-
(a) M. Movassaghi and M. A. Schmidt, Angew. Chem., Int. Ed., 2007, 46, 3725 CrossRef CAS PubMed;
(b) M. Movassaghi, M. A. Schmidt and J. A. Ashenhurst, Angew. Chem., Int. Ed., 2008, 47, 1485 CrossRef CAS PubMed;
(c) J. Kim, J. A. Ashenhurst and M. Movassaghi, Science, 2009, 324, 238 CrossRef CAS PubMed;
(d) J. Kim and M. Movassaghi, J. Am. Chem. Soc., 2010, 132, 14376 CrossRef CAS PubMed;
(e) M. Movassaghi, O. K. Ahmad and S. P. Lathrop, J. Am. Chem. Soc., 2011, 133, 13002 CrossRef CAS PubMed.
- For other applications of our chemistry in the synthesis of cyclotryptamine-based alkaloids, see:
(a) C. Perez-Balado and A. R. de Lera, Org. Lett., 2008, 10, 3701 CrossRef CAS PubMed;
(b) C. Perez-Balado, P. Rodríguez-Graña and A. R. de Lera, Chem.–Eur. J., 2009, 15, 9928 CrossRef CAS PubMed;
(c) E. Iwasa, Y. Hamashima, S. Fujishiro, E. Higuchi, A. Ito, M. Yoshida and M. Sodeoka, J. Am. Chem. Soc., 2010, 132, 4078 CrossRef CAS PubMed;
(d) K. Foo, T. Newhouse, I. Mori, H. Takayama and P. S. Baran, Angew. Chem., Int. Ed., 2011, 50, 2716 CrossRef CAS PubMed.
- For inventive syntheses of C3sp3–N1′ dimers, see:
(a) Y. Matsuda, M. Kitajima and H. Takayama, Org. Lett., 2008, 10, 125 CrossRef CAS PubMed;
(b) T. Newhouse and P. S. Baran, J. Am. Chem. Soc., 2008, 130, 10886 CrossRef CAS PubMed;
(c) V. R. Espejo and J. D. Rainier, J. Am. Chem. Soc., 2008, 130, 12894 CrossRef CAS PubMed;
(d) T. Newhouse, C. A. Lewis and P. S. Baran, J. Am. Chem. Soc., 2009, 131, 6360 CrossRef CAS PubMed;
(e) V. R. Espejo, X.-B. Li and J. D. Rainier, J. Am. Chem. Soc., 2010, 132, 8282 CrossRef CAS PubMed;
(f) V. R. Espejoand and J. D. Rainier, Org. Lett., 2010, 12, 2154 CrossRef PubMed;
(g) C. Perez-Balado and A. R. de Lera, Org. Biomol. Chem., 2010, 8, 5179 RSC;
(h) I. Villanueva-Margalef, D. E. Thurston and G. Zinzalla, Org. Biomol. Chem., 2010, 8, 5294 RSC;
(i) J. D. Rainier and V. R. Espejo, Isr. J. Chem., 2011, 51, 473 CrossRef CAS.
- For representative examples of intramolecular carbon–carbon bond formation using dialkyl diazene intermediates in natural product synthesis, see:
(a) R. D. Little, G. L. Carroll and J. L. Pettersen, J. Am. Chem. Soc., 1983, 105, 928 CrossRef CAS;
(b) R. D. Little, Chem. Rev., 1996, 96, 93 CrossRef CAS PubMed;
(c) V. Mascitti and E. J. Corey, J. Am. Chem. Soc., 2004, 126, 15664 CrossRef CAS PubMed;
(d) P. A. Wender, J.-M. Kee and J. M. Warrington, Science, 2008, 320, 649 CrossRef CAS PubMed.
- G. Barger, A. Jacob and J. Madinaveitia, Recl. Trav. Chim. Pays-Bas, 1938, 57, 548 CrossRef CAS ; (−)-calycanthidine was also isolated more recently from the seeds of Chimonanthus praecox., see:
(a) H. Takayama, Y. Matsuda, K. Masubuchi, A. Ishida, M. Kitajima and N. Aimi, Tetrahedron, 2004, 60, 893 CrossRef CAS PubMed.
