Prospective pedagogy for teaching chemical bonding for smart and sustainable learning
Received
18th March 2014
, Accepted 13th June 2014
First published on 16th June 2014
Abstract
As an important subject in the curriculum, many students find chemistry concepts difficult to learn and understand. Chemical bonding especially is important in understanding the compositions of chemical compounds and related concepts and research has shown that students struggle with this concept. In this theoretical paper based on analysis of relevant science education research, textbooks, and our classroom observations and teaching experiences, the authors argue that the difficulty in learning chemical bonding concepts is associated with the sequence (ionic, covalent and polar covalent bonding) in which students are taught because this sequence receives little support from constructivist theories of learning. Consequently, the paper proposes a sequence to teach chemical bonding (covalent, polar covalent and ionic bonding) for effective and sustainable learning. In this sequence, the concepts are developed with minimum reorganisation of previously learned information, using a format which is claimed to be easy for students to learn. For teaching these concepts, the use of electronegativity and the overlap of atomic orbitals for all types of bonding have also been stressed. The proposed sequence and emphasis on electronegativity and atomic orbital overlap meets the criteria for teaching and learning of concepts based on the psychology of learning including the theory of constructivism necessitating the construction of new knowledge using related prior knowledge. It also provides a better linkage between the bonding concepts learned at secondary and tertiary levels. Considering these proposed advantages for teaching, this sequence is recommended for further research into effective and sustainable teaching.
Introduction
Researchers are deeply concerned about secondary and tertiary students' (a) perceptions of chemistry being difficult, (b) experiencing difficulty in learning the subject, (c) inadequate background content knowledge for learning advanced content, and (d) ability to apply their knowledge to real world problems (Nakhleh, 1992; Boujaoude and Barakat, 2000; Coll and Treagust, 2001, 2002; Dhindsa, 2002; Dhindsa and Treagust, 2009). These authors have also stated that the large amount of content taught in a grade level, content abstractness, the traditional teaching style with emphasis on rote learning and assessment through tests and examinations are among the major factors responsible for students' poor perceptions of chemistry. For example, students' incomplete and vague answers to chemistry examination questions reflect their shallow understanding of the concepts (Dhindsa and Treagust, 2009).
To overcome such problems associated with chemistry teaching and learning, researchers have proposed improvements to the chemistry curriculum including attending to course content sequencing, staff/student relationship, students' social and cultural background, learning styles, use of technology and alternative explanations (Bodner, 1992; Dhindsa, 2000; Kirkwood and Symington, 1996; Gabel, 1999; Dhindsa and Emran, 2006; Nahum et al., 2007; Taber, 2011; Abiola and Dhindsa, 2012). This report concentrates on chemical bonding content sequencing and proposes better explanations for effective teaching and learning.
Curriculum developers (experts) sequence the chemistry content knowledge that is to be taught with increasing difficulty to match the mental development of the students as their age increases. They also aim to support higher levels of content learning using previously learned content as prior knowledge. The selection of content and its organization/sequencing is based on the expertise of curriculum developers. When they are not aware of recent related science education research to guide the selection and sequencing the content, they rely heavily on the way they understand the content that is usually organised from the unknown to the known. Often this sequencing fails to satisfy the learning needs of students especially those needs described by psychological theories of learning (Abiola and Dhindsa, 2012) and hence encourages rote learning. Effective teaching requires sequencing content from the known to the unknown to support student learning (OPSU, 2010). On the other hand, metallurgy topics (say, about iron), for example, are arranged and taught from the unknown to the known (ores, extraction, properties and uses). This sequence is an expert organization, the way experts understand the content, and is not based on the students' daily experiences with iron.
Psychologically, students learn from the known to the unknown (Dunlosky et al., 2013). Therefore, for effective teaching, the organization of the topic about iron, for example, the sequence uses, properties, ores and extraction of iron which makes sense. There has been a belief that once a student learns higher-level chemistry content, the lower level chemistry content becomes clear to him/her. This approach has encouraged teachers to oversimplify the concepts for teaching students at lower levels of schooling. However, it has been found that the oversimplified concepts taught at lower level are misconceptions which resist change even at higher levels (Taber, 2000, 2011, 2012; Coll and Treagust, 2002; Wimmer and Dhindsa, 2005; Dhindsa, 2011). Dhindsa (2011) has reported that graduate students defined an element as a substance with the same type of atoms, an oversimplified definition learned at lower secondary level. Oversimplification of concepts rather adds to students' misconceptions and frustrations (Hurst, 2002; Justi and Gilbert, 2002; Dhindsa, 2011). Moreover, teachers unthinkingly follow textbook sequences and explanations which are purported to also be supported by learning theories. However these sequences are largely how the topics have been introduced over time. These sequences follow the unknown to the known pattern and the explanations often are incomplete (Abiola and Dhindsa, 2012). According to these authors, teachers use the marbles and sand analogy to explain a decrease in volume when equal volumes of ethanol and water are mixed. Based on this explanation, the volume should always decrease during liquid–liquid solution formation because often molecules are not spherical in shape and some part of the molecules can go into the spaces between molecules; however, in other cases the volume may increase or stay the same. Moreover, this explanation ignores the interaction between solvent and solute particles. Such incomplete explanations are not sustainable and require students to learn, unlearn and relearn the process over and again. One approach to address these and other aspects of learning chemical bonding has been to present a coherent view of energy and energetics using an interdisciplinary approach (Cooper and Klymkowsky, 2013).
The authors of this study argue that chemical bonding is another topic that is not sequenced and taught to support effective and sustainable learning at all levels. Moreover, explanations are incomplete and oversimplified. This deficiency is reflected in the numerous problems (including acquiring misconceptions) that students face when learning this topic (Wimmer et al., 2006; Nahum et al., 2007; Ozmen, 2004; Taber, 2011). These researchers also have expressed their dissatisfaction with the way in which chemical bonding is taught; however, there are only a few researchers who have proposed new teaching pedagogies and models to overcome these problems. Taber (2001) considered a progression from electron and metal cation interaction in metals to cation and anion interaction in ionic compounds and proposed that metallic bonding should be taught before ionic bonding. Thereafter covalent bonding in giant covalent structures followed by covalent bonding in simple molecules should be taught. We argue that concept of multiple bonding of an atom with a number of other atoms is a difficult concept for students to understand. Also, as discussed later in the paper, progression from ionic to covalent bonding might be difficult for students to understand. More recently, Taber (2011) proposed an alternate explanation for teaching chemical bonding based on electrostatic attraction; in all bonds, the length of the bond depends on the magnitude of attractive and repulsive forces between the bonding atoms. Nahum et al. (2010) summarized research on chemical bonding and covered various aspects of it including the way it is taught, misconceptions, bonding models and other factors. They also described recent research on this topic by Nahum et al. (2007, 2008). Nahum et al. (2008) proposed the use of Coulomb's law in explaining chemical bonding and further stated that (a) the chemical bonding section should be sequenced based on bond strength for teaching and (b) there are no pure ionic or covalent bonds; all bonds have ionic and covalent bonding components (based on the valance bond theory).
