DOI:
10.1039/C4RA12069H
(Paper)
RSC Adv., 2014,
4, 64347-64353
Application of the polyacrylonitrile fiber as a support for the green heterogeneous base catalyst and supported phase-transfer catalyst†
Received
9th October 2014
, Accepted 13th November 2014
First published on 18th November 2014
Abstract
A facile synthesized polyethylene polyamine functionalized polyacrylonitrile fiber (PANF-PA), capable of acting as the heterogeneous base catalyst and supported phase-transfer catalyst (SPTC), was presented in Knoevenagel condensation–cyclization and nucleophilic substitution for the synthesis of a number of substituted iminocoumarins and sulfones in aqueous systems, respectively. Excellent results are shown in terms of simple procedures, high chemical yields (up to 96%), extensive applicability and superior catalytic recyclability even for 10 cycles. The deeper active principle of the PANF-PA as SPTC was explained through detailed characterization by means of elemental analysis, FTIR spectroscopy and SEM. Moreover, the procedures can be effectively scaled up, and the solvent can be easily recovered. As well as, the prominent features (high strength, good flexibility etc.) of the polyacrylonitrile fiber are very attractive for fixed-bed reactors in the chemical industry.
Introduction
In contemporary synthetic chemistry, the development of environmentally benign and cost-efficient catalytic systems to minimize waste production and maximize catalyst efficiency has become one of the main themes.1,2 And with this objective, the application of novel and recyclable catalysts for organic synthesis has attracted much attention.3 Among those, the heterogenization of homogeneous catalysts onto solid supports has been well investigated and proved to be more crucial to chemical processes, and it will continue to influence and motivate the development of catalysts for environmental remediation.4,5 Currently, the commonly used materials for heterogeneous catalysts are polymers,6,7 mesoporous materials,8,9 and nanoparticles,10,11 exploiting new supports for the more facile heterogeneous type catalytic synthesis, which may quickly move on from the laboratory to the green industrial plant, has received increasing interest.12,13 However, finding a new support with prominent stability for heterogeneous catalysis that provides the desired product in good catalytic activity is very often at the beginning of a long journey.
Polyacrylonitrile fiber (PANF) is known as “artificial wool” and has been widely applied not only in civilian use but also in industry. Given many advantages of PANF, such as low cost and simple production technology, corrosion and mildew resistant, high strength and light density, as well as its contains an abundance of cyano groups which can be easily transformed into carboxyl, amide or other functional groups,14–16 PANF provides an appealing option to other synthons as a support for heterogeneous catalysts. Moreover, the good flexibility of fiber makes it convenient for knitting into the desired shapes, which is very attractive for the fixed-bed reactors in chemical industry. Nevertheless, there are few heterogeneous catalysts based on PANF, so it is highly desirable to develop novel and green catalysts based on PANF. In our previous work, the polypropylene fiber was used as the new support for heterogeneous Brønsted acid catalyst17 and supported ionic liquids catalysis.18 Besides, the modified amine-functionalized PANF was also used to catalyze Knoevenagel condensation and achieved excellent results.19 Following the pioneering studies of our work on fiber catalyst,20 especially the excellent results obtained in aqueous systems, which inspired us to further investigate the use of PANF as support for designing novel heterogeneous catalysts. In the present study, we report a convenient synthesized polyethylene polyamine functionalized PANF (PANF-PA), function as heterogeneous base catalyst and supported phase-transfer catalyst (SPTC), which were verified for the synthesis of iminocoumarins and sulfones in aqueous systems, respectively.
Results and discussion
The preparation of PANF-PA
The PANF-PA was prepared according to the amination procedure as shown in Scheme 1. As discussed in our previous work,19 the amination was found to be strongly influenced by the reaction time and temperature, and the extent of immobilization was measured by weight gain (weight gain = [(W2 − W1)/W1] × 100%, where W1 and W2 are the weight of the fiber sample before and after amination, respectively) and acid exchange capacity. Under the screened condition of amination with polyethylene polyamine in water, a weight gain of 35% PANF-PA was obtained successfully and found to be with opportune loading and strength, and the corresponding acid exchange capacity in aqueous 0.1 M HCl was determined to be 4.02 mmol g−1 (see ESI†).