-
(a) H. F. Hodson, B. Robinson and G. F. Smith, Proc. Chem. Soc., London, 1961, 465 CAS;
(b) Y. Adjibade, B. Weniger, J. D. Quirion, B. Kuballa, P. Cabalion and R. Anton, Phytochemistry, 1992, 31, 317 CrossRef CAS.
- V. Jannic, F. Guéritte, O. Laprévote, L. Serani, M.-T. Martin, T. Sévenet and P. Potier, J. Nat. Prod., 1999, 62, 838 CrossRef CAS PubMed.
- R. K. Duke, R. B. Allan, G. A. R. Johnston, K. N. Mewett, A. D. Mitrovic, C. C. Duke and T. W. Hambley, J. Nat. Prod., 1995, 58, 1200 CrossRef CAS.
-
(a) Y. Adjibadé, H. Saad, B. Kuballa, J. P. Beck, T. Sévent, P. Cabalion and R. Anton, J. Ethnopharmacol., 1990, 29, 127 CrossRef;
(b) H.-E. Saad, S. H. El-Sharkawy and W. T. Shies, Planta Med., 1995, 61, 313 CrossRef CAS PubMed;
(c) T. A. Amador, L. Verotta, D. S. Nunes and E. Elisabetsky, Planta Med., 2000, 66, 770 CrossRef CAS PubMed.
- J. E. Saxton, W. G. Bardsley and G. F. Smith, Proc. Chem. Soc., London, 1962, 148 CAS.
- For a racemic synthesis of (±)-desmethyl-meso-chimonanthine, see: C. Menozzi, P. I. Dalko and J. Cossy, Chem. Commun., 2006, 4638 RSC.
- For leading references on the fragmentation of dialkyl diazenes, see:
(a) L. Horner and W. Naumann, Justus Liebigs Ann. Chem., 1954, 587, 93 CrossRef;
(b) S. F. Nelsen and P. D. Bartlett, J. Am. Chem. Soc., 1966, 88, 137 CrossRef CAS;
(c) S. F. Nelsen and P. D. Bartlett, J. Am. Chem. Soc., 1966, 88, 143 CrossRef CAS;
(d) J. W. Timberlake, J. Alender, A. W. Garner, M. L. Hodges, C. Özmeral and S. Szilagyi, J. Org. Chem., 1981, 46, 2082 CrossRef CAS;
(e) M. T. Hossain and J. W. Timberlake, J. Org. Chem., 2001, 66, 6282 CrossRef CAS PubMed; For other pioneering work in the area of diazene chemistry see:
(f) N. A. Porter and L. J. Marnett, J. Am. Chem. Soc., 1973, 95, 4361 CrossRef CAS;
(g) P. Gölitz and A. de Meijere, Angew. Chem., Int. Ed. Engl., 1977, 16, 854 CrossRef;
(h) N. A. Porter, G. R. Dubay and J. G. Green, J. Am. Chem. Soc., 1978, 100, 920 CrossRef CAS;
(i) J. E. Baldwin, R. M. Adlington, J. C. Bottaro, J. N. Kolhe, I. M. Newington and M. W. D. Perry, Tetrahedron, 1986, 42, 4235 CrossRef CAS;
(j) T. Sumiyoshi, M. Kamachi, Y. Kuwae and W. Schnabel, Bull. Chem. Soc. Jpn., 1987, 60, 77 CrossRef CAS;
(k) R. C. Neuman Jr, R. H. Grow, G. A. Binegar and H. J. Gunderson, J. Org. Chem., 1990, 55, 2682 CrossRef;
(l) P. S. Engel, L. Pan, Y. Ying and L. B. Alemany, J. Am. Chem. Soc., 2001, 123, 3706 CrossRef CAS PubMed;
(m) P. A. Hoijemberg, S. D. Karlen, C. N. Snaramé, P. F. Aramendía and M. A. García-Garibay, Photochem. Photobiol. Sci., 2009, 8, 961 RSC; For relevant reviews see:
(n) P. S. Engel and C. Steel, Acc. Chem. Res., 1973, 6, 275 CrossRef CAS;
(o) P. S. Engel, Chem. Rev., 1980, 80, 99 CrossRef CAS.