However, chemical bonds are still taught as pure ionic and covalent; as well, the role of electronegativity in bonding is often not made clear in ionic bonding. There are four limitations in this teaching approach: (a) the bonding concepts are arranged on the basis of bond strength – a concept that students find difficult to understand, (b) the proposed teaching order is still the traditional ionic, covalent, polar covalent (partly covalent and partly ionic), hydrogen bonds and van der Waals bonds (strong to weak), (c) if the proposed teaching order is low strength to high strength (as commonly understood by students) then the proposed sequence (ionic to van der Waals) of concepts for teaching and expected bond strength (weak to strong) is not in line with each other, and (d) the concept of electronegativity is introduced when polar covalent bonds are introduced. Moreover there are no explanations given to support how an electron pair will move between A+B−, AB and A−B+ states. It also lacks visual representation of bonding concepts. The literature is silent about the requirement of overlap of atomic orbitals to occur for electrons to transfer in all types of bonding. Also a continuous spectrum for bonding from covalent to ionic is not demonstrated visually. In summary, the problems associated with difficulty, misconceptions and lack of understanding of the chemical bonding topic might be associated partly with the way the subtopics of chemical bonding are sequenced and taught to the students. Therefore, in this article the chemical bonding topic has been selected to evaluate its sequence of teaching. According to Watson and Mason (2006), researchers, curriculum developers and teachers can assist students to make connections between prior knowledge and new content by using carefully sequenced examples, including examples of students' own solution strategies, to illustrate key ideas.
Valence bond theory
Valence bond theory (VBT) is based on the Lewis structure of molecules and assumes that the electrons in a molecule occupy atomic orbitals of individual atoms. Therefore, in VBT, the picture of an individual atom during bond formation is retained. The covalent bond is formed by an overlap of atomic orbitals of bonding atoms (e.g. two 1s orbitals of two H atoms overlap to form a H2 molecule). The overlap means two orbitals share a common region in space. There is a high probability for the shared electron pair(s) to be found in the common region but the bonding pair may leave this region. VTB suggests that a valance bond is a linear combination of covalent and ionic states.
ΨVB = Ψ(A − B) + aΨ(A−B+) + bΨ(A+B−) |
However, contributions from ionic and covalent states to form the valence bond may be different. Furthermore, the contribution of each of two ionic states (see a & b in the above equation) is also different and depends on the nature of the atoms involved in bonding. Hence, every valence bond involves some component of a covalent bond that requires overlap of atomic orbitals. Therefore the concept of orbital overlap as suggested by VBT also can be applied to ionic and metallic lattices.
Chemical bonding: teaching sequence and explanations
The analysis of secondary and tertiary (First year) textbooks (Chang, 1988; Zumdahl, 1998; Brown et al., 2009; Hill et al., 2010; Timberlake 2010) as well as our O- and A-level classroom observations in Brunei and teaching experiences in Australia have revealed that the chemical bonding topic is taught in the order of ionic, covalent, non-polar covalent, metallic and inter-molecular bonding. Tsaparlis and Pappa (2011) reported that 10 out of 14 textbooks followed the above order. Only a few authors have considered a sequence different from the above for teaching these concepts (Johnstone et al., 1981; Tsaparlis, 2000; Tsaparlis and Pappa, 2011). A recent analysis of upper secondary level textbooks in Sweden demonstrated that the way models of chemical bonding are presented might cause students to have alternative conceptions and difficulties in understanding chemical bonding (Bergqvist et al., 2013).
Ionic bonding is described as an electrostatic force of attraction between ions of opposite charges. Ions are formed from atoms by the transfer of one or more electrons from one atom to another. The maximum number of electrons transferred can be three thus giving rise to +3 and −3 charge on the cations and anions, respectively. Some anions consisting of more than one atom [e.g. in NaCN, (C–N)−] do have covalent bonds. These covalent bonds are not broken when ionic solids are melted or dissolved. The ionic bond exists between atoms of metals (from groups 1, 2 and 3 in the periodic table) and non-metals (from groups 6 and 7). The octet rule (noble gas configuration) is used to explain the stability of the electronic configuration of ionic states of atoms involved in ionic bonds. All these books gave an example of ionic bonding in sodium chloride formation. The books have also shown graphically the transfer of an electron (jumping electron) from sodium to chlorine using Lewis electron-dot symbols.
Covalent bonding is described as sharing of electron pairs between two atoms of the same or different elements to complete the octet of each atom to attain the stable noble gas configuration. The books use molecules of hydrogen, chlorine and/or fluorine as examples of diatomic covalent bonding and Lewis electron-dot symbols are used to show the sharing of electrons graphically. Often no example is given that involves the sharing of electrons between two atoms of different elements. These examples assume/reflect that bond formation occurs when an equal contribution of electrons from both bonding atoms occurs that leads to equal sharing of bonding pair(s) of electrons. This bond is rarely called a non-polar covalent bond in the textbooks.
Polar covalent bonding is often taught as a separate entity under unequal sharing of bonding electron pairs. It is taught as a covalent bond that involves unequal sharing of bonding pair or pairs of electrons that are equally contributed by the bonding atoms. The basic idea of stability through attaining noble gas configuration following the octet rule is stressed. Electronegativity values of bonding atoms are used to justify that the bonding pair is shared more by one of the bonding atoms than the other. An atom with a higher electronegativity value will share the bonding electron pair more than the one with lower value. As a result, one electron is partially lost by one atom and gained by the other, thus giving rise to partial negative and positive charges on the bonding atoms. This bond is classified as a polar covalent bond. The partial charge on bonding atoms is represented as delta positive (δ+) and delta negative (δ−). The atom with a higher electronegativity value receives δ− and the other receives δ+. The magnitude of the charge depends upon the difference in electronegativity values of the bonding pair of atoms. A coordinate covalent/dative bond is often not discussed in the textbooks.
Metallic bonding is described as bonding due to valence electrons that are delocalised throughout the solid. The metallic bond is described as an array of metal positive ions immersed in a sea of delocalised valence electrons. A cross section of a metal is shown representing equal amounts of negative charge for electrons and positive charge for metallic kernels; the overall charge is zero. The strength of the metallic bond is associated with the number of delocalised valence electrons, e.g., one for sodium (example of a soft metal) and six for chromium (example of a hard metal). This is an old model that is not in line with recent developments in the area of metallic bonding. More details are discussed in a later part of this article.
Analysis of teaching sequence and explanations
The major problems associated with this order of teaching are that the students learn that all types of bonding are independent of each other and there is no association between them. They also learn that electronegativity can explain polar covalent bonding and it does not have a role in ionic bonding. The students gain the notion that during ionic bonding formation electrons can jump from one atom to another under normal conditions, which is not an acceptable conception.
