DOI:
10.1039/C4RA10006A
(Paper)
RSC Adv., 2014,
4, 53711-53721
Graphene oxide–aluminium oxyhydroxide interaction and its application for the effective adsorption of fluoride
Received
8th September 2014
, Accepted 9th October 2014
First published on 9th October 2014
Abstract
A novel aluminium oxy hydroxide [Al–O(OH)] modified graphene oxide was prepared by a chemical precipitation method wherein Al3+ ions could interact effectively with the different functional groups of graphene oxide (GO). The prepared (GO–Al–O(OH) adsorbent was tested for the effective defluoridation of water. The Al3+ modified graphene oxide adsorbent was characterized using FT-IR, FT-Raman, SEM-EDS, XRD and XPS studies. The thermodynamically feasible adsorption is supported by the pseudo second order kinetics and a high Langmuir maximum adsorption capacity (51.42 mg g−1) for the GO–Al–O(OH) adsorbent. Furthermore, we could treat 2.0 L of 5.0 mg L−1 fluoride ion solution to bring the level within the permissible limits and the regeneration of the adsorbent was done using ammonium hydroxide.
Introduction
Fluoride, as an ingredient of drinking water, has beneficial effects when present within the permissible limits in order to foster healthy bone formation. However, as per WHO guidelines1 when the concentration of fluoride exceeds the threshold limit of 1.5 mg L−1, adverse effects such as dental and skeletal fluorosis are observed.2 Excess fluoride could also lead to osteoporosis, brittle bones and thyroid disorders.3 Hence, it is vital to develop effective methods to reduce the fluoride levels to the acceptable limit. Variety of adsorbents are known for the remediation of fluoride from drinking water.4 Among these, carbonaceous materials such as carbon nanotubes5 and activated carbon6 are quite effective for facile defluoridation of water by virtue of their high specific surface area, surface functional groups and adequate pore size distribution.
Graphene oxide has emerged as an attractive member of carbon family in view of its high surface area and presence of various functional groups (hydroxyl, epoxy groups and carboxylic).7,8 Graphene9 has also proven to be an effective adsorbent for the adsorption of F− ion in aqueous solution with an adsorption capacity of 17.65 mg g−1. A novel metalloporphyrin grafted-graphene oxide10 has been utilized as a sensor for F− ion in aqueous solution and hence it is possible that suitable modification of graphene oxide could also assist in the adsorption of F− ion with good adsorption capacity. The incorporation of Zr-hydroxide into graphene oxide can significantly increase the adsorption capacity of negatively charged ionic pollutants.11,12 Furthermore, manganese oxide coated graphene oxide (MOGO)13 studied recently for fluoride ion adsorption as a new hybrid material shows an adsorption capacity of 11.63 mg g−1. Basic aluminum sulfate@graphene hydrogel has been reported to possess an adsorption capacity of 33.4 mg g−1 at pH 7.2 towards F− ion adsorption.14
A careful inspection of the literature shows that graphene oxide has not been explored to its full potential for enhanced fluoride adsorption. Taking advantage of the inherent properties of graphene oxide we embarked on preparing aluminium oxy hydroxide [Al–O(OH)] incorporated graphene oxide for potential application towards defluoridation. GO–Al(OH)3 composites are fairly easy to synthesize15 and chitosan reinforced with nano AlOOH composite16 and chitosan–GO hydrogel composite17 show good capacity to purify water free from microbial contaminants and toxic dyes respectively. To the best of our knowledge, there are no reports on the use of [Al–O(OH)] incorporated graphene oxide for defluoridation. Since, Al3+ is a hard acid, and F− ion is a typical hard base, it is envisaged that the interaction between these two oppositely charged ionic species onto the GO surface would enhance the removal of fluoride. The objective of this work was to prepare the [Al–O(OH)] incorporated graphene oxide and to explore the mechanism of F− ion adsorption onto the adsorbent surface.
