DOI:
10.1039/C4RA11353E
(Paper)
RSC Adv., 2014,
4, 52235-52240
Reversible sensing of aqueous mercury using a rhodamine-appended polyterthiophene network on indium tin oxide substrates†
Received
27th September 2014
, Accepted 7th October 2014
First published on 8th October 2014
Abstract
Herein we report the synthesis, and optical and electrochemical properties of a rhodamine-appended polyterthiophene network thin film which demonstrates ion selective potentiometric, chromic and fluorescent responses. The rhodamine-appended terthiophene monomer (RhoT) was electropolymerized and deposited on an ITO electrode. Ion selective potentiometric studies have shown that the potentials of the conducting polymer films decreased upon adding Hg2+ because interactions of Hg2+ with the rhodamine-appended conducting polymer film may increase charge carrier transport properties on a conjugated polymer through rhodamine-bound Hg2+, reduce the doping states by interfering with ions through ion–ion interactions, and perturb the π-extended conjugated polymer through π–π interactions. Moreover, the lower detection limit of the ultrathin film sensor toward Hg2+ (0.10 μM) was less than that obtained from RhoT (1.34 μM), and the response time was less than 30 seconds. Reusability was evaluated by repeating dipping and rinsing cycles in aqueous Hg2+ and EDTA solutions. This approach may provide an easily measurable and inherently sensitive method for Hg2+ ion detection in environmental and biological applications.
Introduction
Receptor-based conducting polymer networks (CPN) are a class of materials that have been successfully utilized in the development of molecular materials. The reversible binding of an analyte by such a CPN can produce perturbations in the chemical structure, oxidation state, and/or solid state ordering of the system resulting in voltammetric, ionochromic, and chemoresistive responses.1,2 This approach is attractive because materials at the nanometer scale often exhibit unique physical and chemical properties that can be utilized to improve sensor performances. For example, Roncali and co-workers reported a series of macrocyclic oxyethylene-bridged oligo- and polythiophenes in which the oxyethylene groups were attached to the internal β-position of the terminal thiophene units through sulfide linkages.3,4 Such molecules showed conformational transitions in the presence of Ba2+, Sr2+ and Pb2+.3–5 The direct attachment of the calixarene unit to a conjugated polymer has also been reported by Swager and co-workers and has resulted in rigid zigzag and molecular wire structures.6,7 Hexahomotriazacalix[3]arene-carbazole has been successfully developed as a conjugated polymer network ultra-thin films for Zn2+.8
Many recent developments have shown that rhodamine spirolactam is a promising structural scaffold for the design of selective chemosensors. It can undergo a structural change from a spirolactam to an open ring amide by cations induced activation of a carbonyl group in the spirolactone or spirolactam moiety, resulting in a magenta-coloured highly fluorescence compound.9,10 Rhodamine-based chemosensors have received ever-increasing interest for cations and other analytes such as Pb2+, Cu2+, Hg2+, Fe3+, Cr3+, NO, and OCl−.11–18 In our previous works, we successfully designed and synthesized rhodamine-based chemosensors for cations and anions. In 2010, we synthesized fluorescence chemosensors for anions, cations and ditopic receptors utilizing rhodamine B as the fluorophore.19 In 2011, we reported the rhodamine-based reversible chemosensors which could bind Hg2+ with a detectable change in colour. Upon the addition of Hg2+, an overall emission change of 350-fold was observed, and the selectivity was calculated to be 300 times higher than Cu2+.20 In 2013, we investigated a selective detection of Au3+ by using rhodamine-based modified polyacrylic acid (PAA) sensors.21 Polymeric sensors were simply prepared by amidation reactions between PAA and various mole ratios of rhodamine. It was found that polymeric sensor PAA-Rho2 exhibited the highest selectivity and sensitivity responsive colorimetric and fluorescence Au3+-specific sensor over other metal ions. Moreover, a FeNPs-based polymeric sensor PAA-Rho2-FeNPs was designed and synthesized. The Au3+ chelation-induced aggregation of FeNPs was observed and restored using the EDTA solution.