When covalent bonding is taught after ionic bonding, teachers often fail to establish the link between ionic and covalent bonding because these are extreme ends of the bonding spectrum based on differences in the electronegativity values of the bonding atoms. Hence the actual link between ionic and covalent bonding is relatively weak because these ideas are still to be connected by teaching polar covalent bonding. The students, therefore, might develop the notion that covalent bonding is also an independent identity and has nothing to do with ionic bonding. Teaching polar covalent bonding after covalent bonding follows an acceptable order because the concepts are well connected and teachers can establish a link between them using valence bond theory and Lewis dot symbols. The students can also understand the link. However, analysis of textbooks and our school teaching experiences revealed that textbooks and teachers spend very little or no time to show the students that polar covalent bonding is a link that connects ionic and covalent bonding; for the teacher who would like to establish such a link, the teaching order is reversed. This means that after teaching polar covalent bonding, teachers have to go back to ionic bonding that was taught before the covalent bonding. When metallic bonding is taught after polar covalent, teachers often fail to establish a link between polar covalent bonding and metallic bonding and so metallic bonding is taught as an isolated concept.
Teachers and textbooks establish polarity of the polar covalent bond using differences in electronegativity of the bonding atoms, whereas they place very little emphasis on electronegativity data while teaching ionic and covalent bonding. The students therefore often may develop the notion that electronegativity has no role to play in covalent and ionic bonding, despite electronegativity values of bonding atoms playing a significant role in all types of bonding. Covalent bonding occurs between atoms of the same (or slightly different) electronegativity values and ionic bonding between atoms with extremely large differences in electronegativity values. Scientists have agreed to these differences as ≤0.4 for covalent bonding and >1.7 for ionic bonding (the electronegativity values for all atoms on the Linus Pauling scale range from 0.7 to 4.0 (Chang, 1988, p. 318)). Between these values lies the polar covalent bond. These boundaries are fuzzy. The students learn that the electronegativity difference can change the position of the bonding electron pair from the middle to one side. Moreover, the number of examples given in the textbooks and used during classroom teaching is limited to support students' learning of these concepts.
Teachers often use NaCl as an example to teach ionic bonding and use a visual model to demonstrate that an electron jumps from sodium to chlorine without realizing that an ionization energy of 496 kJ mol−1 is required for an electron to leave the outermost orbit of the sodium atom. This is an oversimplified explanation of ionization to establish an ionic bond and it leads to the misconception that an electron from a sodium atom jumps to a chlorine atom. The authors argue that the electron affinity of 348 kJ mol−1 for chlorine cannot cause sodium to lose its outermost electron. A similar argument is reported by Taber et al. (2012). Hence, the transfer of outermost electron from sodium to chlorine will require some mechanism.
The major problems with the way chemical bonding is taught include:
(1) The sequence of ionic, non-polar covalent and polar covalent bonding concepts for teaching is not appropriate to support effective and sustainable learning.
(2) These concepts are not properly connected to show a continuity (or spectrum) of chemical bonding.
(3) Electronegativity data are mainly used to describe the polar covalent bond not the other types of bonds.
(4) No mechanism is described to support the electron transfer from an atom to another in ionic bonding as well as for the formation of A+B− and A−B+ in all types of bonding. Electrons simply do not jump between atoms.
Proposed teaching order
Consistent with the calls for learning progressions being a guide for developing meaningful learning in science (Stevens et al., 2013), the authors believe that chemical bonding should be taught in three stages: (1) covalent, polar covalent and ionic bonding, (2) bonding in lattices: metallic and crystals, and (3) inter- and intra-molecular bonding. This paper concentrates on the first and second stages. During the first stage, covalent bonding should be taught first, followed by polar covalent and then ionic bonding. Hereafter bonding in metals and ionic lattices should be taught. Since this sequence is based on electronegativity values, the role of electronegativity in chemical bond formation becomes evident. Moreover, covalent bonding is explained using an overlap of atomic orbitals; teachers can stress the concept of overlap in each type of bonding as they move from covalent to ionic bonding. It would explain the formation of A+B−, AB and A−B+ states. Hence this sequence will address the above stated problems. The advantages of using this sequence for teaching are discussed in detail in the following section.
Advantages: proposed teaching order and overcoming the stated problems
Learning sequence and constructivism
This order represents a continuum (spectrum) of the bonding from equal sharing of bonding electron pair(s) in covalent bonding to the extreme case of unequal sharing of bonding electron, that is, very little sharing of the electron with the parent atom in ionic bonding. A visual representation of teaching in this order together with some characteristics is reported in Fig. 1. Covalent bonding results from the attraction of the negatively charged electrons to the positively charged nuclei of the atoms of both elements. The resulting equal sharing of bonding electrons by the bonding atoms is due to the electronegativity values of the two atoms being either equal or nearly equal. Teachers should emphasise that sharing of an electron pair increases the net attraction force between the bonding atoms. As the electronegativity difference between bonding atoms increases, the extent of equal sharing of the bonding electron pair decreases as shown by a shift in the position of the electron pair from a central location between the bonding atoms (see Fig. 1; readers should consider both vertical and horizontal flow of information in the figure). A shift in the position of a bonding electron pair from the middle of covalently bonded atoms results in partial charge (δ+, δ−) on the bonding atoms with δ+ on the atom that shares less of the bonding electron pair and δ− on the atom that shares more (example, Hδ+: Nδ−). It further leads to a case when the bonding electron pair is mostly shared by one of the bonding atoms and the other gets very little opportunity to share the pair, resulting in ionic bonding. This approach does fit into our social context when we often share things equally or unequally. For example, when we go for a lunch in a group say of two, each member often contributes equally. Sometimes a member contributes more than other and there are cases when a member invites others and pays the overall bill. Teachers can use this daily life social experience to teach these concepts.
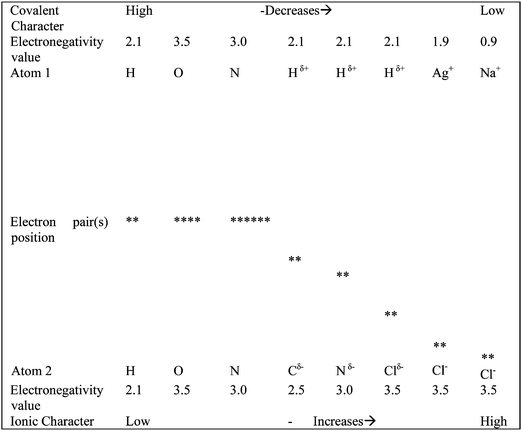 |
| Fig. 1 Continuum of bonding with bonding electrons in expected high probability areas. | |
In this order, the concepts are well connected and build sequentially on each other as supported by the notion of constructivism. Teachers can build their teaching of polar covalent bonding on non-polar covalent bonding and also along similar lines for ionic bonding on polar covalent bonding. They can ask students to compare some characteristics of the three types of bonds such as the nature of bonding atoms (atoms of same or different elements), extent of overlap of orbitals for electron sharing as well as for electron transfer, electronegativity of bonding atoms, the role of electronegativity in three types of bonding, and changes in covalent and ionic characters from covalent to ionic bonding (See Table 1). Teachers can use these characteristics to introduce lattice (ionic and metallic) bonding. This sequence of concepts will allow the students to construct their learning using previously learned bonding concepts as prior knowledge, hence the learning process is supported by the constructivist theory of learning.