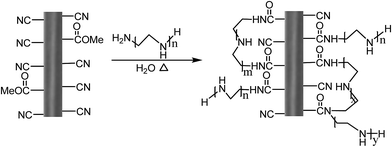 |
| Scheme 1 Preparation of the fiber catalyst PANF-PA. | |
The catalytic properties of PANF-PA
PANF-PA as heterogeneous base catalyst for the synthesis of iminocoumarins. Natural and synthetic coumarins have demonstrated a broad spectrum of applications in biology and medicine.21,22 Moreover, their 2-imino analogues, iminocoumarins, are less known but can be excited at longer wavelengths than coumarins, and comprising a very important class of protein tyrosine kinase (PTK) inhibitors with low molecular weight.23,24 Nevertheless, most of the currently used methods for the synthesis of iminocoumarins suffer from several shortcomings, including the use of non-recoverable catalysts, high-power microwaves, long periods of reaction time, troublesome procedures as well as limited substituents.25–27 Exploiting efficient approaches to iminocoumarins would be of great interest and value. Thus the efficacy of the PANF-PA function as heterogeneous base catalyst was evaluated via Knoevenagel condensation–cyclization between salicylaldehydes and activated nitriles to afford a wide variety of iminocoumarins (Scheme 2).
 |
| Scheme 2 Synthesis of iminocoumarins catalyzed by PANF-PA in a mixed-aqueous system. | |
Based on our previous work,19 and to gauge the scope of this fiber catalyst, a variety of substituted activated nitriles were selected to react with salicylaldehydes in the presence of 5% mol of PANF-PA loading in a mixed-aqueous system (ethanol–water) to produce the corresponding iminocoumarins (Table 1). It is gratifying that the catalytic system is of excellent group tolerance, whether activated nitriles (Table 1, entries 1–6) including ordinary malononitrile, 4-nitrophenylacetonitrile, complicated nitriles such as 4-tosylacetonitrile and N-benzyl-2-cyanoacetamide, or different substituted salicylaldehydes (Table 1, entries 7–10) reacted efficiently to afford the corresponding products in good to excellent yields. Moreover, it is worth mentioning that there was no evident electronic influence of the substituents on the aryl ring of salicylaldehydes on the yields of iminocoumarins. However, the steric effect and electronic effect on the activated nitriles had observable influence on the yield of the synthesized products. For instance, the substrate of 4-nitrophenylacetonitrile (Table 1, entry 1) obtained a high yield due to its bigger steric effect which was favorable for formation of trans Knoevenagel condensation product, and further in favor of the next cyclization, nevertheless, benzoylacetonitrile and 4-tosylacetonitrile (Table 1, entries 3 and 4) with high-activity for Knoevenagel condensation and against for trans products, and finally afforded slightly lower yields of the corresponding iminocoumarins.
Table 1 PANF-PA-mediated synthesis of iminocoumarinsa
Entry |
Salicylaldehyde |
Activated nitrile |
Product |
Time (h) |
Yieldb (%) |
Reaction conditions: salicylaldehydes (2 mmol), activated nitriles (2 mmol) and PANF-PA (0.025 g, 5 mol% catalyst loading based on acid exchange capacity) in water–EtOH mixtures (10 mL, 2 : 8 v/v), under refluxing. Isolated yields. Blank control (without any catalyst). 5 mol% piperidine as the catalyst. |
1 |
 |
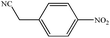 |
1a |
1.0 |
95 |
2 |
 |
 |
1b |
0.5 |
91 |
3 |
 |
 |
1c |
2.5 |
86 |
4 |
 |
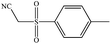 |
1d |
2.5 |
82 |
5 |
 |
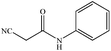 |
1e |
1.5 |
93 |
6 |
 |
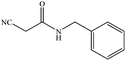 |
1f |
2.0 |
92 |
7 |
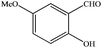 |
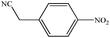 |
1g |
1.0 |
96 |
8 |
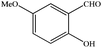 |
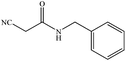 |
1h |
2.0 |
94 |
9 |
 |
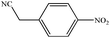 |
1i |
1.0 |
94 |
10 |
 |
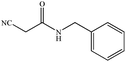 |
1j |
2.0 |
93 |
11 |
 |
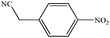 |
1a |
1.0 |
tracec |
12 |
 |
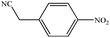 |
1a |
1.0 |
95d |
PANF-PA as supported phase-transfer catalyst for the synthesis of sulfones. The nucleophilic substitution is a crucial and frequently used transformation for the formation of C–C and C–heteroatom bonds in organic synthesis. However, it seldom carried out in neat water owing to the limited water solubility of organic substrates,28 and the most common way to solve this problem is the employ of phase-transfer catalyst (PTC) due to it facilitate reactions between water-soluble reagents and organic soluble substrates.29 The application of sulfones in organic synthesis has especially proven valuable in many of the most demanding and sophisticated total syntheses conducted,30,31 moreover, the polyethylene polyamine functionalized resin have been demonstrated with good phase-transfer catalytic activity.32 Therefore, we next extended the PANF-PA as the SPTC in nucleophilic substitution for the synthesis of sulfones (Table 2). A 5% mol catalyst loading of PANF-PA was used twice to mediate all the substituted halides and sodium 4-toluenesulfinate in neat water to obtain the corresponding sulfones. As list in Table 2, it can be seen that the reactions proceed smoothly with PANF-PA to give the products in excellent yields whether benzyl bromides or benzyl chlorides, and all the latter yields were higher than the former ones. It is worth noting that there was no significant influence of the position of the substituents on the yield of the synthesized products (Table 2 entries 3–5). However, the electronic nature of the substituents on the aryl ring of halides had an evident effect on the yield of sulfones (Table 2 entries 6–8). Besides, trace product formation was observed under the given reaction conditions with the inert haloalkane (Table 2, entry 10).