A terthiophene functionalized rhodamine (RhoT) containing a terthiophene group (energy donor) and a rhodamine group (energy acceptor) to generate FRET-based chemosensor has just been reported and applied as gold nano particles based-chemosensor (AuNPs-RhoT) via simultaneous reduction of AuCl3 with oxidative polymerization of terthiophene functionalized rhodamine (RhoT).22 The chemosensors showed the sensitive and selective detection of Hg2+ in aqueous solution over other metal ions. However, these chemosensors are chemically irreversible, cannot detect a time dependent signal in real time and have rather high detection limits (0.32 μM).
This paper focuses on the synthesis of molecular fluorescent monomer which containing a terthiophene group (easier to be oxidized than the thiophene, carbazole and pyrrole monomers because of lower oxidation potential stemming from the extended π-electron)23 and a rhodamine group (fluorogenic, chromogenic and cation-probe parts). To fabricate sensors, terthiophene units were turned into a π-conjugated polyterthiophene by electropolymerization to produce a novel colorimetric/electrochemical-based ratiometric polymeric sensor for cation sensing (Scheme 1). A multiple-site model of all hybrid materials has been used to increase the selectivity and sensitivity of the measurement. We believe that the relatively easy manipulation of rhodamine derivatives should interest many organic chemists, analytical chemists and biochemists involving in sensor technology for monitoring heavy metals.
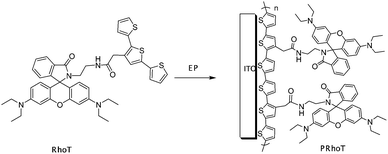 |
| Scheme 1 Fabrication of conjugated polymer-based (PRhoT) chemosensors from the FRET-based (RhoT) molecular sensor. | |
Results and discussion
Synthesis and binding ability of rhodamine-appended terthiophene (RhoT) monomer
RhoT was synthesized in a good yield by an amidation reaction between 2-(2,5-di(thiophen-2-yl)thiophen-3-yl)acetic acid and rhodamine–ethylenediamine in the presence of dicyclohexylcarbodiimide/dimethylaminopyridine (DCC/DMAP) as coupling reagent under N2 at reflux for 3 days in THF.22 RhoT displayed a highly selective fluorescence enhancement and a colour change toward Hg2+ over other metals in DMSO, and could be used as a potential Hg2+-selective chemosensor in the presence of physiologically important metal ions by fluorescence resonance energy transfer (FRET). In addition, the competition experiment was also carried out by adding Hg2+ to the solution of RhoT in the presence of other metal ions. The results shown in Fig. 1 indicate that the sensing of Hg2+ by RhoT is hardly affected by other common interfering ions.
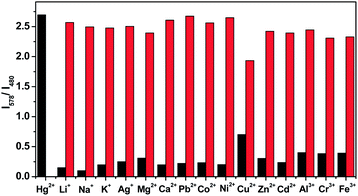 |
| Fig. 1 Fluorescence enhancement response of RhoT (10 μM) in 0.01 mol L−1 of TBAPF6 in DMSO to 10 μM of various cations (the black bar portion) and to the mixture of 10 μM different metal ions with 10 μM of Hg2+ (the red bar portion). | |
Electropolymerization of RhoT
To fabricate a sensor device of RhoT, electropolymerization of RhoT and was carried out by cyclic voltammetry (CV) using indium tin oxide (ITO) as a working electrode, platinum as a counter electrode, and Ag/AgCl as a reference electrode in a three-electrode cell. RhoT was electropolymerized (cross-linked) as films using 1.0 mM concentration of RhoT in methylene chloride containing 0.1 M tetrabutylammoniumhexafluorophosphate as a supporting electrolyte, where the potential was scanned at 100 mV s−1 from 0.0 to +1.3 V. As shown in Fig. 2, the monomer was oxidized at +0.98 V during the first anodic scan, followed by the deposition of the polymer film on the electrode surface immediately. Moreover, a clear decrease in the reduction peaks during deposition is observed. The change in oxidation current per cycle is measured during electropolymerization and a general increase in oxidation current with each cycle over time is observed, indicating a continuous deposition on the substrate (inset of Fig. 2). This suggests that there is an optimal conformational freedom required to allow extended conjugation during cross-linking for these chemosensor.24 The simultaneous appearance of the new onset peaks around 0.82 V, which corresponds to the formation of the polythiophene backbone.25 A linear relationship between peak current and scan rate is observed for all these films, suggesting that the redox-active polymer is attached to the electrode with a typical Fickian diffusion behavior as defined by the Cottrell equation.26 Furthermore, as observed from a mirror placed beneath the working electrode, a reddish brown precipitate has been formed on the electrode surface. After polymerization of RhoT, the electrode coated with PRhoT is washed with dichloromethane to remove the excess monomer and used as ultrathin films for specific cation sensing.
