DOI:
10.1039/C3SC51798E
(Minireview)
Chem. Sci., 2014,
5, 52-57
Tailoring the structures and compositions of one-dimensional organic nanomaterials towards chemical sensing applications
Received 27th June 2013, Accepted 13th August 2013
First published on 14th August 2013
Abstract
One-dimensional (1D) organic nanostructures and their hierarchical assemblies have sparked great interest in sensing applications recently owing to convenient detection, high sensitivity and selectivity, and real-time monitoring with fast response time afforded by systems that utilize them. In this mini-review, we focus on the construction and modulation of 1D nanostructures from single- or multicomponent organic compounds, and the relevant approaches that have employed them into sensing applications. Furthermore, major obstacles and future steps towards ultimate organic nanosensors based on 1D structures are discussed.
Introduction
During the past decade, one dimensional (1D) nanostructures have received increasing interest in physical, chemical and biological sensors1 due to their high surface-to-volume ratios,2 Debye lengths comparable to the target molecule,3 and minimum power consumption.4 Organic 1D nanomaterials, inheriting the high reactivity,5 good processability,6 and high photoluminescence (PL) efficiency7 of organic molecules, offer a number of highly attractive advantages for sensing applications. For instance, gaseous analytes can be either selectively bound to their surfaces or diffused into organic matrices, which might be difficult for other materials such as inorganic semiconductor nanowires or glass nanofibers.8 Furthermore, organic nanomaterials are believed to exhibit diverse and tunable opto-electronic properties through synthesizing new molecules and controlling the size and shape of the structures,9 providing plentiful choices for sensing applications. Hence, organic 1D nanomaterials are expected to act as important potential candidates for the realization of the next generation of sensors.The main strategies currently applied in promoting the sensing applications of organic 1D nanomaterials are focused on the structure-level design by controlling the structure morphology.10 The structures of various 1D nanomaterials, such as discrete nanowires, and their hierarchical assemblies such as mesh-like nanofibers and vertically aligned nanoarrays, not only correlate with the optoelectronic properties of organic materials, but also effectively influence the absorption and diffusion behaviors of the analyte.3 Therefore, considerable efforts have been placed on optimizing the 1D structures and assemblies to achieve high sensitivity, selectivity and fast time response.11 Besides tailoring the morphology and size of the nanostructures, multicomponent materials have provided another platform to achieve superior sensing performance. Such composite nanomaterials may benefit from both the size effects of nanostructures and the energy/matter interaction between different components.12 In the following, we emphasise some recent advances in the field of nanosensors based on organic 1D nanomaterials. The relevant configurations and properties of these nanosensors are also described. The review concludes with the prospective scientific and technological challenges remaining in the field of organic 1D nanosensors.
Single-component organic 1D nanomaterials in sensing applications
A variety of organic molecules have shown promise as sensor materials because their properties can be tailored to detect a wide range of chemical compounds.13 Along with the advantages of 1D nanostructures, such as high crystallinity, high integration density and low power consumption, single-component 1D organic nanomaterials have been successfully used to detect a variety of gaseous specimens including explosives,14 organic amines,15 acids and bases,16etc. Considering the size- and shape-dependent properties of organic materials,17 morphology control plays a key role in optimizing their optoelectronic performance. Extensive efforts have been devoted to controlling 1D molecular self-assemblies for better sensing performance. Nowadays, various 1D nanostructures have been successfully fabricated by a variety of methods including self-assembly in the liquid phase,18 template methods,5a and vapor deposition.16So far, nanowires are the most commonly used 1D nanostructures in sensing applicaitions19 due to high surface-to-volume ratios and their superior crystallinity. The crystallinity of nanomaterials can improve the photon–crystal lattice interactions and the charge-transport mobility, leading