Table 2 PANF-PA-mediated synthesis of sulfonesa
The recyclability of the PANF-PA. As features of the heterogeneous catalysis system, the easy separation from the reaction mixture and the reusability of PANF-PA were also investigated in the reactions described above, which further proved the superior recyclability of the fiber catalyst during vigorous operating conditions. Under the same reaction conditions described in Tables 1 and 2, salicylaldehyde and 4-nitrophenylacetonitrile, benzyl bromide and sodium 4-toluenesulfinate were selected as the model substrates to examine the reusability of PANF-PA, respectively. After completion of each cycle, the PANF-PA was filtered out and washed with the corresponding solvents, then the recycled PANF-PA was used directly for the next cycle without any additional treatment. The results show that the reactions proceeded smoothly without extension of reaction time or marked loss in the yield (Fig. 1). Furthermore, the weight of PANF-PA before and after ten cycles was also detected. In the reaction for the synthesis iminocoumarin, there was almost no weight change of PANF-PA after ten cycles, which is consistent with our previous work.19 However, in the nucleophilic substitution for synthesis of sulfone, the weight of PANF-PA increased obviously from 0.025 to 0.033 g, which motivated us to further perform detailed characterization of the fiber catalyst in this process.
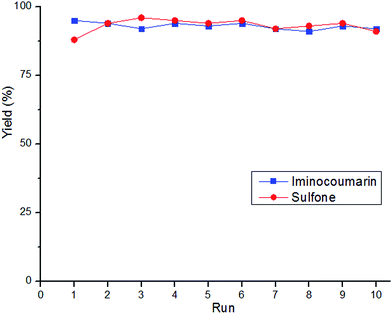 |
| Fig. 1 Recyclability of PANF-PA for the synthesis of iminocoumarin and sulfone. | |
The characterization of PANF-PA
In the nucleophilic substitution for the synthesis of sulfones, all the former yields were lower than the latter ones (Table 2) and the yield of first run (88%) in repeated reactions was lower than all of the subsequent ones (>92%). These results above suggested that there must be some changes on the fiber catalyst during catalytic process. After amination, the PANF-PA contains a lots amine group which can be easily reacted with benzyl bromides and the formation of ammonium salts. Perhaps it is due to the additional phase-transfer ability of ammonium salts, which further improved the catalytic activity of the fiber catalyst. Moreover, the modified amine-functionalized PANF has been characterized thoroughly in our previous work.19 So in order to verify our deduction and investigate the rangeability of fiber catalyst in nucleophilic substitution, we performed detailed characterization of the fiber samples in different stages. The original PANF, PANF-PA, the first recovered catalyst in the model nucleophilic substitution (PANF-PA-1) and the 10th recovered catalyst (PANF-PA-10) were all characterized by means of elemental analysis (EA), Fourier transform infrared spectroscopy (FTIR) and scanning electron microscopy (SEM).
Elemental analyses (EA). EA data of the PANF, PANF-PA, PANF-PA-1 and PANF-PA-10 are listed in Table 3. Compared to the original PANF, the carbon and nitrogen contents of PANF-PA decreased and the hydrogen content increased as expected (Table 3, entries 1 and 2). After PANF-PA being used as the catalyst in the nucleophilic substitution of benzyl bromide and sodium 4-toluenesulfinate, the amounts of carbon, hydrogen and nitrogen of PANF-PA-1 (Table 3, entry 3) all decreased, that perhaps due to the benzyl bromides reacted with the amine groups of the fiber, and it has no nitrogen, less carbon and hydrogen than PANF-PA. These results can be act as an evidence to prove the formation of ammonium salt on the fiber and verify our above inference. What is more, the EA data of the 10th recovered PANF-PA-10 (Table 3, entry 4) almost stayed the same with that of PANF-PA-1, which indicated that the fiber catalyst can be reused more times.