- For recent reviews on C–H amination, see:
(a) D. N. Zalatan and J. Du Bois, Top. Curr. Chem., 2010, 292, 347 CrossRef CAS;
(b) J. Du Bois, Org. Process Res. Dev., 2011, 15, 758 CrossRef CAS PubMed;
(c) J. L. Roizen, M. E. Harvey and J. Du Bois, Acc. Chem. Res., 2012, 45, 911 CrossRef CAS PubMed.
- For recent syntheses of enantioenriched C3a-amino cyclotryptamines, see:
(a) T. Benkovics, I. A. Guzei and T. P. Yoon, Angew. Chem., Int. Ed., 2010, 49, 9153 CrossRef CAS PubMed;
(b) Z. Zhang and J. C. Antilla, Angew. Chem., Int. Ed., 2012, 51, 11778 CrossRef CAS PubMed.
-
(a) C. G. Espino and J. Du Bois, Angew. Chem., Int. Ed., 2001, 40, 598 CrossRef CAS;
(b) C. G. Espino, K. Fiori Williams, M. Kim and J. Du Bois, J. Am. Chem. Soc., 2004, 126, 15378 CrossRef CAS PubMed;
(c) K. Fiori Williams and J. Du Bois, J. Am. Chem. Soc., 2007, 129, 562 CrossRef PubMed;
(d) K. Huard and H. Lebel, Chem.–Eur. J., 2008, 14, 6222 CrossRef CAS PubMed;
(e) D. N. Zalatan and J. Du Bois, J. Am. Chem. Soc., 2009, 131, 7558 CrossRef CAS PubMed;
(f) T. Kurokawa, M. Kim and J. Du Bois, Angew. Chem., Int. Ed., 2009, 48, 2777 CrossRef CAS PubMed;
(g) K. W. Fiori, C. G. Espino, B. H. Brodsky and J. Du Bois, Tetrahedron, 2009, 65, 3042 CrossRef CAS PubMed.
- For enantioselective Rh- and Ru-catalyzed C–H amination, see:
(a) J. –L. Liang, S. –X. Yuan, J. –S. Huang, W. –Y. Yu and C. –M. Che, Angew. Chem., Int. Ed., 2002, 41, 3465 CrossRef CAS;
(b) R. P. Reddy and H. M. L. Davies, Org. Lett., 2006, 8, 5013 CrossRef CAS PubMed;
(c) D. N. Zalatan and J. Du Bois, J. Am. Chem. Soc., 2008, 130, 9220 CrossRef CAS PubMed;
(d) E. Milczek, N. Boudet and S. Blakey, Angew. Chem., Int. Ed., 2008, 47, 6825 CrossRef CAS PubMed.
- For diastereoselective C–H amination using optically active nitrogen sources, see:
(a) C. Liang, C. Fruit, F. Robert-Peilard, P. Müller, P. R. H. Dodd and P. J. Dauban, J. Am. Chem. Soc., 2008, 130, 343 CrossRef CAS PubMed;
(b) F. Collet, C. Lescot, C. Liang and P. Dauban, Dalton Trans., 2010, 39, 10401 RSC;
(c) H. Lebel, C. Spitz, O. Leogane, C. Trudel and M. Parmentier, Org. Lett., 2011, 13, 5460 CrossRef CAS PubMed;
(d) H. Lebel, C. Trudel and C. Spitz, Chem. Commun., 2012, 48, 7799 RSC;
(e) C. Lescot, B. Darses, F. Collet, P. Retailleau and P. Dauban, J. Org. Chem., 2012, 77, 7232 CrossRef CAS PubMed.