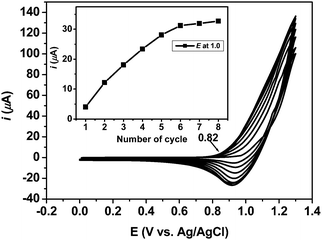 |
| Fig. 2 Cyclic voltammograms of the electrochemical cross-linking/deposition on ITO substrate of RhoT at a scan rate of 100 mV s−1, 8 cycles. | |
Morphology studies
The morphology after electropolymerization on the ITO was characterized by FESEM and AFM as illustrated in Fig. 3. RhoT was electropolymerized for 8 cycles at a range of 0–1.0 V and 0.0–1.3 V with a scan rate of 100 mV s−1 in 0.1 M TBAPF6/anhydrous CH2Cl2 as electrolyte (WE, ITO-coated slide; CE, Pt wire; RE, Ag/AgCl wire). The result showed that the small quantity was deposited with applied potentials from 0 to 1.0 V (Fig. S1 in the ESI†). However, in the case of higher applied potential (up to 1.3 V), both FESEM and AFM images in Fig. 3 showed that the ultrathin film deposited on ITO as a rougher and patchy film.
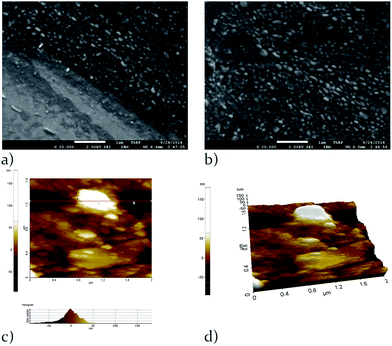 |
| Fig. 3 FESEM images of (a) the boundary of ITO and the film of PRhoT on ITO (b) the film of PRhoT on ITO (c) 2D and (d) 3D AFM images of the film of PRhoT on ITO. PRhoT was electropolymerized at 50 mV s−1 in TBAPF6/CH2Cl2 electrolyte (WE, ITO; CE, Pt wire; RE, Ag/AgCl wire) on the ITO substrate at the range of 0.0–1.3 V. | |
Spectroelectrochemistry
Spectroelectrochemical measurements were performed using a previously described setup.27 The spectroelectrochemical measurement of PRhoT was performed to investigate the cross-linking polymerization process in situ and the presence of polaronic states associated with the formation of a more conjugated polymer. The in situ UV-vis absorption spectra of RhoT were measured simultaneously with electropolymerization in a 0.1 M TBAPF6–CH2Cl2 solution as electrolyte (WE, ITO; CE, Pt wire; RE, Ag/AgCl) at a scan rate of 100 mV s−1. The absorption spectrum of PRhoT in Fig. 4 shows the absorption band at 320 nm due to π to π* electron transition of polyterthiophene.28 The broad band centered at 800 nm could be assigned to the polaronic and bipolaronic bands originating from the formation of the conjugated polyterthiophene species and their complexed ion redox couple with hexafluorophosphate ions. The bathochromic shift from 320 nm to 343 nm and ring-opened characteristic band at 556 nm were observed upon the addition of Hg2+ (Fig. 4). Moreover, it can be inferred that incorporation of Hg2+ into the binding sites forces a rotation about the terthiophene axis resulting in increasing π-orbital overlap.29
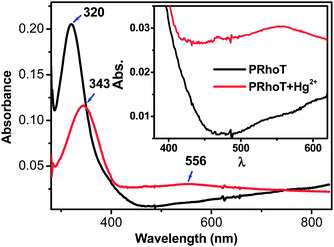 |
| Fig. 4 UV-vis absorption spectrum of PRhoT films upon the addition of Hg2+ with the concentration 10−6 M on ITO substrates. | |