Table 3 EA data of PANF, PANF-PA, PANF-PA-1, and PANF-PA-10
Entry |
Fiber sample |
C (%) |
H (%) |
N (%) |
1 |
PANF |
65.55 |
5.669 |
23.54 |
2 |
PANF-PA |
54.92 |
7.057 |
20.37 |
3 |
PANF-PA-1 |
54.23 |
6.079 |
15.71 |
4 |
PANF-PA-10 |
54.18 |
6.072 |
15.56 |
Fourier transform infrared spectroscopy (FTIR). Samples of PANF, PANF-PA, PANF-PA-1 and PANF-PA-10 were pulverized by cutting and then prepared into KBr pellets. Their FTIR spectra are shown in Fig. 2. On comparing the FTIR spectra of the PANF-PA (Fig. 2b) with that of the PANF (Fig. 2a), the most striking change is the broad absorption bands around 3250 cm−1, which is characterized as the N–H stretching vibration. After used in nucleophilic substitution, the PANF-PA-1 (Fig. 2c) exhibited new absorption bands at 768 and 695 cm−1 that belongs to the vibrational modes of benzene ring, which can be act as another evidence to prove the formation of ammonium salt on the fiber (agree well with the EA data). Moreover, the specific absorption peak of the PANF-PA-10 (Fig. 2d) are quite similar to PANF-PA-1 but with a slight enhancement, which further verifies the above deduction and indicates that the active groups are still catalytically active after being recycled many times.
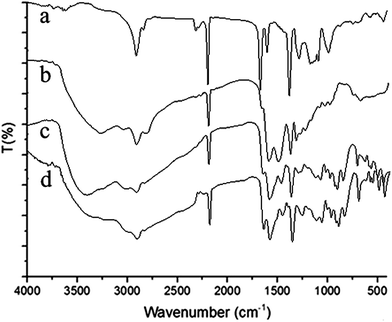 |
| Fig. 2 FTIR spectra of (a) PANF, (b) PANF-PA, (c) PANF-PA-1 and (d) PANF-PA-10. | |
Scanning electron microscope (SEM). Fig. 3 presents the SEM images of PANF, PANF-PA, PANF-PA-1, and PANF-PA-10. Compared to the original PANF (Fig. 3a), the diameter of PANF-EA body extended obviously, and the surface of the PANF-PA was thickly dotted with scarring (Fig. 3b). With the sequential utilization, the surface of the fiber samples became increasingly coarser (Fig. 3c and d). However, the overall integrity of the fiber was untouched. This observation above may be ascribed to “some changes on the PANF” and as a consequence of the modification and the catalytic processes.
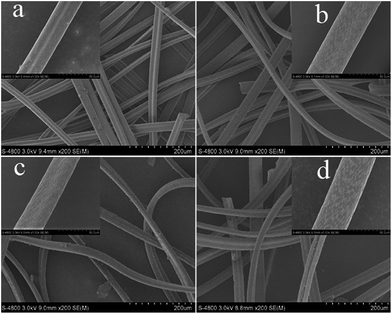 |
| Fig. 3 SEM images of (a) PANF, (b) PANF-PA, (c) PANF-PA-1 and (d) PANF-PA-10. | |
Scaleup procedures for the synthesis of iminocoumarin and sulfone catalyzed by PANF-PA
Finally, to illustrate the synthetic utility of the fiber catalyst, the large-scale reactions of the two processes were also conducted on the model reactions (see ESI†). The approaches were scaled up to gram scales, and both reactions proceeded smoothly without any extension of reaction time to afford high product yields of 96% (iminocoumarin) and, 89% and 97% (two runs for sulfone). From the combination of the above experimental results and the potential performance for being used in a fixed-bed reactor, it is obvious that the PANF-PA will be very attractive to the chemical industry.
Conclusions
In summary, a facile synthesized and reusable PANF-PA has been developed, and successfully employed as highly efficient heterogeneous base catalyst and supported phase-transfer catalyst for the synthesis of a range of iminocoumarins and sulfones in aqueous systems, respectively. The detailed characterization by EA, FTIR and SEM confirmed the deeper active principle of the PANF-PA functioned as SPTC and was recycled with excellent stability, moreover, which is very helpful for us to further design novel SPTC based on PANF. To the best of our knowledge, such a functionalized fiber has been used here for the first time in organic synthesis not only used as heterogeneous base catalyst but also as SPTC, respectively, and displays high activity and recyclability. The simple procedures can be efficiently scaled up, as well as the prominent features of PANF are very attractive to the fixed-bed reactor in the chemical industry.