Experimental details
Materials
Graphite with a mean particle size (<20 μm) was procured from Sigma Aldrich. Orthophosphoric acid (H3PO4, 85%), hydrogen peroxide (H2O2, 30%), potassium permanganate (KMnO4), aluminum sulfate hydrate (Al2 (SO4)3·16H2O), sodium hydroxide (NaOH) and sodium fluoride (NaF) were purchased from S.d. fine Chemicals, India. TISAB II buffer solution (HI 4010-00) was obtained from Hanna instruments, USA. Sulfuric acid (H2SO4, 96.0%), hydrochloric acid (HCl, 35.0%) and ethanol (CH3CH2OH, 95.0%) were procured from Himedia laboratories, India.
Preparation of graphene oxide from graphite
An improved method was used for the synthesis of graphene oxide as reported earlier.18 About 1.5 g of graphite powder was taken and gradually added to 9
:
1 mixture of concentrated H2SO4/H3PO4. The reaction was gently warmed to 35–40 °C followed by heating to 60 °C and constantly stirred for 12 h. The above reaction mixture was cooled to room temperature and slowly poured in ice cold peroxide solution, where the brown colour entirely turned to yellow. Subsequently, the mixture was centrifuged at 4000 rpm for 4 h and the supernatant was decanted away. The solid material obtained after centrifugation was thoroughly washed with 200 mL of water followed by 200 mL of 30% HCl and 200 mL of ethanol. After each wash, the filtrate was centrifuged at 4000 rpm for 2 h and the supernatant was discarded. The solid material was kept for drying at 30 °C in vacuum oven (Biotechnics, India) for 48 h.
Preparation of [Al–O(OH)] incorporated graphene oxide
About 0.2 g of graphene oxide was dispersed in 100 mL of Milli-Q water. The exfoliation was done by sonication (Ultrasonic bath, Biotechnics, India) for 1 h at a 15 min intermittent time interval. Subsequently, 6.30 g of aluminium sulfate was added to the exfoliated graphene oxide solution and stirred for 2 h. The pH was adjusted to 7.0 using 1.0 mol L−1 sodium hydroxide solution and stirred for an extended time interval of 16 h. The reaction mixture was filtered, washed with Millipore water several times to remove sulfate ions (tested using BaCl2 solution) thoroughly. The adsorbent was dried overnight at 30 °C in a vacuum oven.
Instrumentation
A 1 L volume of 1000 mg L−1 fluoride ion stock solution was prepared using the appropriate amount of sodium fluoride and stored in a polypropylene bottle (1 L). Fluoride ion selective electrode (Metrohm 867 pH/ion analysis, fluoride ISE 6.0502.150, Switzerland) was used to measure the concentration levels of fluoride before and after adsorption. The instrument was calibrated with 1.0, 2.0 and 5.0 mg L−1 fluoride ion standard solutions and ensured that the slope lies between 55–65 mV before the analysis of fluoride ion. In order to obtain an optimum pH during the measurement of fluoride ion, the total ionic strength was adjusted using (1
:
100) TISAB II buffer solution.
The initial pH adjustments of the aqueous solutions with the adsorbent were done using an Elico pH meter (Model LI-127) supplied by Elico, India. An incubator shaker was procured from Biotechnics, India. The electronic spectrum of graphene oxide was recorded using UV-visible spectrophotometer (Jasco model V 650). The FT-IR spectra were recorded by grinding 0.01 g of the adsorbent (in various forms) with spectroscopy grade KBr using a Jasco-4200 FT-IR spectrometer in the range 400 to 4000 cm−1 at 4 cm−1 resolution. FT Raman spectra of the adsorbent were recorded using a thermo electron corporation Nexus 670 model spectrometer using an NdYAG laser excitation source. The X-ray diffraction (XRD) pattern of GO, GO–Al–O(OH) adsorbent before and after the adsorption of fluoride ion were obtained using a Bruker AXS D8 Advance XRD diffractometer with Cu Kα radiation source (λ = 1.5406 Å) source energized at 40 kV and 35 mA with step size and time of 0.020°and 65.6 s respectively. The samples were scanned at the rate 2.0° min−1 in the 2θ range 5° to 100°. The surface morphology of all the samples were obtained using a JEOL Model JSM-6390LV scanning electron microscope along with the energy dispersive X-ray spectrum (JEOL Model JED – 2300). XPS data of the adsorbent and the starting material were recorded using a VG Multilab 2000 spectrometer (Thermo VG Scientific, Southend-On-Sea, Essex, UK) in an ultra-high vacuum and an unmonochromatized Mg Kα (1253.6 eV) X-ray source with a spherical section analyzer. Survey scans and core peak data were acquired with pass energies of 50 eV, respectively. The XPS spectrum calibration was done with hydrocarbon C1s peak at a binding energy of 284.5 eV.