to exquisite selectivity, sensitivity and short response/recovery time. Because of the great diversity of available organic molecules and the tunable electronical and chemical properties,20 organic nanowire-based sensors have inspired growing research efforts.To enhance the reactivity and decrease the detection limit in chemical detection, Zhao et al. have developed tris(2,2′-bipyridyl)ruthenium(II) (Ru(bpy)32+) nanowires (Fig. 1a) as reagents for electrogenerated chemiluminescence (ECL) sensors.18 These low dimensional nanostructures with a large aspect ratio can amplify the electro-active surface area of an electrode, which is helpful for improving the sensing property of the ECL sensor. The reduced graphene oxide (RGO)–Nafion composite films were subsequently used to immobilize the Ru(bpy)32+nanowires onto a glassy carbon electrode (GCE) and facilitate the charge transfer (Fig. 1b). In this case, the prepared ECL biosensor displays remarkable detection performance and can be used to detect various biological molecules. The effect of the dopamine (DA) concentration on the ECL intensity of the biosensor is shown in Fig. 1c. The ECL intensity was monitored as a function of the DA concentration ranging from 1.0 × 10−12 to 1.0 × 10−5 M, and the curve can be well fitted with the following equation:
(I − I0)/I0 = −0.71 − 0.04log CDA |
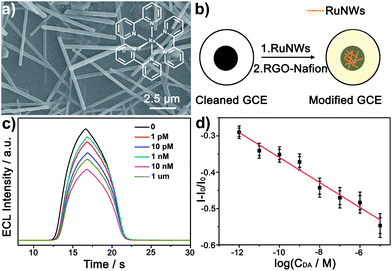 |
| Fig. 1 (a) SEM image of Ru(bpy)32+nanowires (RuNWs). Inset: Molecular structure of Ru(bpy)32+. (b) A schematic diagram showing the preparation process of the ECL biosensor. (c) Dependence of the ECL intensity of the RGO–Nafion/RuNWs/GCE on different DA concentrations. Scan rate: 100 mV s−1. (d) Linear calibration plot for DA determination. I0 and I are the ECL intensity of the RGO–Nafion/RuNWs/GCE without and with DA, respectively. The error bars represent the standard deviation of three measurements. Figures are adapted from ref. 18. | |
This typical calibration curve in Fig. 1d indicates the highly sensitive ECL response in a wide linear range with very low detection limit.
Fluorescence of 1D mesh-like organic nanofibers for sensing applications
In addition to the wide utilization of nanowire-based sensors, 1D nanofiber assemblies, as an alternative to discrete 1D nanostructures, have also been successfully used in sensor systems,21 due to high flexibility and the relatively simple preparation methods that allows large-scale production. Moreover, the entangled inherent property of nanofibers makes it easy to form mesh-like films that possess porosity on a number of length scales. Such a continuous and porous structure not only provides increased surface area for enhanced adsorption of gaseous molecules, but also enables expedient diffusion of guest molecules across the film matrix, leading to efficient sensing with both high sensitivity and fast time response.Asymmetric perylene tetracarboxylic diimide (PTCDI) molecules have been employed in a successful fabrication of millimeter long nanofibers in Zang's group. The long-range exciton migration intrinsic to the 1D well-organized molecular arrangement within the nanofiber enables amplified fluorescence quenching by surface adsorbed analytes. Taking advantages of such amplified fluorescence quenching, they synthesized a new type of molecule with bulky branched side chain (Fig. 2a inset), which may distort the π–π stacking to afford increased fluorescence for the molecular assembly. The well-defined nanofibers fabricated from the half-hydrolyzed PTCDI demonstrate strong fluorescence15 (with yield ca. 15%) as depicted in fluorescence microscopy images (Fig. 2a and b). The strong fluorescence of the nanofibers enables great sensitivity of a fluorescence sensor for detecting reductive volatile organic compounds, such as organic amines, through electron-transfer-based fluorescence quenching. Fig. 2c shows the fluorescence quenching efficiency (1 − I/I0) of a nanofibril film measured at three different vapor pressures of aniline. The quenching data are well fitted to the Langmuir equation with an assumption that the quenching efficiency is proportional to the surface adsorption (coverage) of amines. Upon exposure to saturated vapor of aniline (880 ppm), the fluorescence of the mesh-like nanofiber film was instantaneously quenched by almost 100% and the response time was only 0.32 s for the quenching process (Fig. 2d). The fast response obtained for the sensing is attributed to the three-dimensional (3D) continuous pores formed by the entangled piling of the nanofibers, which largely increases the mass transport rate of the analyte.