Potentiometric sensing
The sensing selectivity and sensitivity studies were investigated by open-circuit potentiometry. Changes in potential (ΔE) were recorded simultaneously as a function of time at constant zero-current voltage. We also monitored the redox state of the polymeric thin film since it may be influenced by either introducing an electric charge or adding a cation which interfered with the redox equilibrium. The selectivity studies by potentiometry were performed using the electrochemically cross-linked PRhoT film on ITO against 10−6 M of the various cations (0.01 M TBACl as supporting electrolyte). Fig. 5a showed the change in ΔE of PRhoT film in the presence and absence of 10−6 M of various cations. As expected, the highest selectivity (ΔE = −0.05 V) was observed upon addition of Hg2+ as compared to other cations. This may be due to the fit between Hg2+ and the receptor size, steric effect from the polymer backbone and compatible hard–soft acid and base pair of Hg2+ and donor atoms. These results are consistent with our previous observations that two pseudo-azacrown rhodamine based chemosensors can be produced a specific hole and distance with Hg2+.20 In order to evaluate the sensing phenomena of the PRhoT thin film, we varied the concentration of Hg2+ in the testing system (Fig. 5b). At the lowest concentration (10−7 M), the ΔE was suddenly decreased in the first 100 s, followed by a slight increase until reaching a steady state. The same results were observed after adding 10−6 and 10−5 M Hg2+. This indicated that the decrease of potential at low concentration level of Hg2+ over time was observed due to the complexation of Hg2+ with rhodamine moieties through cation-dipole interactions. On the other hand, the increasing of ΔE was evaluated in the first 100 s at higher concentration (10−4 M) referred to the occupied binding site of the rhodamine moieties on PRhoT thin film. Then, free cations may complex with other species in the polymer backbone.23
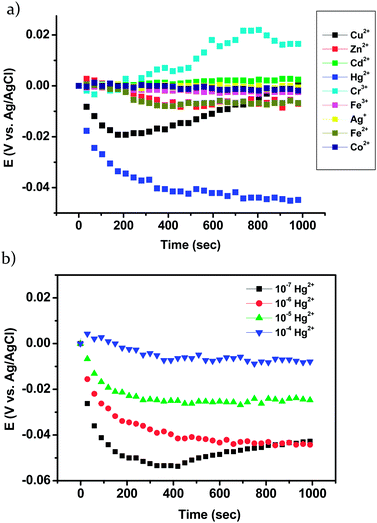 |
| Fig. 5 Potentiometric profiles of PRhoT on ITO in 0.01 M TBACl aqueous solution: (a) various cation analytes and (b) various concentrations of Hg2+. Plotted data are within 5% deviation from several measurements. | |
Moreover, colour (pale yellow to pink) and fluorescent changes are observed on the regions of PRhoT on ITO substrate after exposure to the solution of Hg2+ for 3 min (Fig. 6), resulting from a ring-opening form of the spirolactam.