Batch adsorption study
For the batch adsorption studies, about 50 mg of the GO–Al–O(OH) adsorbent was added to 100 mL of 5 mg L−1 fluoride ion solution in the pH range 6.5–7.5 and equilibrated in an orbital incubator shaker (Biotechnics, India) at different time intervals. The initial and final (equilibrium) concentrations of fluoride present in the solution phase were estimated using the F− ion selective electrode. The amount of fluoride adsorbed (mg g−1) at equilibrium (qe) was calculated using the following expression |
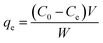 | (1) |
where C0 and Ce are the initial and final (equilibrium) fluoride ion concentrations (mg L−1) present in the aqueous phase, 0.1 L is the volume of aqueous solution and 0.05 g is the weight of the GO–Al–O(OH) adsorbent used in the adsorption studies. The adsorption percentage of fluoride increased with time and good removal efficiency occurs within 60 min thereby bringing the fluoride ion concentration to the allowable limits as per the WHO guidelines.
Results and discussion
Physicochemical characterization of adsorbent
Electronic and vibrational spectroscopy studies. Fig. 1A shows the UV-vis spectrum of synthesized graphene oxide which was dispersed with Milli-Q water by ultrasonication. The absorption around 225 nm along with a shoulder peak around 300 nm corresponds to π–π* transition of C–C bonds19 and C
O bonds of graphene oxide.20 Graphene oxide, a single layer of graphite oxide has various functional groups such as carboxyl, carbonyl, epoxy, hydroxyl21 and these were identified using FT-IR spectroscopy.
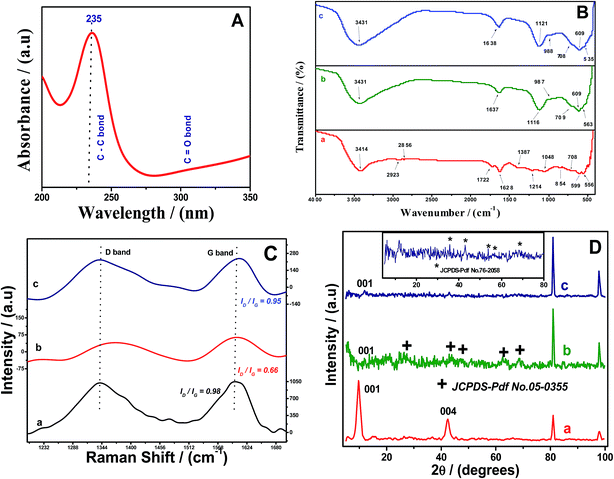 |
| Fig. 1 (A) UV-vis spectrum of graphene oxide (GO) (B) FT-IR spectra (C) Raman spectra (D) XRD spectra of synthesized GO (a), GO–Al–O(OH) adsorbent before (b) and after fluoride ion adsorption (c). | |
The FT-IR spectrum of native graphite (figure not shown) yields two peaks around 1672 cm−1 and 1536 cm−1 corresponding to C
C stretching vibrations as reported previously.22 In addition, the peaks at 3152 cm−1 and 1401 cm−1 correspond to aromatic C–H stretching and C–H bending respectively.22 Fig. 1B shows the FT-IR spectrum of graphene oxide, [Al–O(OH)] incorporated graphene oxide adsorbent and after the adsorption of fluoride. The peaks observed at 3414 cm−1 and 1387 cm−1 could be ascribed to C–OH groups that are introduced in the graphite matrix during oxidation.23 The absorption bands around 2856 cm−1 and 2923 cm−1 corresponds to νsym and νasym stretching vibrations of CH2.24 The broadness of peak at 3414 cm−1 could be attributed to the OH stretching vibrations.25 The peaks around 1725 cm−1 and 1627 cm−1 correspond to C
O stretching of carboxylic/carbonyl functional groups while the peaks at 1215 cm−1 and 1050 cm−1 correspond to C–O bond26,27 stretching vibrations confirming the formation of graphene oxide. After the impregnation of aluminium ion onto the surface of graphene oxide, the band at 3414 cm−1 was shifted to 3431 cm−1 and the peak broadened, indicating the interaction of metal ion with O–H groups of graphene oxide thereby increasing the proximity of Al–OH groups towards the surface of graphene oxide. In addition to this, the strong band at 609 cm−1 is assigned to the vibrational mode of AlO6 while another two bands at 1116 and 1637 cm−1 can be attributed to Al–OH stretching and bending vibrations in the boehmite lattice.28,29 With the adsorption of fluoride onto the adsorbent surface, the peak intensities are modified and the peak at 535 cm−1 could be related to the Al–F interaction.30