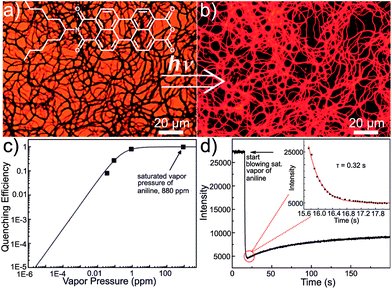 |
| Fig. 2 (a) Bright-field and (b) fluorescence optical microscopy image of a nanofibril film assembled from a half-hydrolyzed PTCDI. Inset: molecular structure. (c) Fluorescence quenching efficiency (1 − I/I0) as a function of the vapor pressure of aniline: data error (5%) fitted with the Langmuir equation. (d) Time-course of fluorescence quenching of a nanofibril film upon blowing over with saturated vapor of aniline (880 ppm), indicating a response time of about 0.32 s. Figures are adapted from ref. 15. | |
Real-time gas detection with vertically aligned organic nanowire arrays
As mentioned above, the surface areas of the sensing films and the analyte transport rate are critical to detection sensitivity.13b Growing research efforts have been made on the evolution of structures from netted 1D nanofibers to hierarchical 1D assemblies (3D supernanostructures/arrays).22 Most recently, engineered 3D nanowire arrays as the transduction elements have been explored for sensing applications with excellent performance.23 Even if there has been tremendous advancement in fabrication techniques of inorganic nanowire arrays with controllable size, orientation, growth site and density,24 much less has been done with organic materials, especially site-selective growth. Therefore, advances in capabilities of fabricating well-ordered and large-area organic arrays and integrating them with patterned substrates are of crucial importance and may lead to advanced functions in clinical trials, food safety, environmental, military and other areas.Huang and co-workers prepared vertical organic nanowire arrays from 1,5-diaminoanthraquinone (DAAQ) dye molecules with a facile physical vapor transport method (Fig. 3a).16 Protonating the N atoms in the DAAQ molecules enables the interruption of the intramolecular charge transfer, resulting in changes in the DAAQ fluorescence. Based on the color changes, acid sensing experiments can be carried out by exposing the nanowire arrays into the acidic vapor with very low concentrations. As shown in Fig. 3b, The PL intensity rapidly decreased upon exposure to HCl vapor (ca. 5 ppm) and reached over 90% quenching after only 30 s. Interestingly, the PL can be recovered in air to ∼95% of its original intensity in ∼2 h and can be rapidly reset by basic vapors (e.g., NH3) within 2 s. This also provides a mechanism for detecting basic vapors. The basic vapor deprotonated the amine groups and helped to restore the intramolecular charge transfer, leading to the recovery of color and fluorescence. During the vapor transport experiments, it is noted that the DAAQ tends to preferably nucleate and grow faster on high surface energy sites.25 This makes it possible to directly integrate the DAAQ nanowires onto a sensing device such as a portable fiber optics spectrometer (Fig. 3c) for real-time chemical detection. The fluorescence of the coated nanowires can be monitored for the detection of trace amounts of acidic vapors with very high sensitivity (Fig. 3d).