 |
| Fig. 6 Colour (A and D) and fluorescence changes (a and d) of PRhoT in the absent and presence of 10 μM of Hg2+; (A and a) PRhoT only and (D and d) PRhoT + Hg2+. | |
The fluorescence Hg2+ image disappears and the non-fluorescence and colourless of the Hg2+-exposed region of the PRhoT thin film is restored. Infrared spectroscopy (Fig. 7) is also employed to confirm the binding of the carbonyl group on rhodamine moieties of PRhoT with Hg2+ ions and the reversible process. It is clearly observed that upon addition of Hg2+, the carbonyl stretching band of PRhoT at 1731 cm−1 was changed to the lower number (1706 cm−1) and turned back to 1731 cm−1 after treatment with an aqueous EDTA solution under basic conditions.20,21 The fluorescence change is reproducible over several cycles of exposure recovery (Fig. 8). Although some variations in the intensities of the emission minima and maxima are observed (±12%), these changes do not affect the overall hybrid sensor performance which might be due to wettability properties of the hybrid sensor.30 In addition, according to changes in signaling (ΔE and fluorescence emission) upon adding various Hg2+ concentration, the limit of detection31,32 of PRhoT for Hg2+is calculated to be 0.10 μM, lower than that observed using RhoT (1.34 μM) and the detection time is less than 30 seconds. As compared to RhoT, PRhoT gave a lower detection limit and a higher sensitivity toward Hg2+. In addition, our PRhoT sensor provides a better detection limit (DL) toward Hg2+and can be used in water as compared to previous reports as listed in Table 1tbl1.33–35
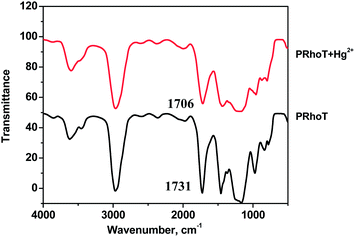 |
| Fig. 7 FT-IR spectra of PRhoT and PRhoT·Hg2+. | |
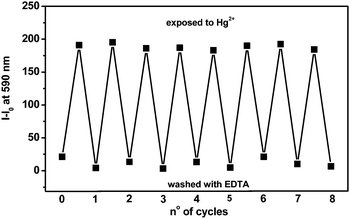 |
| Fig. 8 Changes in the fluorescent (measured at 580 nm) of the PRhoT during the cyclic detection/reactivation processes. | |
Table 1 Selected examples of Hg2+ sensors with rhodamine-based sensor and synthetic receptors immobilized into conducting polymer
Polymer or rhodamine-based |
Receptor |
Working solvent |
DL (M) |
Rhodamine-appended polyterthiophene |
Rhodamine |
H2O |
0.10 × 10−6 |
Polymer-triazole33 |
Triazole |
CH3CN |
4.6 × 10−7 |
Polypyrrolel/polyantimonic acid34 |
Polyantimonic acid |
H2O |
5.0 × 10−5 |
Rhodamine–AuNPs22 |
Rhodamine |
DMSO + H2O |
0.32 × 10−6 |
Rhodamine–terthiophene22 |
Rhodamine |
DMSO |
1.34 × 10−6 |
Phenylthiosemicarbazide rhodamine 6G derivative35 |
Rhodamine |
H2O |
0.30 × 10−6 |
Conclusions
We have designed and synthesized an ion-responsive sensory material based on a rhodamine-appended polyterthiophene by electrochemical crosslinking of monomer RhoT. The incorporation of rhodamine moiety as a neutral cation-binding receptor into a conjugated polyterthiophene network facilitates high selectivity and sensitivity for Hg2+. The conducting polymeric sensors, PRhoT, showed a highest selectivity (ΔE = −0.05 V) toward Hg2+ over other cations. After placing the sensor into the EDTA solution, the free sensor could be restored. The limit of detection of PRhoT for Hg2+ was 0.10 μM and the detection time was less than 30 seconds. We believe that, this approach may provide an easily handled and inherently sensitive method for Hg2+ detection in environmental and biological applications.