Raman spectroscopy is yet another valuable non-destructive spectroscopic tool used to acquire valuable evidence for carbonaceous materials such as graphene.31,32 Earlier reports33,34 suggest that two distinct bands (G and D) are observable in the Raman spectra of graphitic materials. In case of pristine graphite, these bands are obvious at 1351 cm−1 and 1582 cm−1. The D band is quite distinct for sp3 domains in carbon layers and the G band provides reliable information about the sp2 in-plane vibrations.31,35 Fig. 1C shows the Raman spectra of GO showing a strong band at 1601 cm−1 (G band) for the optical E2g in plane vibration at the Brillouin zone center and a weak band around 1340 cm−1 (D band) ascribed to A1g breathing mode of vibrations.36 Generally, in plane vibrations attributed to E2g would be Raman active for carbonaceous materials possessing sp2 hybridized carbon atoms. Nevertheless, the A1g mode of vibrations are normally activated, when some defects appear at near k-point of Brillouin zone through an inter-valley double-resonance Raman phenomenon.37 The intensity of D band is often used as a yardstick to quantify the extent of disorder in carbon-based materials. When the Al3+ ion interacts with GO, the intensity ratio ID/IG decreases from 0.98 to 0.66 and also the D band shows a shift from 1340 cm−1 to 1365 cm−1 which reveals the reduction of the oxidized molecular defects.37 Further, ID/IG ratio is inversely related to the average crystallite size in graphitic materials.38 After the fluoride adsorption, the intensity of ID/IG increases to 0.95 which implies that the level of disorder increases at surface as compared to the adsorbent thus leading to an increase in the ratio of ID/IG.
Powder X-ray diffraction (XRD) and SEM/EDS studies. Earlier literature reports suggest33,39 the distinct diffraction peaks for graphite at 2θ = 26.38° and 54.54° corresponding to the planes (002) and (004) planes respectively in accordance with JCPDS no. 00-041-1487. In case of GO (Fig. 1D) we observed the diffraction peaks (002) and (004), to be displaced by approximately 16° and 12° as a result of exfoliation of the graphite layers. The other diffraction peaks (100), (101), (102) and (103) characteristics of graphite were not observed and this indicates the presence of carbonyl, hydroxyl and carboxyl functional groups in GO during the exfoliation. The contributions to the reflection of other planes in graphite are negligible due to the exfoliation resulting from the oxidation of the graphite layers.38 Further, the interlayer distance of the (002) peak plane40 for GO is 9.10 Å (2θ = 9.709°) which is larger than the native graphite (3.37 Å), thereby confirming the expansion and exfoliation of graphite layers. The interlayer distance of GO increases to 9.24 Å in ethanolic medium compared to approximately 6.6 Å for a solvent free medium and this could be attributed to the intercalation of a solvent monolayer in the GO structure.41The XRD pattern of GO–Al–O(OH) adsorbent (Fig. 1D) clearly shows that (002) and (004) planes of graphene oxide completely disappeared and shifted to around 2θ = 12° due to the interaction of Al3+ ions with the surface functional groups of GO. Since graphite is c-axis oriented, during oxidation process the functional groups are primarily introduced in the (002) and (004) planes only. Due to the amorphous nature of metal hydroxide, new peaks appear with small intensities at 2θ = 27.05°, 43.44°, 47.17°, 62.89° and 68.03° corresponding to the (120), (140), (131), (151) and (061) planes and these could be attributed to the presence of aluminium oxy hydroxide on the surface of graphene oxide (JCPDS no. 05-0355). After the adsorption of fluoride ion, the peaks were observed at 35.65°, 42.92°, 53.99°, 56.38° and 68.45° corresponding to the (101), (210), (211), (220) and (301) planes of aluminium oxy fluoride (JCPDS no. 76-2058) indicating the replacement of O–H groups by fluoride ions to enhance the adsorption.