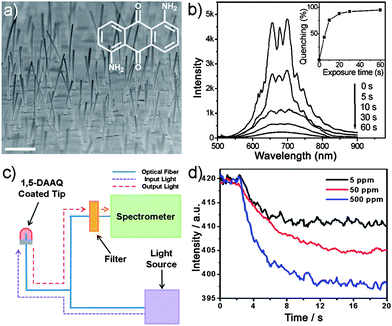 |
| Fig. 3 (a) SEM image of vertically aligned DAAQ nanowire arrays. Scale bar is 1 μm. Inset: molecular structure of DAAQ. (b) Time dependent PL spectra of the DAAQ nanowire arrays on exposure to HCl vapor of 5 ppm in air. (c) Schematic illustration of the setup for the sensing measurement. (d) The PL intensities of the nanowires monitored at 685 nm when exposed to HCl vapors of 5, 50 and 500 ppm, respectively. Figures are adapted from ref. 16 and 25. | |
Multicomponent organic 1D nanomaterials in sensing applications
It can be seen that single-component organic 1D nanostructures or assemblies have demonstrated electro-chemical and chemical sensing applications towards specific gases or analytes. In contrast, more tunable and reliable optical properties are expected in tailored binary systems.26 The binary systems can not only combine the properties of different compounds, but also generate new functionality based on intermolecular interactions and energy transfers.27 To prepare composite materials, the doping technique is mostly used because even small amounts of dopant can significantly change the charge mobility and enhance the opto-electronic performance.28 Besides, hetero-junction structures are very important in fabricating optical and electronic model devices.29 In this section, we emphasize on the recent developments in the field of 1D nanosensors based on composite materials.In binary systems, regulation of nonradiative energy transfer (e.g.fluorescence resonance energy transfer, FRET) provides an important route towards tuning the emission of nanomaterials.30 In this approach, even if a small amount of energy acceptor is doped into the donor matrix of energy donor materials, the energy could be efficiently transferred from the donor to the acceptor molecules and the emission color can be tuned to a large extent. Hence, the chemical sensors based on FRET might be promising for the integration of next-generation miniaturized optical sensor devices. To this end, Zhao et al. reported a supramolecular synthesis of nanobelts of 9,10-diphenylanthracene (DPA, a blue light emitting dye) doped with a very small amount of Nile red (NR, a red light emitting dye).31 Here the energy acceptor, NR, is sensitive to acid and basic chemical vapors, while the donor DPA molecule is not. Therefore, the fluorescence emission behavior of the synthesized binary nanobelts can act as an indication to identify such surrounding vapors, which leads to the opening (base) and closing (acid) of the energy transfer process in the composites, respectively.As displayed in Fig. 4a, when binary nanobelts with a DPA/NR molar ratio of 200
:
1 are exposed to HCl vapor (ca. 15 ppm in air) for 5 s, the red PL intensity of NR decreased by 25% while the blue PL intensity of DPA increased by 50%. The PL spectra evolved with an increase of exposure time to HCl vapor at room temperature, and became stable after exposing for 50 s due to the absorption equilibrium of HCl vapor. After removal from the HCl vapor, the PL of the nanobelt films can be slowly recovered by exposing them to the air for a few days, or can be quickly restored by exposing to NH3 vapor for tens of seconds (Fig. 4b). This operation can be repeated without any observable device fatigue, showing good sensor durability. Fig. 4c displays the change of the PL colors with fluorescence microscopy, through which the chemical vapor could be directly ‘read out’ by the naked eye, which makes it very convenient for the practical applications. This chemical sensing material composites based on the FRET mechanism provides a reference for the synthesis of other biochemical sensing complexes and will also find applications in the integrated and miniaturized optical sensing devices.