Experimental
Chemical and methods
All reagents were standard analytical grade. Rhodamine was purchased from Aldrich. DCC, DMAP, ethylenediamine, MeOH were from Merck and used without further purification. Commercial grade solvents, such as acetone, hexane, dichloromethane, methanol and ethyl acetate, were distilled before use. DMF was dried over CaH2 and freshly distilled under nitrogen prior to use. Acetonitrile and dichloromethane for set up the reaction were dried over calcium hydride and freshly distilled under nitrogen atmosphere prior to use. Tetrahydrofuran was dried using sodium benzophenoneketyl and immediately distilled under nitrogen before use. N-(rhodamineB)lactam-ethylenediamine-2-(2,5-di(thiophen-2-yl)thiophen-3-yl)acetate (RhoT) was synthesized using the previous published procedure.22 The solutions of metal ions were prepared from Cu(NO3)2·3H2O, Zn(NO3)2·3H2O, Cd(NO3)2·4H2O, Hg(Cl)2, CrCl3·6H2O, FeCl3·6H2O, respectively, and were dissolved in deionized water. Aqueous TBACl (0.1 mol L−1) solution was used as supporting electrolyte to maintain the ionic strength of all solutions in the experiments.
Instrumentation
UV-vis absorption measurements were performed on a Perkin Elmer Lambda 25 UV/VIS spectrophotometer. Fluorescence spectra were recorded using a Perkin Elmer luminescence spectrophotometer LS50B. Infrared spectra were obtained on a Nicolet Impact 410 using KBr pellets.
Cyclic voltammetry (CV) experiments were carried out on a 910 PSTAT mini Metrohm with a modified ITO substrate as the working electrode coupled with a Pt plate counter and Ag/AgCl reference electrode.
Atomic force microscopy (AFM) imaging was examined in ambient conditions with a Park NX10 (Park Systems Corp.) in the Tapping mode (AAC mode).
FESEM images were obtained from a field emission scanning electron microscope (JSM-7001F) using an accelerated voltage of 2 kV and a working distance of 6.3 mm and operating in the secondary electron image (SEI) mode. The samples (PRhoT on ITO glass) were sputtered with gold (Quorum, Q150RS) using sputter current of 10 mA and sputter time of 45 s before taking images.
Synthesis of N-(rhodamine B)lactam-ethylenediamine-2-(2,5-di(thiophen-2-yl)thiophen-3-yl)acetate (RhoT).22
RhoT was synthesized from the reaction of compound Rho (0.20 g, 0.65 mmol) and 2-(2,5-di(thiophen-2-yl)thiophen-3-yl)acetic acid (0.15 g, 0.82 mmol) in the presence of dicyclohexylcarbodiimide (DCC) (0.20 g, 0.98 mmol) and p-(dimethylamino)pyridine (DMAP) (0.12 g, 0.98 mmol) in 20 mL of anhydrous THF. After purification by column chromatography on silica gel (CH2Cl2/n-hexane (1
:
1)), RhoT was obtained as an orange crystal (0.13 g, 82% yield). 1H NMR (400 MHz, CDCl3) 7.71 (d, J = 7.6 Hz, 1H, ArH), 7.35–7.26 (m, 3H, ArH), 7.15–7.11 (m, 2H, ArH), 7.03–7.01 (m, 2H, ArH), 6.97–6.90 (m, 3H, ArH), 6.66 (bs, 1H, CH2NHCO), 6.28–6.26 (m, 1H, ArH), 6.23 (s, 1H, ArH), 6.21 (s, 1H, ArH), 6.11–6.07 (m, 2H, ArH), 3.98–3.92 (m, 2H, ArCH2CO), 3.52 (s, 1H, NCH2CH2), 3.46–3.36 (m, 2H, NCH2CH2N), 3.20 (q, J = 7.6 Hz, 8H, NCH2CH3), 2.87–2.84 (m, 1H, NCH2CH2N), 1.05 (t, J = 7.2 Hz, 12H, NCH2CH3). IR (KBr) ν 3385, 3240, 2971, 1789, 1683, 1526, 1511, 1312, 1277, 1202, 1103, 779 and 693 cm−1. MS (MALDI-TOF) calcd for [C44H44N4O3S3]+: m/z 772.26. Found: m/z 773.388 [M + H]+. Anal. calcd for C44H44N4O3S3: C, 68.36; H, 5.74; N, 7.25. Found: C, 68.36; H, 5.64; N, 7.28.