Certain marked differences in the SEM micrographs along with the EDS spectra (Fig. 2) of graphene oxide and metal hydroxide incorporated graphene oxide were observed. The SEM micrographs of GO show crumpled morphology with stacked layers. However, the adsorbent surface is adorned with some bright spots showing the presence of aluminium oxy hydroxide onto GO surface. After adsorption of fluoride ion, the surface of adsorbent appears more congested with some small weak spots attributed to the interaction of fluoride onto the adsorbent surface. Moreover, the SEM image of graphene oxide showed trace impurities on its surface due to the presence of Cl and S which was also confirmed through the EDS peaks at 2.62 keV and 2.31 keV respectively. The amorphous nature of Al–O(OH) is also visible in the SEM micrograph of the adsorbent. It is quite predictable from these images that Al–O(OH) is anchored on the surface of graphene oxide effectively. After fluoride adsorption, the surface of the adsorbent becomes almost homogeneous due to the agglomeration of Al–O(F) particles. The EDS spectrum (Fig. 2) confirms the presence of Al, O, Na and C elemental peaks at 1.486, 0.525, 1.041 and 0.277 keV and this clearly indicates that aluminium oxy hydroxide was loaded successfully onto the surface of GO.
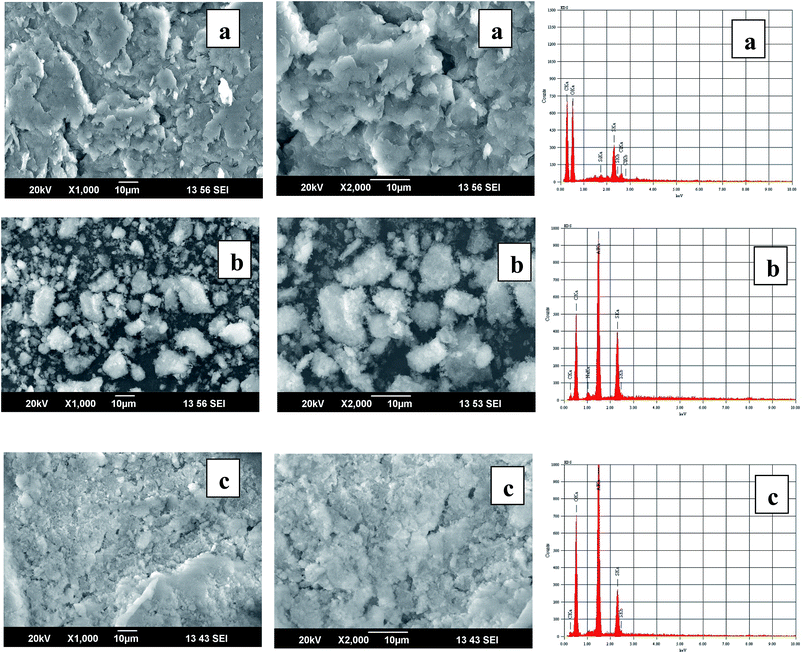 |
| Fig. 2 SEM images at different magnifications with corresponding EDS spectra of GO (a), Aluminium oxy hydroxide incorporated GO (b) and after adsorption of fluoride (c). | |
X-ray photoelectron spectroscopy studies. The XPS analysis was carried out to obtain valuable information about the surface changes during the preparation of graphene oxide from native graphite, GO–Al–O(OH) and to recognize the adsorbent surface changes before and after the adsorption of fluoride (Fig. 3). The full survey XPS spectra (Fig. 3A) shows the photoelectron lines at binding energy (B.E) values 285, 530, 74 and 685 eV attributed to C1s, O1s, Al2p and F1s respectively. The C1s XPS spectrum (Fig. 3B and Table 1) studies for graphite shows diverse peaks corresponding to non-oxygenated carbon (284.6 eV, 59.9%), in addition to the C–C (285.5 eV, 17.29%) bonding present in hexagonal graphite (284.1 eV, 17.5%).42 The assignment of peaks for the O1s spectra of graphite reveals that the B.E. at 530.5 (1.92%), 531.7 (1.47%), 533 (1.29%) and 534.5 eV (0.63%) could be the result of contributions from C
O or O