Experimental
Reagents
Commercially available polyacrylonitrile fiber (PANF, the number average molecular weight of the spinning solution is 53
000 to 106
000, 93.0% acrylonitrile, 6.5% methyl acrylate and 0.4–0.5% sodium styrene sulfonate) with a length of 10 cm and a diameter of 30 ± 0.5 μm (from the Fushun Petrochemical Corporation of China) was used. All the salicylaldehydes, activated nitriles, halides, sodium p-toluenesulfinate and polyethylene polyamine, and the other reagents used were analytical grade and employed without further purification. Water was deionized.
Apparatus and instruments
The Elemental analyses (EA) were performed on a vario micro cube analyzer (Elementar). Fourier transform infrared (FTIR) spectra were obtained with an AVATAR 360 FTIR spectrometer (Thermo Nicolet), KBr disc. A scanning electron microscope (SEM) (Hitachi, model S-4800) was used to characterize the surface of the fibers. 1H NMR spectra were recorded on an AVANCE III (Bruker, 400 MHz) instrument using TMS as the internal standard. 13C NMR spectra were recorded on an AVANCE III (Bruker, 101 MHz) instrument with complete proton decoupling. Melting points were measured with a Yanagimoto MP-500 apparatus and were uncorrected.
Preparation of polyethylene polyamine functionalized polyacrylonitrile fiber (PANF-PA)
Dried PANF (3.00 g), polyethylene polyamine (30 g) and deionized water (30 mL) were introduced into a three-necked flask connected with a condenser. The mixture was refluxed (105 °C) with stirring for 24 h. The fiber was filtered out and washed repeatedly with water (60–70 °C) until the pH of the washed water was 7, and then the fiber sample was dried overnight at 60 °C under vacuum to give the PANF-PA (4.0560 g, with a weight gain of 35% and the acid exchange capacity was 4.02 mmol g−1, see ESI†).
General procedure for the synthesis of iminocoumarins
A mixture of salicylaldehyde (2 mmol), activated nitrile (2 mmol), and PANF-PA (0.025 g, 5% mol based on acid exchange capacity) in water–EtOH (10 mL, 2
:
8 v/v) was stirred under refluxing for the corresponding time in Table 1. After completion of the reaction, the fiber catalyst and the crude product were filtered out, then the former was just taken out with common tweezers and washed with ethanol or toluene (10 mL), and the later was recrystallized with the above solvent to obtain the pure product. For recycle process, the washed fiber catalyst was conducted to the next cycle without any further treatment.
General procedure for the synthesis sulfones
A mixture of halide (2 mmol), sodium p-toluenesulfinate (2.5 mmol), and PANF-PA (0.025 g, 5% mol based on acid exchange capacity) in water (10 mL) was stirred under 90 °C for 0.5 h. After completion of the reaction, the mixture was cooled to room temperature, and then the fiber catalyst and the crude product were filtered out, the fiber catalyst was just taken out with common tweezers and washed with water (20 mL), and was used again in the same substrates. The filter cake (crude product) was rinsed with the above water and then dried to obtain the pure sulfone. For recycle process, the washed fiber catalyst was conducted to the next cycle without any further treatment.
The physical data, 1H and 13C NMR data of all compounds
3-(4-Nitrophenyl)iminocoumarin (1a). Yellow solid; mp = 181–182 °C; 1H NMR (400 MHz, CDCl3) δ 8.28 (d, J = 8.0 Hz, 2H), 7.85 (d, J = 7.8 Hz, 2H), 7.70–7.14 (m, 6H). 13C NMR (101 MHz, CDCl3) δ 153.82, 147.96, 143.28, 135.56, 131.71, 130.10, 129.79, 129.08, 128.29, 124.13, 123.91, 119.84, 115.82.
3-Cyaniminocoumarin (1b). Yellow solid; mp = 164–166 °C; 1H NMR (400 MHz, DMSO) δ 8.41 (s, 1H), 7.69–7.64 (m, 1H), 7.42–7.36 (m, 2H), 7.17 (s, 1H), 5.90 (s, 1H). 13C NMR (101 MHz, DMSO) δ 162.75, 154.64, 149.76, 147.85, 147.20, 135.06, 130.43, 125.19, 118.44, 116.48.
3-Benzoyliminocoumarin (1c). Yellow solid; mp = 214–215 °C; 1H NMR (400 MHz, DMSO) δ 8.47 (s, 1H), 8.01–7.45 (m, 10H). 13C NMR (101 MHz, DMSO) δ 192.61, 158.91, 155.06, 146.22, 136.98, 134.80, 134.49, 130.70, 130.44, 129.64, 127.28, 125.80, 119.16, 117.25.