Electrochemical synthesis of cross-linked polymers (PRhoT)
The precursor polymers were synthesized using the cyclic voltammetry (CV) technique. In a three-electrode cell, 0.10 M tetra-butylammoniumhexafluorophosphate (TBAPF6) was used as a supporting electrolyte along with 1 mM RhoT dissolved in anhydrous methylene chloride in a cell. The electropolymerization of the precursor polymers was performed by sweeping the voltage at a scan rate of 100 mV s−1 from 0 to 1.3 V using Ag/AgCl as a reference electrode and platinum as a counter electrode. The ITO was used as a working electrode and as a substrate. UV-vis (nm): 320 (λπ–π*), 600–900 (λπ–polarlon, λπ–π, λpolarlon–π*). FT-IR (cm−1): 1706 (νCO), 1440, 1350 (νArC–C).
Sensitivity and selectivity studies of PRhoT by using potentiometry
PRhoT was studied as a sensor using 0.01 M TBACl as electrolyte. Using Teflon cell, TBACl of 0.01 M was injected, until potential signal was kept constant. To study sensitivity and selectivity of the polymer, different concentrations of cations was held constant for 1000 s. The change in potential (ΔE), [(ΔE) = observed potential (E0) − initial potential (Ei)] was recorded simultaneously as a function of time.
Acknowledgements
The authors gratefully acknowledge funding from Mahasarakham University and the Thailand Research Fund (RTA5380003) and Center of Excellence for Innovation in Chemistry (PERCH-CIC), Office of the Higher Education Commission, Ministry of Education. PSTAT mini was purchased with a loan fund from Faculty of Science MSU. We thank Professor Sojiphong Chatraphorn of Thailand Center of Excellence in Physics (ThEP), Department of Physics, Faculty of Science, Chulalongkorn University for FESEM images.
Notes and references
- D. T. McQuade, A. E. Pullen and T. M. Swager, Chem. Rev., 2000, 100, 2537–2574 CrossRef CAS PubMed.
- G. D. Joly and T. M. Swager, Chem. Rev., 2007, 107, 1339–1386 CrossRef PubMed.
- D. Demeter, P. Blanchard, I. Grosu and J. Roncali, Electrochem. Commun., 2007, 9, 1587–1591 CrossRef CAS PubMed.
- D. Demeter, P. Blanchard, M. Allain, I. Grosu and J. Roncali, J. Org. Chem., 2007, 72, 5285–5290 CrossRef CAS PubMed.
- B. Jousselme, P. Blanchard, E. Levillain, J. Delaunay, M. Allain and P. Richomme, J. Am. Chem. Soc., 2003, 125, 1363–1370 CrossRef CAS PubMed.
- J. H. Wosnick and T. M. Swager, Chem. Commun., 2004, 2744–2745 RSC.
- H. Yu, B. Xu and T. M. Swager, J. Am. Chem. Soc., 2003, 125, 1142–1143 CrossRef CAS PubMed.
- C. Kaewtong, G. Jiang, Y. Park, A. Baba, T. Fulghum, B. Pulpoka and R. Advincula, Chem. Mater., 2008, 20, 4915–4924 CrossRef CAS.
- H. N. Kim, M. H. Lee, H. J. Kim, J. S. Kim and J. Yoon, Chem. Soc. Rev., 2008, 37, 1465–1472 RSC.
- L. Yuan, W. Lin, K. Zheng, L. He and W. Huang, Chem. Soc. Rev., 2013, 42, 622–661 RSC.
- C.-Y. Li, Y. Zhou, Y.-F. Li, X.-F. Kong, C.-X. Zou and C. Weng, Anal. Chim. Acta, 2013, 774, 79–84 CrossRef CAS PubMed.
- A. Sikdar, S. Roy, K. Haldar, S. Sarkar and S. S. Panja, J. Fluoresc., 2013, 23, 495–501 CrossRef CAS PubMed.
- J. Wu, I. Hwang, K. Kim and J. Kim, Org. Lett., 2007, 9, 907–910 CrossRef CAS PubMed.
- Z. Dong, X. Tian, Y. Chen, J. Hou, Y. Guo, J. Sun and J. Ma, Dyes Pigm., 2013, 97, 324–329 CrossRef CAS PubMed.