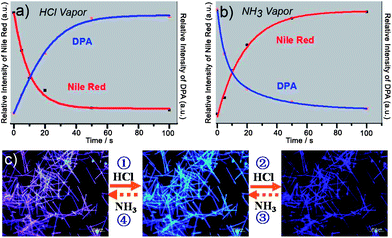 |
| Fig. 4 (a) PL intensity at 600 nm (red) and 440 nm (blue) with time when binary nanobelts are exposed to ∼15 ppm HCl vapor. (b) PL intensity at 600 nm (red) and 440 nm (blue) with time when the same sample was exposed to ∼50 ppm NH3 vapor. (c) Fluorescence microscopy images of the corresponding nanobelts on exposure to the chemical vapors. Figures are adapted from ref. 31. | |
Single cable-like composite nanowire for optical waveguide based gas sensing
Compared with the uniformly doped materials, heterostructures prepared by controllable anisotropic doping or epitaxial growth are expected to be more suitable for fabricating integrated optical and electronic devices.32 To date, there has been a move towards making sensors based on various heterostructures.33 Following the interest in the design of novel systems for the rapid and sensitive detection of chemical vapors, our group designed a core/sheath organic nanowire-based H2O2 sensor.34 In such a structure, single-crystalline 9,10-bis(phenylethynyl) anthracenenanowire was chosen as the core and H2O2-sensitive peroxalate ester as the shell. By utilizing the evanescence wave coupling, we realized an optical waveguide sensor with a single heterojunction wire, which exhibits both fast response and high selectivity for H2O2 vapor probing (Fig. 5a).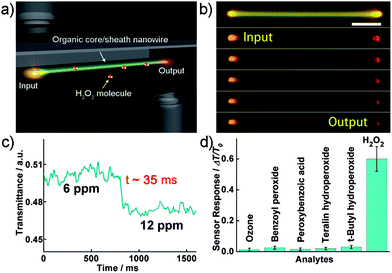 |
| Fig. 5 (a) Schematic for the measurement of the waveguide sensor. (b) A series of PL images of a single wire waveguide sensor in an atmosphere of H2O2 vapor (6 ppm). Scale bar is 5 μm. Here 351 nm light (∼100 pW) is guided from left tip to right. (c) Typical time-dependent transmittance of the sensor reveals the response time of about 35 ms when H2O2 vapor concentration is raised from 6 to 12 ppm. (d) Selectivity analysis for H2O2 vapor detection by monitoring the relative change of optical transmission. Figures are adapted from ref. 34. | |
As depicted in Fig. 5b, once the gas mixture containing 6 ppm H2O2 was injected into the sensing region, the output of the waveguide was drastically attenuated (ca. 50%) with the input luminous flux remaining unchanged. This monotonic decrease of the PL output with H2O2 exposure can be used for H2O2 sensing. The instant response of the sensor was investigated by introducing sudden changes of the H2O2 vapor concentration in the chamber, with typical time-dependent transmittance shown in Fig. 5c. The estimated response time of the sensor is about 35 ms when H2O2 vapor concentration jumps from 6 to 12 ppm. The remarkably fast response of the sensor can be attributed to the small diameter and large surface-to-volume ratio of the nanocable that enable rapid diffusion and capture of the H2O2 molecules, as well as fast signal retrieval using the optical approach. It should be noted that the core/sheath nanoprobe can distinguish H2O2 from ozone and other organic volatile peroxides, as is clearly shown in Fig. 5d. As is known, nanowire-based field-effect sensors exhibit limited sensitivity, because the conductive channel in the device provides little free volume for the mass transport of an analyte. This cable-like optical waveguide sensing platform complements the present nanowire field-effect sensors with the ability to rapidly and selectively monitor the amplified optical variation across the wire waveguiding, showing that a single nanocable is very promising for developing optical probes to detect individual responses on a chip.