- For leading references for sulfamide formation via sulfamyl chlorides, see:
(a) L. F. Audrieth and M. Sveda, J. Org. Chem., 1944, 9, 89 CrossRef CAS;
(b) N. C. Hansen, Acta Chem. Scand., 1963, 17, 2141 CrossRef CAS PubMed;
(c) G. Weiss and G. Schulze, Justus Liebigs Ann. Chem., 1969, 729, 40 CrossRef CAS;
(d) J. A. Kloek and K. L. Leschinsky, J. Org. Chem., 1976, 41, 4028 CrossRef CAS;
(e) J. W. Timberlake, W. J. Ray Jr, E. D. Stevens and K. L. Cheryl, J. Org. Chem., 1989, 54, 5824 CrossRef CAS.
-
(a) E. T. Kaiser, Acc. Chem. Res., 1970, 3, 145 CrossRef CAS;
(b) G. E. Du Bois and R. A. Stephenson, J. Org. Chem., 1980, 45, 5371 CrossRef CAS;
(c) G. E. Du Bois, J. Org. Chem., 1980, 45, 5373 CrossRef CAS;
(d) J. Micklefield and K. J. Fettes, Tetrahedron, 1998, 54, 2129 CrossRef CAS;
(e) T. Kaneko, R. S. J. Clark, N. Ohi, T. Kawahara, H. Akamatsu, F. Ozaki, A. Kamada, K. Okano, H. Yokohama, K. Muramoto, M. Ohkuro, O. Takenaka and S. Kobayashi, Chem. Pharm. Bull., 2002, 50, 922 CrossRef CAS;
(f) M. Frezza, L. Soulère, S. Reverchon, N. Guiliani, C. Jerez, Y. Queneau and A. Doutheau, Bioorg. Med. Chem., 2008, 16, 3550 CrossRef CAS PubMed;
(g) L. Gavernet, J. E. Elvira, G. A. Samaja, V. Patore, M. S. Cravero, A. Enrique, G. Estiu and L. E. Bruno-Blanch, J. Med. Chem., 2009, 52, 1592 CrossRef CAS PubMed;
(h) J.-R. Chen, L. Fu, Y.-Q. Zou, N.-J. Chang, J. Rong and W.-J. Xiao, Org. Biomol. Chem., 2011, 9, 5280 RSC.
-
(a) J. Charalambous, M. J. Frazer and W. Gerrard, J. Chem. Soc., 1964, 5480 CAS;
(b) K. J. Fettes, N. Howard, D. T. Hickman, S. A. Adah, M. R. Player, P. F. Torrence and J. Micklefield, Chem. Commun., 2000, 765 RSC;
(c) K. J. Fettes, N. Howard, D. T. Hickman, S. A. Adah, M. R. Player, P. F. Torrence and J. Micklefield, J. Chem. Soc., Perkin Trans. 1, 2002, 485 RSC.
- In preliminary studies we found 4-nitrophenyl sulfamate esters to be competent coupling partners with our tricyclic amines. However, synthesis of the 4-nitrophenyl sulfamate ester from the tricyclic amine is complicated by undesired homodimeric sulfamide formation; see ref. 24.
-
(a) D. H. R. Barton, H. A. Dowlatshahi, W. B. Motherwell and D. Villemin, J. Chem. Soc., Chem. Commun., 1980, 732 RSC;
(b) D. H. R. Barton, D. Crich and W. B. Motherwell, J. Chem. Soc., Chem. Commun., 1983, 939 RSC;
(c) D. H. R. Barton, D. Crich and W. B. Motherwell, Tetrahedron, 1985, 41, 3901 CrossRef CAS.
- J. L. Roizen, D. N. Zalatan and J. Du Bois, Angew. Chem., Int. Ed., 2013, 52, 11343 CrossRef CAS PubMed.
- For examples of the unexpected intramolecular Rh-catalyzed C–H amination of secondary C–H bonds next to nitrogen, see:
(a) S. Toumieux, P. Comapin, O. R. Martin and M. Selkti, Org. Lett., 2006, 8, 4493 CrossRef CAS PubMed;
(b) B. M. Trost, B. M. O'Boyle, W. Torres and M. K. Ameriks, Chem.–Eur. J., 2011, 17, 7890 CrossRef CAS PubMed.