Table 1 Some characteristics of chemical bonds
Bond |
Atoms type |
Electron sharing |
Electronegativity difference |
Orbital overlap |
Covalent character |
Ionic character |
Note: ? represents questions for students to decide which of the above characteristic fits most for metallic bonding. |
Covalent |
Same & different |
High probability |
None or small |
Large |
Maximum |
Minimum |
Polar covalent |
Different |
Intermediate probability |
Small to large |
Large to small |
Less than covalent bonding |
More than covalent bonding |
Ionic bonding |
Different |
Low probability |
Large |
Small |
Minimum |
Maximum |
Metallic |
? |
? |
? |
? |
? |
? |
To introduce metallic bonding, teachers can ask the students about the type of bonding they expect between atoms in a metallic lattice. The teacher may guide the students to consider the characteristics of chemical bonds in Table 1 before introducing metallic bonding. A consideration of these characteristics may guide the students to think of metallic bonding as a type of covalent bonding. However, to differentiate between metallic and covalent bonds, we recommend that teachers compare the differences between these bonds in addition to the similarities. For example, a metallic atom can form multiple bonds with other atoms of its kind by overlap between spherical s-orbitals of atoms. The concept of formation of multiple bonds in metallic bonding is distinct from other type of bonds. This approach can help us support metallic bonding using VBT. The electron sea and metal kernel model does not clearly explain the concept of overlap of atomic orbitals required for the movement of electrons from one metallic atom to another. However, VBT is inclusive of explanations made by an electron sea and metal kernel model. Teachers can adopt a similar approach to teach bonding in ionic lattices.
Emphasis on electronegativity
The covalent bond (non-polar) is an easy concept to teach by using simple examples of diatomic molecules such as hydrogen, oxygen and nitrogen molecules. The use of these examples of non-polar covalent bonding will help the students learn single, double and triple bonds. The Lewis dot notation or overlap of atomic orbitals can be used for visual representation of covalent bonding. Also, the role of electronegativity in bonding can be emphasized. Teachers can then use this information to teach the concept of polar covalent bonding. They can emphasise that an increase in electronegativity difference between bonding atoms, reduces the extent of overlap as more electron density is transferred to a more electronegative atom. As a result, the shared pair of electrons spends more time with the more electronegative atom. Teachers can further link teaching of ionic bonding to polar covalent bonding, with the former being a case of maximum polarity. In this sequence of teaching, teachers are required to use electronegativity data as a basis for classifying these types of bonding. Therefore, they can use the electronegativity data to explain the probability of finding electrons between the bonding atoms.
Ionic bonding and atomic overlap
In the proposed sequence of learning these concepts, students learn the importance of overlap of atomic orbitals. They will also learn that with an increase in difference of electronegativity values of bonding atoms, bonding electrons spend more time closer to an atom with higher electronegativity than that with lower electronegativity. The probability of a bonding pair spending time close to an atom with lower electronegativity is low. Teachers can extend this concept to ionic bonding and can emphasise that in ionic bonding the bonding pair spends much more time with the more electronegative atom and least time with the less electronegative atom. However, the probability for bonding electron pair(s) to spend time with a less electronegative atom is not zero as supported by VBT, that is, the wave function of a valence bond is a linear combination of A+B−, AB and A−B+ states. This sequence can help students learn that an overlap of atomic orbitals is essential for ionic bonding and the probability of complete electron transfer from an atom to another is high but not 100%. Hence, students can visualize the importance of atomic overlap during ionic bonding.
Scientists have used the difference in electronegativities of two elements to classify the chemical bonds as: non-polar covalent, polar covalent and ionic bonds. When the difference of electronegativity is 0.4 or less, the bond is usually non-polar covalent; between 0.4 and 1.7, the bond is usually polar covalent and for ≥1.7, the bond is usually ionic. The word ‘usually’ used in the above phrases is an indicator of uncertainty of cutoff points. The readers should therefore consider that there are grey areas around differences of 0.4 and 1.7 as supported by the word ‘usually’ that is used in the classification criteria. For example a bond can be classified as ionic with an electronegativity difference of 1.4 and covalent with difference >0.4. For example ZnBr and AgCl with electronegativity differences of 1.2 and 1.6 respectively are ionic. HF with electronegativity difference of 1.9 is polar covalent. These grey areas also suggest that there is an overlap of atomic orbitals during the formation of ionic bonds. Hence, electronegativity and overlap of atomic orbitals can be used to explain covalent, polar covalent and ionic bonding.
Discussion
The discussion is reported under three headings: learning science perspective, links to other chemistry concepts, and limitations. These headings describe how the proposed pedagogy on chemical bonding is linked to psychology of learning and to those concepts which students have learned prior to and post learning of chemical bonding. Moreover, some concerns about its limitations are also discussed.
Learning science perspective
Human learning may occur as part of education, personal development, schooling, or training. It is well recognised that learning including of chemistry concepts should build on theories and models of human cognition (information processing) and psychology of learning (see Taber, 2013). These aspects are discussed below under these two headings.
Information processing perspective.
Human information processing involves a large number of cognitive processes and each process requires some time to complete (see Anderson and Demetrius, 1993). Each cognitive process takes time and slows down the information processing rate and increases wait time (Dhindsa, 2010). Therefore, a way to improve the information processing rate is to minimize the use of these processes. The hippocampus is a region within the temporal lobe of the cerebral cortex and it plays a significant role during the processes of coding and consolidation (Abiola and Dhindsa, 2012). However, the hippocampus is bypassed when information is meaningfully learned or permanently stored and well linked to previously stored information. Bypassing the hippocampus helps to reduce the number of cognitive processes involved in activating and transferring the desired information from long term memory to short term memory for fast interpretation of new information. It also means that when stored information is properly chunked together in a cognitive structure, it can bypass the hippocampus. If we consider memory capacity in terms of Miller's magic number of 7 ± 2 (recently revised to 4 ± 1; Mathy and Feldman, 2012), it is limited. To make effective use of it, larger and effectively linked cognitive structures (chunks of knowledge) need to be built so that students can bring larger chunks of prior knowledge to support new learning. This can help learners use memory space efficiently to learn new knowledge. Learning occurs by (a) adding completely new information to the brain without any link to existing memory (rote learning), (b) completely replacing the existing cognitive structure (replacing a misconception) (c) modifying the existing cognitive structure (correcting partial misconceptions), (d) appending new information to the existing cognitive structure (enlarging existing cognitive structure) and (e) a mixture of all four. The most effective way to learn is to append new information to the existing cognitive structure with minimum modifications. This idea is supported by Jensen (2000) who stated that new neuron connections should be established with minimum loss of existing connections. In this way, the role of the hippocampus is minimised. To achieve this outcome, teaching and learning should meet the criteria of sustainable learning (Dhindsa, 2011; Abiola and Dhindsa, 2012). The proposed sequence builds on previous concepts. For example, the concepts of covalent and polar covalent bonding are well connected and require limited modification when polar covalent bonding is taught after covalent bonding. These modifications can be mostly achieved by appending new information to the existing cognitive structure describing the covalent bond. Similarly, information on ionic bonding can be added to polar covalent bonding with little additional modifications to the above stated cognitive structure representing covalent and polar covalent bonding. The overall cognitive structure can be modified to teach lattice (metallic) bonding. Each time students can activate a larger cognitive structure to effectively append additional information to it. Hence students can use their existing small short-term memory effectively. In this way, we can increase the size of a cognitive structure with minimum modification to it.