Conclusion
In summary, 1D organic nanomaterials with various structures and compositions provide a promising platform that combines tailored sensitivity and chemical selectivity for highly efficient chemical sensing applications. This review has reviewed recent advances in 1D sensors based on small organic molecules including their fabrication, assembly, functionalization and sensing performances. We can see all these features and applications strongly depend on the crystal morphology and the molecular composition. The nanostructure morphology shows great impact on the optical properties as well as the diffusion and detection of the analyte. New opportunities therefore exist for better understanding the effects of size, shape and dimensionality on the optical and electrical properties of sensing materials. Moreover, the composite nanomaterials could combine intermolecular energy transfer with the shape-dependent optical properties, making them more functional and suitable for integration. From these considerations, the design and synthesis of novel organic crystal structures and multicomponent assemblies are essential to the building up of future sensing elements.However, there is still a long way to fully exploit the sensing applications of these functional nanomaterials, because the fabrication and integration of 1D nanomaterials into practical sensing devices are still in their infancy. Many efforts should also be made to find practical routes for fabricating 1D nanostructures from a diversified and wide range of raw materials, rapidly and at reasonable cost. More importantly, a thorough understanding of the growth mechanism is of particular significance both for the hierarchical assembly and alignment of 1D nanostructures as well as for the fabrication of sensing devices using these structures. For example, assembling larger and more complex nanowire sensor arrays and integrating them with nanoscale electronics for processing will overcome the shortcomings of single nanowire field-effect sensors and lead to exquisite sensor system performances. In spite of the numerous challenges, 1D organic materials offer unlimited research opportunities for the development of next-generation sensor devices.
Acknowledgements
This work was supported by the National Natural Science Foundation of China (nos 21125315, 91022022, 51203165), the Chinese Academy of Sciences, and the Ministry of Science and Technology of China (2012YQ120060 and National Basic Research 973 Program).Notes and references
-
(a) D. J. Sirbuly, M. Law, P. Pauzauskie, H. Q. Yan, A. V. Maslov, K. Knutsen, C. Z. Ning, R. J. Saykally and P. D. Yang, Proc. Natl. Acad. Sci. U. S. A., 2005, 102, 7800 CrossRef CAS PubMed;
(b) J. X. Huang, S. Virji, B. H. Weiller and R. B. Kaner, J. Am. Chem. Soc., 2003, 125, 314 CrossRef CAS PubMed;
(c) E. Comini, G. Faglia, G. Sberveglieri, Z. W. Pan and Z. L. Wang, Appl. Phys. Lett., 2002, 81, 1869 CrossRef CAS;
(d) J. Kong, N. R. Franklin, C. Zhou, M. G. Chapline, S. Peng, K. Cho and H. Dai, Science, 2000, 287, 622 CrossRef CAS.
- M. C. McAlpine, H. Ahmad, D. W. Wang and J. R. Heath, Nat. Mater., 2007, 6, 379 CrossRef CAS PubMed.
- N. S. Ramgir, Y. Yang and M. Zacharias, Small, 2010, 6, 1705 CrossRef CAS PubMed.
-
(a) M. Law, D. J. Sirbuly, J. C. Johnson, J. Goldberger, R. J. Saykally and P. Yang, Science, 2004, 305, 1269 CrossRef CAS PubMed;
(b) L. Tong, J. Lou, R. R. Gattass, S. He, X. Chen, Liu and E. Mazur, Nano Lett., 2005, 5, 259 CrossRef CAS PubMed.
-
(a) Y. S. Zhao, H. Fu, A. Peng, Y. Ma, D. Xiao and J. Yao, Adv. Mater., 2008, 20, 2859 CrossRef CAS;
(b) S.-J. Lim, B.-K. An, S. D. Jung, M.-A. Chung and S. Y. Park, Angew. Chem., Int. Ed., 2004, 43, 6346 CrossRef CAS PubMed.
-
(a) L. Zang, Y. Che and J. S. Moore, Acc. Chem. Res., 2008, 41, 1596 CrossRef CAS PubMed;
(b) A. D. Peng, D. B. Xiao, Y. Ma, W. S. Yang and J. N. Yao, Adv. Mater., 2005, 17, 2070 CrossRef CAS.