C–OH groups, surface chemisorbed oxygen species, C–OH groups and water respectively.43,44 This could probably emanate from the nature of fluid (CO, CO2 and CH4)-deposited onto graphite from the atmosphere.42 Also, the % C/O ratio (Table 1) for graphite was found to be 17.83 and for graphene oxide it decreased to 1.64%, confirming the oxidation of graphite. The high resolution C1s XPS spectrum of graphene oxide (Fig. 3B and Table 1) distinctly indicates considerable degree of oxidation with diverse functional groups including C
C (282.61 eV, 19.66%) C–OH (284.6 eV, 30.59%), and C–O–C or C
O bonds (286.12 eV, 8.89%).45,46 The XPS results are in good agreement with the FT-IR spectroscopic data discussed earlier. The O1s spectrum is more surface specific compared to C1s and the peaks present at 530.29, 529.19 and 531.4 eV in the O1s data of graphene oxide can be assigned to C–OH, C–O–C (O2− oxidation state47) and C
O groups respectively.
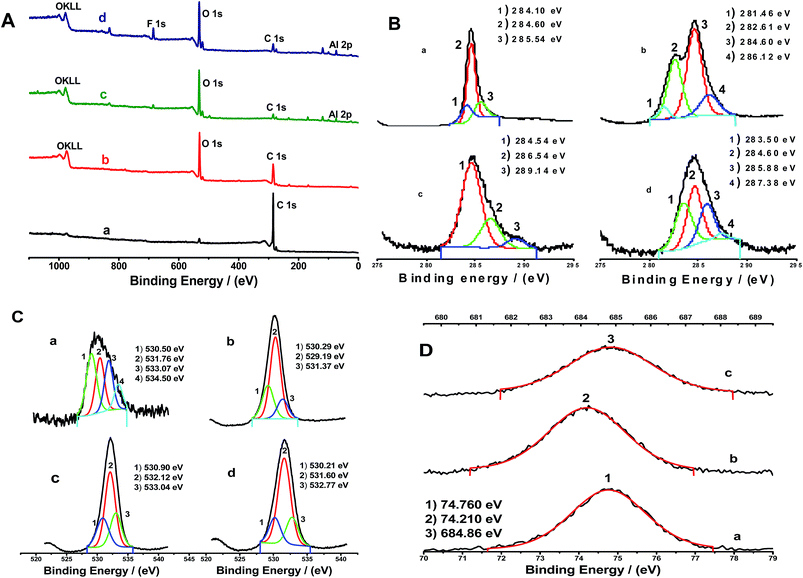 |
| Fig. 3 (A) Total survey of XPS spectra (B) C1s spectrum (C) O1s spectrum of graphite (a), prepared GO (b), GO–Al–O(OH) adsorbent (c) and after adsorption fluoride (d). (D) Al2p spectrum of GO–Al–O(OH) adsorbent (a) and fluoride adsorbed onto the adsorbent surface (b), F1s spectrum of F− ion adsorbed onto the adsorbent surface (c). | |
Table 1 Elemental composition of various atoms present on the adsorbent surface
Materials |
XPS analysis |
At% of C |
At% of O |
C/O ratio |
At% of Al |
At% of F |
Sp3 C |
Sp2 C |
C–OH |
C O orC–O |
Total |
Graphite |
17.29 |
77.74 |
— |
— |
94.69 |
5.31 |
17.83 |
— |
— |
Graphene oxide |
2.99 (C2−) |
19.66 |
30.59 |
8.89 |
62.13 |
37.88 |
1.64 |
— |
— |
GO–Al–O(OH) |
— |
13.97 |
4.80 |
1.50 |
20.27 |
60.27 |
0.34 |
19.46 |
Nil |
GO–Al–O(F) |
8.62 |
7.78 |
5.54 |
0.63 |
22.57 |
47.90 |
0.47 |
20.82 |
8.72 |
After the incorporation of aluminum oxy hydroxide, C/O ratio decreased from 1.64 to 0.34% which confirms the interaction between the graphene oxide and Al–O(OH). Furthermore, the high resolution C1s XPS spectrum of GO–Al–O(OH) clearly indicates that the Al–metal hydroxide interacts with hydroxyl, epoxy groups and carbonyl groups of graphene oxide. The decrease in intensity was apparent corresponding to the carbon atoms in different functional group environment such as C–OH (284.5 eV, 13.97%), and C–O–C or C
O bonds (286.54 eV, 4.8%), O