Psychology of learning perspective.
Learning is a lasting change in behavior that results from experience. The main assumption behind all learning psychology is that the environment, conditioning, and reinforcement affect human behavior. It is well documented that reinforcement plays a significant role in bringing permanent behavior change and its role is more prominent to support rote learning of completely new concepts that are used to support meaningful learning of more new knowledge (Ausubel, 2000). Piaget (1970/1972) emphasized the roles of assimilation, disequilibration, accommodation and equilibration in learning. The proposed pedagogy starts with single bond formation with equal sharing of a single electron between two H-atoms of the same electronegativity value. The O-level school syllabus in Brunei shows that students (age 15+ years) learn diatomic molecules (H2, O2, N2, I2) in the chapters on kinetic particle theory and atomic theory (including shells, number of electrons in shells, molecular mass and gases) prior to bonding. This means covalent bonding is not a completely new concept because there are anchors to link this information.
Teachers can activate students’ prior knowledge by reminding them of the existence of a stable hydrogen molecule consisting of two hydrogen atoms which they have learned previously in the above stated chapters. Students can use this information to interpret new information (assimilation). A question dealing with why two H-atoms form a stable molecule is new information and will create a state of disequilibration because the students cannot explain it with their existing knowledge. To facilitate the accommodation process, teachers can take examples from daily life of friendships between two students that are based on sharing of some common traits between friends. The concept of sharing can now be further extended stating that hydrogen atoms have one electron to share and two H-atoms share a pair of electrons that increases the force of attraction between the two atoms and it is defined as a bond. This concept is new and students have to partly rote-learn it and partly learn meaningfully as it has been linked to their daily life experience. The students now will modify their existing knowledge about a hydrogen molecule to include a bond formation as a result of electron sharing using the accommodation process to reach a state of equilibration. Here we would recommend that teachers introduce the concept of overlap of atomic orbitals for the sharing of electrons and activate the concept of electronegativity to support the position of bonding electrons between two atoms. When both atoms are equally sharing the bonding electron pair it is in the middle of both atoms (H–H), if one H-atom is using both electrons, the other will not be able to use them (H−H+) and vice versa (H+H−).
In this way teachers can lay the foundation for introducing concepts of all bonds being covalent, polar covalent and ionic as explained by the valance bond theory. We will not recommend linking bonding to the octet rule as it does not apply to hydrogen and many other molecules. Rather, we recommend that while teaching electronic configuration, s, p and d orbitals should be introduced to students. Also students should be able to determine the orbital to which the last electron is added using a staircase type model as proposed by some authors (Chang, 1988; Dhindsa, 2011). Since in the case of hydrogen, the first and last (only one) electron enters the s-orbital, therefore to complete this s-orbital hydrogen requires two electrons. Hence only one pair of electrons is shared between two hydrogen atoms to form a bond. The above process will be repeated (reinforcement) to explain the formation of double and triple bonds in O2 and N2 molecules, respectively. The students will use explanations learned about the hydrogen molecule to assimilate, accommodate and equilibrate information related to O2 and N2 molecules: the overlap of atomic orbitals and the role of electronegativity to determine the position of shared electron pairs between bonding atoms.
Teachers can ask students to draw various possible ways that three electron pairs can be shared between two nitrogen atoms bonded using a triple bond (N:::N; N: ::N, N:: :N, …). Hereafter, bonding between different atoms (polar covalent bonding) is discussed and the role of electronegativity in determining and explaining the position of the shared pair will predominate. As soon as a teacher poses a new question, for example, relating to what happens when atoms with different electronegativity form a bond such as the C–H bond, well-informed students will certainly use their prior knowledge to assimilate the position of electrons towards the more electronegative atom, whereas other students will experience disequilibration. With the help of the teacher's explanations/activities, the concept of equal sharing for bonding will be modified to reach equilibration. The concept of covalent bonding will be modified meaningfully to polar covalent bonding using processes of assimilation, disequilibration, accommodation and equilibration as proposed by Piaget as well as rote learning and meaningful learning through reinforcement as proposed by Ausubel (2000). The students will learn the concept of bond polarity and its association to electronegativity. The teachers should reinforce the concepts of atomic orbital overlap and the role of electronegativity using other examples. Lastly, teachers can extend the concept of polar bond to ionic bond, which can be defined as an example of a maximum polar bond. The above discussion supports the proposed sequence of teaching bonding that is well supported by the psychology of learning. However, when we consider the existing teaching sequence, that is teaching of ionic bonding first and then covalent bonding, the students' prior knowledge of ionic bonding concepts is not used by teachers to teach covalent bonding. Rather teachers introduce covalent bonding as a completely different/new unconnected concept; because electron transfer and sharing are distinctly different for teachers and students, students are unable to link ionic bonding to covalent bonding. The readers can realize that teaching of ionic bonding prior to covalent bonding is not as well supported as the proposed sequence by the theories of learning.
In this article, the authors have suggested that the proposed order for teaching chemical bonding concepts is supported by the psychology of learning including the constructivist theory of learning. Under these circumstances, the learning of these concepts should also be linked to related concepts taught at lower grades and those to be taught at upper grades.
Links to other chemistry concepts
This section is divided into two parts: links to prior learning and links to post learning. Links to prior learning deals with how chemical bonding links with concepts students have learned prior to learning of this concept and links to post learning deals with its links to concepts taught after the teaching of chemical bonding.
Links to prior learning.
The concept of bonding is usually taught after students have learned about atoms, molecules and ions; molecular and ionic compounds; gases; kinetic particle theory, electronic structure of the atom and the periodic table (Cambridge O-level syllabus, 2014). This means students know the existence of stable diatomic molecules, molecular compounds, structure of atoms, shells, electrons in shells and their motion. Ion formation and electronegativity are introduced prior to teaching ionic and polar covalent bonding, respectively. These concepts play an essential role in explaining chemical bonding. Moreover there are a number of analogies available to extend the explanation of these concepts to link with bonding. The knowledge of these concepts can provide enough anchors for students to construct new knowledge on chemical bonding when taught using the proposed pedagogy.
Links to post learning.
(a) Application of valence bond theory.
In this teaching sequence, electronegativity determines the high probability area where bonding electrons can be found and an overlap of atomic orbitals is proposed for electron sharing and transfer between bonding atoms. This proposal is in line with the recent development in VBT; that is the existence of covalent [AB (NaCl)] and ionic [A+B− (Na+Cl−); A−B+ (Na−Cl+)] forms in each type of bonding. However, the probability of finding the bonding electron pair in regions outside the specified regions by overlapping of atomic orbitals is not zero; otherwise formation of Na−Cl+ becomes impossible. Teachers can introduce examples (C–H; N–H) where the electronegativity difference between bonding atoms is small to introduce the concept that it is also possible for a bonding electron pair to move to atoms with lower electronegativity values, thus producing a reversal of change of the bonding atoms. A part of Fig. 1 can be transformed to Fig. 2 for visual representation of such reversal. The students can learn and observe that an electron pair can completely transfer to bonding atoms with low electronegativity. When Fig. 1 and 2 are considered together, the students can visualize the existence of a cases of covalent, polar covalent and ionic bonding (also see Fig. 3).