-
(a) J. Luo, Z. Xie, J. W. Y. Lam, L. Cheng, H. Chen, C. Qiu, H. S. Kwok, X. Zhan, Y. Liu, D. Zhu and B. Z. Tang, Chem. Commun., 2001, 1740 RSC;
(b) Y. S. Zhao, H. Fu, A. Peng, Y. Ma, Q. Liao and J. Yao, Acc. Chem. Res., 2010, 43, 409 CrossRef CAS PubMed;
(c) Y. S. Zhao, A. Peng, H. Fu, Y. Ma and J. Yao, Adv. Mater., 2008, 20, 1661 CrossRef CAS.
- F. X. Gu, L. Zhang, X. F. Yin and L. M. Tong, Nano Lett., 2008, 8, 2757 CrossRef CAS PubMed.
-
(a) C. Zhang, Y. S. Zhao and J. Yao, Phys. Chem. Chem. Phys., 2011, 13, 9060 RSC;
(b) Q. H. Cui, Y. S. Zhao and J. Yao, J. Mater. Chem., 2012, 22, 4136 RSC;
(c) J. Xiao, H. Yang, Z. Yin, J. Guo, F. Boey, H. Zhang and Q. Zhang, J. Mater. Chem., 2011, 21, 1423 RSC.
- A. K. Wanekaya, W. Chen, N. V. Myung and A. Mulchandani, Electroanalysis, 2006, 18, 533 CrossRef CAS.
- Y. Huang, B. Quan, Z. Wei, G. Liu and L. Sun, J. Phys. Chem. C, 2009, 113, 3929 CAS.
- D. Gust, T. A. Moore and A. L. Moore, Acc. Chem. Res., 1993, 26, 198 CrossRef CAS.
-
(a) Y. Che, X. Yang, G. Liu, C. Yu, H. Ji, J. Zuo, J. Zhao and L. Zang, J. Am. Chem. Soc., 2010, 132, 5743 CrossRef CAS PubMed;
(b) L. Li, P. Gao, M. Baumgarten, K. Müllen, N. Lu, H. Fuchs and L. Chi, Adv. Mater., 2013, 25, 3419 CrossRef CAS PubMed;
(c) M. Schlupp, T. Weil, A. J. Berresheim, U. M. Wiesler, J. Bargon and K. Müllen, Angew. Chem., Int. Ed., 2001, 40, 4011 CrossRef CAS.
- T. Naddo, Y. Che, W. Zhang, K. Balakrishnan, X. Yang, M. Yen, J. Zhao, J. S. Moore and L. Zang, J. Am. Chem. Soc., 2007, 129, 6978 CrossRef CAS PubMed.
- Y. Che, X. Yang, S. Loser and L. Zang, Nano Lett., 2008, 8, 2219 CrossRef CAS PubMed.
- Y. S. Zhao, J. Wu and J. Huang, J. Am. Chem. Soc., 2009, 131, 3158 CrossRef CAS PubMed.
- Z. Tian, Y. Chen, W. Yang, J. Yao, L. Zhu and Z. Shuai, Angew. Chem., Int. Ed., 2004, 43, 4060 CrossRef CAS PubMed.
- Q. Li, J. Y. Zheng, Y. Yan, Y. S. Zhao and J. Yao, Adv. Mater., 2012, 24, 4745 CrossRef CAS PubMed.
-
(a) F. S. Kim, G. Ren and S. A. Jenekhe, Chem. Mater., 2011, 23, 682 CrossRef CAS;
(b) G. Zhao, H. Dong, L. Jiang, H. Zhao, X. Qin and W. Hu, Appl. Phys. Lett., 2012, 101, 103302 CrossRef.
-
(a) X. Zhang, J. Jie, W. Zhang, C. Zhang, L. Luo, Z. He, X. Zhang, W. Zhang, C. Lee and S. Lee, Adv. Mater., 2008, 20, 2427 CrossRef CAS;
(b) H. Bi, H. Zhang, Y. Zhang, H. Gao, Z. Su and Y. Wang, Adv. Mater., 2010, 22, 1631 CrossRef CAS PubMed.