- See the ESI for details.†.
- In the intramolecular Rh-catalyzed C–H amination, secondary ethereal C–H bonds react preferentially over both secondary benzylic and tertiary C–H bonds, see ref. 19g.
-
tert-Butylbenzenesulfonyl protective group was utilized to increase solubility of tricycle (−)-18 in isopropyl acetate, the optimal solvent for the rhodium-catalyzed C–H amination.
- For key references on oxidation of sulfamides, see:
(a) R. Ohme and E. Schmitz, Angew. Chem., Int. Ed. Engl., 1965, 4, 433 CrossRef;
(b) F. Golzke, G. A. Oberlinner and C. Rüchardt, Nouv. J. Chim., 1977, 1, 169 Search PubMed;
(c) H.-H. Chang and B. Weinstein, J. Chem. Soc., Perkin Trans. 1, 1977, 1601 RSC;
(d) H. Ikeda, Y. Hoshi, H. Namai, F. Tanaka, J. L. Goodman and K. Mizuno, Chem.–Eur. J., 2007, 13, 9207 CrossRef CAS PubMed.
- For recent examples of photolysis in the solid state on related systems, see:
(a) P. A. Hoijember, S. D. Karlen, C. N. Sanramé, P. F. Aramendía and M. A. García-Garibay, Photochem. Photobiol. Sci., 2009, 8, 961 RSC;
(b) S. Shiraki, A. Natarajan and M. A. García-Garibay, Photochem. Photobiol. Sci., 2011, 10, 1480 RSC;
(c) D. de Loera and M. A. García-Garibay, Org. Lett., 2012, 14, 3874 CrossRef CAS PubMed;
(d) S. Shiraki, C. S. Vogelsberg and M. A. García-Garibay, Photochem. Photobiol. Sci., 2012, 11, 1929 RSC;
(e) D. de Loera, A. Stopin and M. A. García-Gariby, J. Am. Chem. Soc., 2013, 135, 6626 CrossRef CAS PubMed.
- For examples of LiEt3BH reduction of alkyl halides, see:
(a) S. Krishnamurthy and H. C. Brown, J. Org. Chem., 1980, 45, 849 CrossRef CAS;
(b) S. Krishnamurthy and H. C. Brown, J. Org. Chem., 1983, 48, 3085 CrossRef CAS.
- For isolation of calycanthine, see: R. G. Eccles, Proc. Assoc. Am. Physicians, 1888, 84, 382 Search PubMed.
- The acid-mediated rearrangement of meso-chimonanthine under similar conditions has previously been described by Overman and co-workers, see: ref. 4i. For the acid mediated rearrangement of a related natural product containing the meso-chimonanthine substructure, see:
(a) F. Guéritte-Voegelein, T. Sévenet, J. Pusset, M. T. Adeline, B. Gillet, J. C. Beloeil, D. Guenard and P. Potier, J. Nat. Prod., 1992, 55, 923 CrossRef;
(b) A. D. Lebsack, J. T. Link, L. E. Overman and B. A. Sterns, J. Am. Chem. Soc., 2002, 124, 9008 CrossRef CAS PubMed.
- Although the specific decomposition products could not be fully identified, mass spectral analysis indicated the presence of oxindole related byproducts.
- Attempts at the acid-mediated rearrangement of both (−)-calycanthidine (1) and (+)-DMMC (3) led to exclusive fragmentation of the C3a–C3a′ bond as evidenced by the isolation of N-methyltryptamine. A similar result has been observed in the attempted acid-mediated rearrangement of (±)-folicanthine, see ref. 4h.
Footnote |
† Electronic supplementary information (ESI) available: Details for all biological assays as well as experimental procedures, spectroscopic data, and copies of 1H, 13C, and 19F NMR spectra. See DOI: 10.1039/c3sc52451e |
|
This journal is © The Royal Society of Chemistry 2014 |
Click here to see how this site uses Cookies. View our privacy policy here.