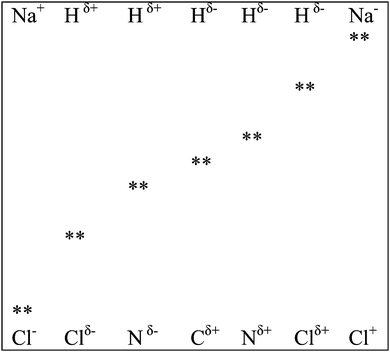 |
| Fig. 2 Continuum of bonding with bonding electrons in high and low probability areas. | |
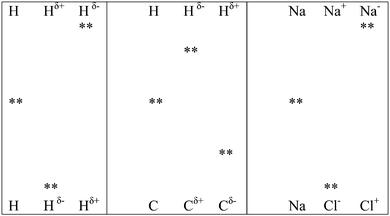 |
| Fig. 3 Continuum of bonding with bonding electrons in high and low probability areas. | |
Tertiary teachers can use Fig. 3 and 4 to demonstrate the existence of three clear bonding states for covalent, polar as well as for ionic bonds. They can also use information from Fig. 4 to explain the polar covalent bonding concept in ionic bonding. This arrangement is impossible without an overlap of atomic orbitals of bonding atoms. Hence teachers can teach the mathematical concept ψVB = ψ(A − B) + aψ(A−B+) + bψ(A+B−) by linking it to what students have learned at school.
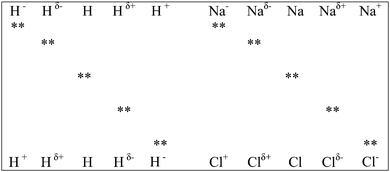 |
| Fig. 4 Possible covalent, polar covalent and ionic bonding states in H2 and NaCl. | |
The authors of this article also believe that with the help of advanced mathematical modeling techniques and high power computers we may be able to compute solutions to more complex models and the valence bond representation may change from eqn (1):
| ψVB = ψ(A − B) + aψ(A− + B+) + bψ(A+ + B−) | (1) |
to
eqn (2):
|  | (2) |
where
δi is the charge at
ith location between bonding atoms and its value changes from a value >0 to 1.0, and b
i and d
i represent the magnitude of contributions of each function. For
δi = 1, the function should represent the contribution of an ionic state and for all other cases (
δi value between 0 and 1 ends excluded) the function represents polar covalent states. A simple graphic representation of bonding between hydrogen–hydrogen, and, sodium–chlorine atoms using the above formula is given for limited values of
δi in
Fig. 4. This graphic demonstrates a continuous spectrum for shifting a bonding pair of electrons between the bonding atoms.
(b) Classifying compounds as covalent, polar covalent and ionic.
A common tradition is to classify compounds into three categories: covalent, polar covalent and ionic. Once we accept that there are no pure types of bonds, it becomes difficult to classify compounds into these categories. Since all bonds exhibit five possible characteristics as shown in Fig. 4, the best way to classify a compound as covalent, polar covalent or ionic is to look for a dominant character of the bond. For example, in the case of the bond between hydrogen atoms to form H2 molecule, the H:H character will be dominant, therefore hydrogen is classified as covalent. Similarly, sodium chloride is ionic as Na+ Cl− predominates and HCl is polar covalent as Hδ+ Cl− predominates.
(c) Resonance structures.
In chemistry, certain molecules or polyatomic ions cannot be expressed by one single Lewis formula. Such molecules or ions are presented by more than one structure and each contributes to the actual structure. These structures are called resonance structures. Resonance is a way of describing delocalized electrons within certain molecules or polyatomic ions. This is an important topic in chemistry that students find difficult to understand. Appropriate prior knowledge is required to link this concept for effective teaching. This model of teaching chemical bonding can act as an anchor to teach resonance because the proposed model suggests teaching covalent and ionic bonding as a continuum spectrum, and students know that electrons can move to different locations during chemical bonding. Therefore it provides appropriate prior knowledge for tertiary teachers to develop this concept further. The university teachers may use this prior knowledge to teach contributions of each resonance structure to the overall bonding. For example the chance of obtaining F+ ion is small but not zero, hence H–F bonding is mainly contributed to by two resonance structures (H–F and H+F−).
(d) Molecular orbital theory.
Molecular orbital theory (MOT) and valence bond theory (VBT) provide two ways of support to each other. For example, overlap of atomic orbitals as in the proposed model of teaching chemical bonding also has support from molecular orbital theory which states that when atoms come close, a sufficient overlap must occur for actual bonding to take place and an insufficient overlap will not result in a strong bond (see Housecroft and Constable, 2010, p. 151). However, such overlap would allow electrons to flow from one atom to another.
On the other hand when teaching the MOT wave function for the hydrogen molecule, the VBT wave function can be used as an anchor. These wave functions are described below.
ψVB = ψ(A − B) + aψ(A− + B+) + bψ(A+ + B−) |
ψMO = ½[1SA(1)1SB(2) + 1SA(2)1SB(1)] + ½[1SA(1)1SA(2) + 1SB(1)1SB(2)] |
The first part of the MO wave function consisting of two terms represents the ionic states of the molecule (
Barrow, 1996). It is the same as is the case in VBT. Therefore, when teaching MOT, tertiary teachers can use the wave function of VBT as prior knowledge to develop the concept of MOT.
While considering metallic bonding, most metals have outermost electron/s in a s-orbital – a spherical space. The spherical nature of the orbital facilitates overlap between multiple atoms depending upon the atomic size, though restricted by number of other factors. Similarly, in ionic lattices, the outermost orbitals of non-metals can establish an overlap with the s-orbital of outermost metal atoms, and electronegativity can guide the movement of electron density between bonding atomic identities.
Limitations
This section is divided into four sections: octet rule, teaching atomic orbitals prior to chemical bonding teaching, ionic, covalent and polar covalent sequence and misconceptions.
Octet rule.
The octet rule has been widely used in textbooks as well as by teachers to explain chemical bonding (see Chang, 1988; Zumdahl, 1998; Housecroft and Constable, 2010), but this rule has limited application to explain the formation of some molecules. However, there are many exceptions to this rule which have been linked to students' misconceptions. Considering exceptions, the octet rule cannot explain bonding of some elements in electron deficient compounds (see H2, BeCl2 and BCl3), with elements following the octet rule in some compounds and not following the rule in other compounds. For example, sulfur in SF2 has 8 valence electrons, whereas in SF4 and SF6 sulfur has 10 and 12 valence electrons, respectively. Similarly, the octet rule is unable to explain bonding in stable free radicals such as nitrogen(IV) oxide (NO2). Moreover, some researchers have highlighted misconceptions associated with the use of the octet rule (Taber, 1995, 2000; Dhindsa and Treagust, 2009). We therefore decided against its use.