- C. Zhang, Y. Che, X. Yang, B. R. Bunes and L. Zang, Chem. Commun., 2010, 46, 5560 RSC.
- L. Wang, Y. Zhou, J. Yan, J. Wang, J. Pei and Y. Cao, Langmuir, 2009, 25, 1306 CrossRef CAS PubMed.
- Y. Cui, Q. Wei, H. Park and C. M. Lieber, Science, 2001, 293, 1289 CrossRef CAS PubMed.
-
(a) I. Lombardi, A. I. Hochbaum, P. Yang, C. Carraro and R. Maboudian, Chem. Mater., 2006, 18, 988 CrossRef CAS;
(b) A. I. Hochbaum, R. Fan, R. He and P. Yang, Nano Lett., 2005, 5, 457 CrossRef CAS PubMed;
(c) H. J. Fan, P. Werner and M. Zacharias, Small, 2006, 2, 700 CrossRef CAS PubMed.
- Y. S. Zhao, P. Zhan, J. Kim, C. Sun and J. Huang, ACS Nano, 2010, 4, 1630 CrossRef CAS PubMed.
- J. Y. Zheng, Y. Yan, X. Wang, Y. S. Zhao, J. Huang and J. Yao, J. Am. Chem. Soc., 2012, 134, 2880 CrossRef CAS PubMed.
- C. Zhang, Y. S. Zhao and J. Yao, New J. Chem., 2011, 35, 973 RSC.
-
(a) C. W. Tang, S. A. Vanslyke and C. H. Chen, J. Appl. Phys., 1989, 65, 3610 CrossRef CAS;
(b) V. Shrotriya, Y. Yao, G. Li and Y. Yang, Appl. Phys. Lett., 2006, 89, 063505 CrossRef.
- Y. Wu, J. Xiang, C. Yang, W. Lu and C. M. Lieber, Nature, 2004, 430, 704 CrossRef CAS.
-
(a) Y. Ner, J. G. Grote, J. A. Stuart and G. A. Sotzing, Angew. Chem., Int. Ed., 2009, 48, 5134 CrossRef CAS PubMed;
(b) F. He, Y. Tang, S. Wang, Y. Li and D. Zhu, J. Am. Chem. Soc., 2005, 127, 12343 CrossRef CAS PubMed;
(c) T.-H. Kim and T. M. Swager, Angew. Chem., Int. Ed., 2003, 42, 4803 CrossRef CAS PubMed.
- J. Y. Zheng, C. Zhang, Y. S. Zhao and J. Yao, Phys. Chem. Chem. Phys., 2010, 12, 12935 RSC.
-
(a) M.-Y. Lu, J. Song, M.-P. Lu, C.-Y. Lee, L.-J. Chen and Z. L. Wang, ACS Nano, 2009, 3, 357 CrossRef CAS PubMed;
(b) R. X. Yan, D. Gargas and P. D. Yang, Nat. Photonics, 2009, 3, 569 CrossRef CAS;
(c) J. H. Kim, A. Watanabe, J. W. Chung, Y. Jung, B.-K. An, H. Tada and S. Y. Park, J. Mater. Chem., 2010, 20, 1062 RSC.
-
(a) X. Wang and C. S. Ozkan, Nano Lett., 2008, 8, 398 CrossRef CAS PubMed;
(b) S. K. Jha, C. P. Liu, Z. H. Chen, K. J. Chen, I. Bello, J. A. Zapien, W. Zhang and S.-T. Lee, J. Phys. Chem. C, 2010, 114, 7999 CrossRef CAS.
- J. Y. Zheng, Y. Yan, X. Wang, W. Shi, H. Ma, Y. S. Zhao and J. Yao, Adv. Mater., 2012, 24, OP194 CrossRef CAS PubMed.
|
This journal is © The Royal Society of Chemistry 2014 |
Click here to see how this site uses Cookies. View our privacy policy here.