- H. Ouyang, Y. Gao and Y. Yuan, Tetrahedron Lett., 2013, 54, 2964–2966 CrossRef CAS PubMed.
- N. R. Chereddy, S. Thennarasu and A. B. Mandal, Dalton Trans., 2012, 41, 11753–11759 RSC.
- M. J. Jou, X. Chen, K. M. K. Swamy, H. N. Kim, H.-J. Kim, S.-G. Lee and J. Yoon, Chem. Commun., 2009, 7218–7220 CAS.
- X. Chen, K.-A. Lee, E.-M. Ha, K. M. Lee, Y. Y. Seo, H. K. Choi, H. N. Kim, M. J. Kim, C.-S. Cho, S. Y. Lee and W. J. Lee, Chem. Commun., 2011, 4373–4375 RSC.
- C. Kaewtong, J. Noiseephum, Y. Uppa, N. Morakot, N. Morakot, B. Wanno, T. Tuntulani and B. Pulpoka, New J. Chem., 2010, 34, 1104–1108 RSC.
- C. Kaewtong, B. Wanno, Y. Uppa, N. Morakot, B. Pulpoka and T. Tuntulani, Dalton Trans., 2011, 40, 12578–12583 RSC.
- N. Niamsa, C. Kaewtong, V. Srinonmuang, B. Wanno, B. Pulpoka and T. Tuntulani, Polym. Chem., 2013, 4, 3039–3046 RSC.
- C. Kaewtong, N. Niamsa, B. Wanno, N. Morakot, B. Pulpoka and T. Tuntulani, New J. Chem., 2014, 38, 3831–3839 RSC.
- J. Chen, A. K. Burrell, W. M. Campbell, D. L. Officer, C. O. Too and G. G. Wallace, Electrochim. Acta, 2004, 49, 329–337 CrossRef CAS PubMed.
- M. Ponnapati, J. Felipe, J. Y. Park, J. Vargas and R. Advincula, Macromolecules, 2010, 43, 10414–10421 CrossRef.
- M. Schäferling and P. Bäuerle, J. Mater. Chem., 2004, 14, 1132–1141 RSC.
- P. Taranekar, T. Fulghum, A. Baba, D. Patton and R. Advincula, Langmuir, 2007, 23, 908–917 CrossRef CAS PubMed.
- F. Tran-Van and C. Chevrot, Electrochim. Acta, 2002, 47, 2927–2936 CrossRef CAS.
- C. Kaewtong, G. Jiang, R. Ponnapati, B. Pulpoka and R. Advincula, Soft Matter, 2010, 6, 5316–5319 RSC.
- K. Sugiyasu and T. M. Swager, Bull. Chem. Soc. Jpn., 2007, 80, 2074–2083 CrossRef CAS.
- F. Lupo, C. Capici, G. Gattuso, A. Notti, M. F. Parisi, A. Pappalardo, S. Pappalardo and A. Gulino, Chem. Mater., 2010, 22, 2829–2834 CrossRef CAS.
- L. Chen, W. Lu, X. Wang and L. Chen, Sens. Actuators, B, 2013, 182, 482–488 CrossRef CAS PubMed.
- Z. Yuan, N. Cai, Y. Du, Y. He and E. S. Yeung, Anal. Chem., 2014, 86, 419–426 CrossRef CAS PubMed.
- X. Huang, J. Meng, Y. Dong, Y. Cheng and C. Zhu, Polymer, 2010, 51, 3064–3067 CrossRef CAS PubMed.
- A. A. Khan and M. M. Alam, Anal. Chim. Acta, 2004, 504, 253–264 CrossRef CAS PubMed.
- F. J. Orriach-Fernández, A. Medina-Castillo, J. F. Fernández-Sánchez, A. Muñoz de la Peña and A. Fernández-Gutiérrez, Anal. Methods, 2013, 5, 6642–6648 RSC.
Footnote |
† Electronic supplementary information (ESI) available. See DOI: 10.1039/c4ra11353e |
|
This journal is © The Royal Society of Chemistry 2014 |