Teaching atomic orbitals prior to chemical bonding teaching.
It is true that the secondary school curriculum does not require teaching atomic orbitals before teaching chemical bonding because the O-level curriculum does not need this information to define chemical bonding. We know that science information is dynamic in nature. New knowledge is generated and outdated knowledge is discarded at a fast rate. Therefore this information can be accommodated in its simplest form such that originality of the information is not changed; otherwise students may oversimplify information as a hybrid model which can lead to misconceptions (Justi and Gilbert, 2002). To use this model, the introduction of 1s, 2s and 2p orbitals is required. The first shell is renamed as 1s, hence only 2s and 2p orbitals are to be taught. Teaching of these concepts is not difficult and it can be further facilitated by the use of ICT. We therefore do not consider it as a serious limitation.
Ionic, covalent and polar covalent sequence.
In this article we proposed the covalent, polar covalent and ionic bonding sequence for effective teaching and have demonstrated that it is well supported by the constructivist psychology of learning. However, readers may think that the existing sequence can also be supported by learning theories, given that proper care is taken to explain the crucial aspects such as covalent and ionic bonds mainly produce covalent and ionic compounds, respectively. We do agree with the above statement because chemistry concepts of bonding are highly interlinked. However, for effective teaching we opt for a sequence that can be easily explained using multiple teaching modes such as visualization of the position of bonding electrons in the proposed model. Moreover, if we link the covalent and ionic molecules, we are comparing the product of bonding aspect rather than the process aspect of bonding. Therefore we believe, the proposed order is better supported that other sequences.
Misconceptions.
There is extensive research associated with misconceptions about chemical bonding concepts (Taber, 2000; Justi and Gilbert, 2002; Taber and Coll, 2002; Dhindsa and Treagust, 2009). These studies have highlighted a number of sources for the misconceptions including the teachers' content knowledge, the way information is presented to students and oversimplification of the content. Taber (2000) stated that teaching models should reflect optimum level of simplifications without losing authenticity of scientific content. He further added that it can be achieved but if proper care is not taken, teaching may impede learning and add misconceptions. We believe these factors will also have an impact on the teaching of this proposed sequence. Misconceptions can be minimized by teaching authentic scientific content with the use of information communication technology (Barke et al., 2009). Moreover, we need to revisit the process of identifying the misconceptions.
In the past, all our identification tools were based on the fact that we taught all bonding types as distinct: ionic bonding has been associated with electron transfer and covalent bonding with electron sharing. There has been little or no emphasis on teaching that all bonds are covalent, polar covalent and ionic to varying extents in nature. Emphasis on all bonds being covalent, polar covalent and ionic will increase with the adaptation of the proposed pedagogy. We acknowledge that the proposed sequence according to Taber and Coll (2002) may cause other student difficulties: for instance, to teach covalent bonding before ionic bonding may cause students to see an ionic lattice and all bonded materials as containing molecules. It will be interesting to see the impact of the proposed pedagogy on students' and teachers' misconceptions when their knowledge and understanding is evaluated in the above context.
Future research directions
The proposed model is a theoretical model based on its sound foundation in the psychology of learning and human information processing models and theories. However, its actual success in classroom situations needs to be evaluated. Future research therefore needs to be directed to evaluating the impact of the proposed pedagogy on students' actual learning and understanding of the bonding concepts as well as on their overall achievement. There is also need to ascertain whether or not the proposed pedagogy helps to minimize students' alternative conceptions. Moreover, research into explanations of intermolecular attraction forces and bonding in lattices is also warranted.
Conclusions
This study proposes a sequence to teach chemical bonding (covalent, polar covalent and ionic bonding) for effective and sustainable learning. The use of electronegativity and overlap of atomic orbitals for all types of bonding has also been stressed. The proposed sequence and emphasis on electronegativity and atomic orbital overlap meets the criteria for teaching and learning of concepts of learning theories of psychology including constructivism. This sequence not only provides a better link between the bonding concepts at school but can also be easily linked to what students will learn at the tertiary level. The authors have considered some limitations for the proposed order. Therefore, the proposed teaching model details the advantages of a different approach to teaching chemical bonding to the one currently used in the classroom. When using this teaching model, we recommend that teachers explicitly make students aware of the range of tools such as models, representations of various kinds and metaphors that are used to explain different kinds of bonds. Future research in applications of the model is recommended.
References
- Abiola O. O. and Dhindsa H. S., (2012), Improving classroom practices using our knowledge of how the brain works, Int. J. Environ. Sci. Educ., 1(7), 71–81.
- Anderson O. R. and Demetrius O. J., (1993), A flow-map method of representing cognitive structure based on respondents' narrative using science content, J. Res. Sci. Teach., 30(8), 950–969.
- Ausubel D. P., (2000). The acquisition and retention of knowledge: a cognitive view, Dordrecht: Kluwer Academic Publishers.
- Barke H. D., Hazari A. and Yitbarek S. (2009), Misconceptions in chemistry, Berlin: Springer-Verlag.
- Barrow G. M., (1996), Physical Chemistry, 6th edn, London: McGraw-Hill.
- Bergqvist A., Dreschler M., De Jong O. and Rundgren S.-N. C., (2013), Representations of chemical bonding models in school textbooks – help or hindrance for understanding? Chem. Educ. Res. Pract., 14, 589–606.
- Bodner G. M., (1992), Why changing the curriculum may not be enough, J. Chem. Educ., 69 (3), 186–190.
- Boujaoude S. and Barakat H., (2000), Secondary school students' difficulties with stoichiometry, Sch. Sci. Rev., 296, 91–98.
- Brown T. L., Murphy C. J., Lemay Jr., H. E., Langford S. J., Bursten B. E. and Sagatys D., (2009), Chemistry – The central science: A broad perspective, Boston: Prentice.
- Cambridge O-level Syllabus, (2014), SYLLABUS, Cambridge O Level Chemistry 5070, http://www.cie.org.uk/images/90185-2014-syllabus.pdf, retrieved on June 1, 2014.
- Chang R., (1988), Chemistry, New York: Random House.
- Coll R. K. and Treagust D. F., (2001), Learners' mental models of chemical bonding, Res. Sci. Educ., 31, 357–382.
- Coll R. K. and Treagust D. F., (2002), Exploring tertiary students' understanding of covalent bonding, Res. Sci. Technol. Educ., 20, 241–267.
- Cooper M. M. and Klymkowsky M. W., (2013), The trouble with chemical energy: why understanding bond energies requires an interdisciplinary systems approach, CBE Life Sci. Educ., 12(2), 306–312.
- Dhindsa H. S., (2000), Using students' pre-conceptions for teaching chemistry concepts meaningfully: an action research project, J. Appl. Res. Educ., 4(1), 38–50.
- Dhindsa H. S., (2002), Pre-service science teachers' conceptions of pH, Aust. J. Educ. Chem., 60, 19–24.
- Dhindsa H. S., (2010), Teacher communication in Bruneian secondary science classes: wait-time, Asia Pac. J. Educ. Educ., 25, 73–88.