3-(4-Tolylsulfonyl)iminocoumarin (1d). Yellow solid; mp = 191 °C; 1H NMR (400 MHz, DMSO) δ 9.16 (s, 1H), 8.14–7.39 (m, 9H), 2.43 (s, 3H). 13C NMR (101 MHz, DMSO) δ 155.83, 155.54, 149.65, 145.86, 136.53, 136.39, 132.22, 130.59, 129.56, 127.83, 126.19, 118.35, 117.31, 22.03.
3-(N-Phenylcarboxamide)iminocoumarin (1e). Yellow solid; mp = 189–190 °C; 1H NMR (400 MHz, DMSO) δ 12.82 (s, 1H), 9.27 (s, 1H), 8.59 (s, 1H), 7.84 (d, J = 7.4 Hz, 1H), 7.71 (d, J = 7.7 Hz, 2H), 7.61 (s, 1H), 7.41 (t, J = 7.4 Hz, 2H), 7.34–7.25 (m, 2H), 7.16 (s, 1H). 13C NMR (101 MHz, DMSO) δ 160.57, 156.70, 154.42, 142.49, 139.15, 134.13, 131.01, 129.96, 129.59, 125.11, 124.93, 121.62, 121.08, 120.51, 119.41, 115.86.
3-(N-Benzylcarboxamide)iminocoumarin (1f). White solid; mp = 133–135 °C; 1H NMR (400 MHz, DMSO) δ 10.72 (br 1H), 9.02 (s, 1H), 8.50 (s, 1H), 7.95–6.80 (m, 9H), 4.52 (d, J = 5.4 Hz, 2H). 13C NMR (101 MHz, DMSO) δ 162.44, 158.71, 156.38, 154.38, 141.85, 139.73, 133.82, 130.82, 129.37, 128.24, 124.94, 121.14, 119.38, 115.77, 43.67.
6-Methoxy-3-(4-nitrophenyl)iminocoumarin (1g). Yellow solid; mp = 202–203 °C; 1H NMR (400 MHz, CDCl3) δ 8.26 (d, J = 8.3 Hz, 2H), 7.84 (d, J = 8.2 Hz, 2H), 7.38–6.85 (m, 5H), 3.82 (s, 3H). 13C NMR (101 MHz, CDCl3) δ 155.95, 148.19, 147.96, 143.35, 135.48, 130.12, 129.79, 129.50, 123.89, 120.12, 118.47, 116.68, 111.34, 77.36, 56.16.
3-(N-Benzylcarboxamide)-6-methoxyiminocoumarin (1h). Yellow solid; mp = 148–149 °C; 1H NMR (400 MHz, CDCl3) δ 10.76 (s, 1H), 8.46 (s, 1H), 7.62–7.28 (m, 5H), 7.04 (dd, J = 6.8, 5.8 Hz, 2H), 6.94 (s, 1H), 4.65 (d, J = 5.6 Hz, 2H), 3.83 (s, 3H). 13C NMR (101 MHz, CDCl3) δ 162.71, 158.19, 156.15, 148.66, 142.01, 138.76, 128.94, 127.92, 127.52, 121.10, 120.36, 119.53, 116.54, 111.99, 56.19, 44.13.
6-Bromo-3-(4-nitrophenyl)iminocoumarin (1i). Yellow solid; mp = 214–215 °C; 1H NMR (600 MHz, CDCl3) δ 8.29 (d, J = 8.4 Hz, 2H), 7.83 (d, J = 8.2 Hz, 2H), 7.54–6.99 (m, 5H). 13C NMR (101 MHz, DMSO) δ 157.31, 148.29, 140.98, 136.14, 130.99, 127.88, 125.29, 123.43, 119.17, 117.96, 111.07, 110.25.
3-(N-Benzylcarboxamide)-6-bromoiminocoumarin (1j). White solid; mp = 197–198 °C; 1H NMR (400 MHz, CDCl3) δ 10.60 (s, 1H), 8.41 (s, 1H), 7.75–7.49 (m, 3H), 7.35–7.28 (m, 5H), 7.00 (d, J = 8.7 Hz, 1H), 4.64 (d, J = 5.7 Hz, 2H). 13C NMR (101 MHz, CDCl3) δ 162.13, 157.18, 152.99, 140.67, 138.55, 135.52, 131.90, 128.97, 127.92, 127.60, 121.90, 120.90, 117.31, 116.83, 44.20.
1-(Benzylsulfonyl)-4-methylbenzene (2a). White solid; mp = 141–142 °C; 1H NMR (400 MHz, DMSO) δ 7.62 (d, J = 8.0 Hz, 2H), 7.42–7.32 (m, 5H), 7.18 (d, J = 6.4 Hz, 5H), 4.66 (s, 5H), 2.42 (s, 3H). 13C NMR (101 MHz, DMSO) δ 145.13, 136.51, 131.84, 130.43, 129.70, 129.17, 129.09, 128.94, 61.72, 21.95.
1-Nitro-4-(4-tolylsulfonylmethyl)benzene (2b). White solid; mp = 188–189 °C; 1H NMR (400 MHz, DMSO) δ 8.21 (d, J = 8.5 Hz, 2H), 7.65 (d, J = 8.0 Hz, 2H), 7.49–7.44 (m, 4H), 4.92 (s, 2H), 2.43 (s, 3H). 13C NMR (101 MHz, DMSO) δ 148.33, 145.55, 137.45, 136.22, 133.17, 130.61, 128.96, 124.17, 60.90, 40.45, 21.98.
1-Bromo-4-(4-tolylsulfonylmethyl)benzene (2c). White solid; mp = 170–171 °C; 1H NMR (400 MHz, DMSO) δ 7.63 (d, J = 8.0 Hz, 2H), 7.54 (d, J = 8.2 Hz, 2H), 7.44 (d, J = 8.0 Hz, 2H), 7.14 (d, J = 8.2 Hz, 2H), 4.69 (s, 2H), 2.43 (s, 3H). 13C NMR (101 MHz, DMSO) δ 145.30, 136.30, 133.90, 132.10, 130.52, 129.25, 128.95, 122.78, 60.84, 21.97.
1-Bromo-3-(4-tolylsulfonylmethyl)benzene (2d). White solid; mp = 117–119 °C; 1H NMR (400 MHz, DMSO) δ 7.65–7.18 (m, 8H), 4.71 (s, 2H), 2.44 (s, 3H). 13C NMR (101 MHz, DMSO) δ 145.37, 136.24, 134.49, 132.39, 132.03, 131.20, 130.85, 130.51, 128.98, 122.09, 60.84, 21.96.
1-Bromo-2-(4-tolylsulfonylmethyl)benzene (2e). White solid; mp = 147–148 °C; 1H NMR (400 MHz, DMSO) δ 7.63–7.32 (m, 8H), 4.79 (s, 2H), 2.44 (s, 3H). 13C NMR (101 MHz, DMSO) δ 145.57, 136.50, 134.12, 133.66, 131.49, 130.63, 129.40, 129.11, 128.55, 126.34, 61.50, 22.00.
1-Methyl-4-(4-tolylsulfonylmethyl)benzene (2f). White solid; mp = 152–153 °C; 1H NMR (400 MHz, DMSO) δ 7.62 (d, J = 8.0 Hz, 2H), 7.42 (d, J = 7.9 Hz, 2H), 7.12 (d, J = 7.8 Hz, 2H), 7.06 (d, J = 7.8 Hz, 2H), 4.60 (s, 2H), 2.42 (s, 3H), 2.30 (s, 3H). 13C NMR (101 MHz, DMSO) δ 145.08, 138.52, 136.58, 131.72, 130.44, 129.70, 128.95, 126.61, 61.41, 21.96, 21.64.
1-Fluoro-4-(4-tolylsulfonylmethyl)benzene (2g). White solid; mp = 170–171 °C; 1H NMR (400 MHz, DMSO) δ 7.62 (d, J = 8.0 Hz, 2H), 7.43 (d, J = 8.0 Hz, 2H), 7.24–7.14 (m, 4H), 4.68 (s, 2H), 2.43 (s, 3H). 13C NMR (101 MHz, DMSO) δ 164.26, 161.83, 145.24, 136.31, 133.94, 133.85, 130.49, 128.98, 126.10, 126.07, 116.17, 115.95, 60.73, 21.97.
4-Tosylacetonitrile (2h). White solid; mp = 145–146 °C; 1H NMR (400 MHz, DMSO) δ 7.91 (d, J = 8.1 Hz, 2H), 7.58 (d, J = 8.0 Hz, 2H), 5.24 (s, 2H), 2.48 (s, 3H). 13C NMR (101 MHz, DMSO) δ 146.96, 135.33, 131.10, 129.23, 113.29, 45.69, 22.09.
Acknowledgements
This work was financially supported by the National Natural Science Foundation of China (no.: 20834002 and 21306133) and Tianjin Research Program of Application Foundation and Advanced Technology (no.: 14JCYBJC22600).
Notes and references
- M. Lancaster, Green Chemistry: An Introductory Text, RSC, Cambridge, 2002 Search PubMed.
- R. A. Sheldon, Chem. Soc. Rev., 2012, 41, 1437 RSC.