- Dhindsa H. S., (2011), Teaching for sustainable content learning, Chem. Edu. J., 14(1), (Serial no. 26), http://chem.sci.utsunomiya-u.ac.jp/v14n1/1Dhindsa/harkirat.html.
- Dhindsa H. S. and Emran S. H., (2006), Use of the interactive whiteboard in constructivist teaching for higher student achievement, in Stewart S. M., Olearski J. E. and Thompson D., (ed.), Proceedings of the Second Annual Conference for Middle East Teachers of Science, Mathematics and Computing, Abu Dhabi, UAE: METSMaC, pp. 175–188.
- Dhindsa H. S. and Treagust D. F., (2009), Conceptual understanding of Bruniean tertiary students: chemical bonding and structure, Brunei International Journal of Science and Mathematics Education, 1(1), 33–51.
- Dunlosky J., Rawson K. A., Marsh E. J., Nathan M. J. and Willingham D. T., (2013), Improving students' learning with effective learning techniques: promising directions from cognitive and educational psychology, Psychol. Sci. Public Interest, 14(1), 4–58.
- Gabel D., (1999), Improving teaching and learning through chemistry education research: a look into the future, J. Chem. Educ., 76, 548–554.
- Hill W. J., McCreary T. W. and Kolb D. K., (2010), Chemistry for changing times, 12th edn, New York: Prentice Hall.
- Housecroft C. E. and Constable E. C., (2010), Chemistry, 4th edn, Pearson Education Ltd: Essex, England.
- Hurst O., (2002), How we teach molecular structures to freshmen? J. Chem. Educ., 79(6), 763–764.
- Jensen E., (2000), Brain-based learning: the new science of teaching and training, revised edn, Alexandria, VA: ASCD Press.
- Johnstone A. H., Morrison T. I. and Reid N., (1981), Chemistry about us, London: Heinemann Educational Books.
- Justi R. and Gilbert J. K., (2002), Modelling, teachers' views on the nature of modelling, implications for the education of modelers, Int. J. Sci. Educ., 24(4), 369–387.
- Kirkwood V. and Symington D., (1996), Lecturer perceptions of student difficulties in a first-year chemistry course, J. Chem. Educ., 73(4), 339–340.
- Mathy F. and Feldman J., (2012), What's magic about magic numbers? Chunking and data compression in short-term memory, Cognition, 122, 346–362.
- Nahum T. L., Mamlok-Naaman R. and Hofstein A., (2008), A new bottom up framework for teaching chemical bonding, J. Chem. Educ., 85(12), 1680–1685.
- Nahum T. L., Mamlok-Naaman R., Hofstein A. and Krajcik J., (2007), Developing a new teaching approach for chemical bonding concept aligned with current scientific and pedagogical knowledge, Sci. Educ., 91, 579–603.
- Nahum T. L., Mamlok-Naaman R., Hofstein A. and Taber K. S., (2010), Teaching and learning the concept of chemical bonding, Stud. Sci. Educ., 46(2), 179–207.
- Nakhleh M. B. (1992), Why some students don't learn chemistry, J. Chem. Educ., 69, 191–196.
- OPSU, (2010), Oklahoma Criteria for Effective Teaching, http://www.opsu.edu/.../OklahomaCriteriaforEffectiveTeaching.htm, retrieved on 23/4/2010.
- Ozmen H., (2004), Some student misconceptions in chemistry: a literature review of chemical bonding, J. Sci. Educ. Technol., 13, 146–159.
- Piaget J., (1970/1972), The principles of genetic epistemology, London: Routledge & Kegan Paul.
- Stevens S. Y., Shin N. and Peek-Brown D., (2013), Learning progressions as a guide for developing meaningful science learning: a new framework for olds ideas, Educ. Quim., 24(4), 381–390.
- Taber K. S. (1995). Prior learning as an epistemological block? The octet rule – an example from science education. A paper presented at the European Conference on Educational Research, incorporating the annual research conference of the British Educational Research Association at University of Bath, http://www.leeds.ac.uk/educol/documents/00001474.htm , retrieved on 11 February, 2014.
- Taber K. S., (2000), Multiple frameworks?: evidence of manifold conceptions in individual cognitive structure, Int. J. Sci. Educ., 22(4), 399-417.
- Taber K. S., (2001), Building the structural concepts of chemistry: some consideration from educational research, Chem. Educ.: Res. Pract. Eur., 2(2), 123–158.
- Taber K. S. (2011), A master class in teaching the topic of bonding, basing chemical explanation on physical forces, Educ. Chem., May issue, http://www.rsc.org/Education/EiC/issues/2011May/ChemicalBonding.asp.
- Taber K. S., (2012), Upper secondary students' understanding of the basic physical interactions in analogous atomic and solar systems, Res. Sci. Educ., 1–30, DOI: 10.1007/s11165-012-9312-3.
- Taber K. S., (2013), Revisiting the chemistry triplet: drawing upon the nature of chemical knowledge and the psychology of learning to inform chemistry education, Chem. Educ. Res. Pract., 14, 156–168.
- Taber K. S. and Coll R., (2002), Chemical Bonding, in Gilbert J. K. et al., (ed.), Chemical Education: Research-based Practice, Dordrecht: Kluwer Academic Publishers BV, pp. 213–234.
- Taber K. S., Tsaparlis G. R. and Nakiboglu C., (2012), Students conceptions of ionic bonding: patterns of thinking across three European contexts, Int. J. Sci. Educ., 34(18), 2843–2873.
- Timberlake K. C., (2010), General, organic and biological chemistry structures of life, London: Prentice Hall.
- Tsaparlis G. R. (2000). The states-of-matter approach (SOMA) to introductory chemistry, Chem. Educ. Res. Pract., 1, 161–168, DOI: 10.1039/A9RP90017A.
- Tsaparlis G. and Pappa E. T. (2011), Types of intra- and intermolecular bonding: the case of general chemistry textbooks. E-Proceedings of the ESERA 2011 Conference, http://www.esera.org/ and select Publications/ESERA Conference Proceedings/Proceedings of ESERA 2011/Strand 2, retrieved on May 8, 2014.
- Watson A. and Mason J., (2006), Seeing an exercise as a single mathematical object: using variation to structure sense-making, Mathematical Thinking and Learning, 8, 91–111.
- Wimmer F. L. and Dhindsa H. S., (2005), Effects of teaching higher level content on students' understanding of and confidence in lower level content knowledge, in Dhindsa H. S., Kyeleve I. J., O. Chukwu and Perera J. H. S. Q. (ed.), Future directions in science, mathematics and technical education. Gadong: Universiti Brunei Darussalam: ETC.
- Wimmer F. L., Dhindsa H. S., Seddigi Z. S. and Khaled M. K., (2006). Teaching and learning chemistry: chemical bonding, Shaping the future of science, mathematics and technical education, Gadong: Universiti Brunei Darussalam-ETC, pp. 33–41.
- Zumdahl S. S., (1998), Chemical principles, New York: Houghton Mifflin Company.
|
This journal is © The Royal Society of Chemistry 2014 |