- M. Benaglia, Recoverable and Recyclable Catalysts, Wiley, New York, 2009 Search PubMed.
- P. Barbaro and F. Liguori, Chem. Rev., 2009, 109, 515 CrossRef CAS PubMed.
- Y. Zhang and S. N. Riduan, Chem. Soc. Rev., 2012, 41, 2083 RSC.
- J. Lu and P. H. Toy, Chem. Rev., 2009, 109, 815 CrossRef CAS PubMed.
- H.-J. Cho, S. Jung, S. Kong, S.-J. Park, S.-M. Lee and Y.-S. Lee, Adv. Synth. Catal., 2014, 356, 1056 CrossRef CAS.
- C. Perego and R. Millini, Chem. Soc. Rev., 2013, 42, 3956 RSC.
- C. M. A. Parlett, D. W. Bruce, N. S. Hondow, M. A. Newton, A. F. Lee and K. Wilson, ChemCatChem, 2013, 5, 939 CrossRef CAS.
- D. Wang and D. Astruc, Chem. Rev., 2014, 114, 6949 CrossRef CAS PubMed.
- R. Mrówczyński, A. Nan and J. Liebscher, RSC Adv., 2014, 4, 5927 RSC.
- M. Rose, ChemCatChem, 2014, 6, 1166 CAS.
- L. M. Rossi, N. J. S. Costa, F. P. Silva and R. Wojcieszak, Green Chem., 2014, 16, 2906 RSC.
- O. M. Vatutsina, V. S. Soldatov, V. I. Sokolova, J. Johann, M. Bissen and A. Weissenbacher, React. Funct. Polym., 2007, 67, 184 CrossRef CAS PubMed.
- R. X. Liu, B. W. Zhang and H. X. Tang, React. Funct. Polym., 1999, 39, 71 CrossRef CAS.
- G. Moroi, D. Bilba and N. Bilba, Polym. Degrad. Stab., 2004, 84, 207 CrossRef CAS PubMed.
- X.-L. Shi, H. Yang, M. Tao and W. Zhang, RSC Adv., 2013, 3, 3939 RSC.
- X.-L. Shi, M. Zhang, Y. Li and W. Zhang, Green Chem., 2013, 15, 3438 RSC.
- G. Li, J. Xiao and W. Zhang, Green Chem., 2011, 13, 1828 RSC.
- X.-L. Shi, X. Xing, H. Lin and W. Zhang, Adv. Synth. Catal., 2014, 356, 2349 CrossRef CAS.
- O. A. Abd-Allah, Farmaco, 2000, 55, 641 CrossRef CAS.
- H. S. P. Rao, M. Babua and A. Desaia, RSC Adv., 2014, 4, 11064 RSC.
- T. R. Burke, B. Lim, V. E. Marquez, Z. H. Li, J. B. Bolen, I. Stefanova and I. D. Horak, J. Med. Chem., 1993, 36, 425 CrossRef CAS.
- T.-I. Kim, M. S. Jeong, S. J. Chung and Y. Kim, Chem.–Eur. J., 2010, 16, 5297 CrossRef CAS PubMed.
- D. Guo, T. Chen, D. Ye, J. Xu, H. Jiang, K. Chen, H. Wang and H. Liu, Org. Lett., 2011, 13, 2884 CrossRef CAS PubMed.
- J. Volmajer, R. Toplak, S. Bittner, I. Leban and A. M. Le Marechal, Tetrahedron Lett., 2003, 44, 2363 CrossRef CAS.
- Y. Shen, S. Cui, J. Wang, X. Chen, P. Lu and Y. Wang, Adv. Synth. Catal., 2010, 352, 1139 CrossRef CAS.
- C. Arani and V. F. Valery, Chem. Rev., 2009, 109, 725 CrossRef PubMed.
- J. Li, J. Cao, J. Wei, X. Shi, L. Zhang, J. Feng and Z. Chen, Eur. J. Org. Chem., 2011, 19, 229 CrossRef.
- N. S. Simpkins, Sulfones in Organic Synthesis, Tetrahedron Organic Chemistry Series, Pergamon Press, New York, 1993 Search PubMed.
- B. T. V. Srinivas, V. S. Rawat, K. Konda and B. Sreedhar, Adv. Synth. Catal., 2014, 356, 805 CrossRef CAS.
- A. Akalah and D. C. Sherring, Chem. Rev., 1981, 81, 557 CrossRef.
Footnote |
† Electronic supplementary information (ESI) available: The acid exchange capacity of PANF-PA, scale-up experimental procedures and NMR spectra of all compounds. See DOI: 10.1039/c4ra12069h |
|
This journal is © The Royal Society of Chemistry 2014 |