C–OH bond (289.14 eV, 1.5%). As shown in Fig. 3C, the O1s spectrum confirms that the Al–O(OH) could be anchored to graphene oxide by three different oxygen species namely, Al–O–Al at 531.0 eV (14.17%), Al–OH at 532.0 eV (31.11%) and adsorbed water (H–O–H) or C–OH of GO at 533.4 eV (14.99%). In addition, a distinct Al2p transition was observed at 74.7 eV, characteristic of Al–O(OH) or pseudoboehmite.48–50 In order to corroborate the interactions between fluoride and GO–Al–O(OH), XPS studies of the adsorbent before and after F− ion adsorption at pH 7.0 were conducted. After the fluoride adsorption, the high resolution O1s XPS spectra showed peaks at 531.6 eV (30.44%) which corresponds to the Al–OH formation and the peak at 532.7 eV (9.13%) suggests that the hydroxyl groups might be involved in the fluoride adsorption process (ligand exchange) and also the experimental Al2p peak maximum is shifted from 74.7 to 74.2 eV. Usually, the binding energies of organic fluoride (688.0 to 689.0 eV) are presumably higher than the metal fluoride (684.0 to 685.5 eV) and thus the adsorption of fluoride was confirmed through the high resolution F1s XPS spectra peak at 684.8 eV.51 This indicates the interaction of fluoride ion with Al3+ ions immobilized onto the surface of graphene oxide.
Influence of pH and a plausible mechanism for the interaction of fluoride ions onto the GO–Al–O(OH) adsorbent surface. The interaction of fluoride ions onto the GO–Al–O(OH) adsorbent surface depends on the solution pH, existence of fluoride in different forms (HF, F−, and HF2−) and zero-point charge or isoelectric point of the adsorbent surface. The pH at which the adsorbent surface acquires a neutral charge is referred to as zero-point charge and this could be easily determined by batch equilibrium technique.52 About 100 mg of GO–Al–O(OH) adsorbent was added to 100 mL F− ion solution (100 mg L−1) at different initial pH (3.0–9.0) by addition of 0.1 mol L−1 HCl or NaOH. The experiments were carried out in a thermostat shaker at 27 °C for 24 h. After a time period of 24 h, the final pH and F− ion concentration in the supernatant solutions was measured. The zero-point charge (pHzpc) of GO–Al–O(OH) adsorbent was determined from the plot of ΔpH [pHinitial − pHfinal] versus pHinitial (Fig. 4).
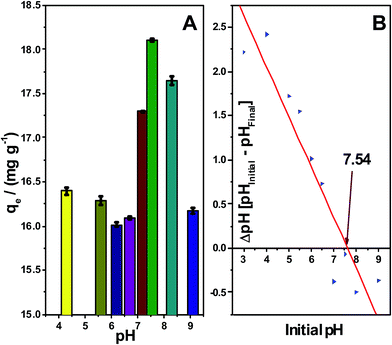 |
| Fig. 4 Adsorption of fluoride ion influenced by pH and zero point charge onto the adsorbent surface. | |
The resultant zero-point charge of GO–Al–O(OH) adsorbent was found to be 7.54 which implies that the adsorbent surface is positive below pHzpc and negative above pHzpc. The influence of pH on the adsorbent is shown in Fig. 4. The maximum equilibrium adsorption capacity of fluoride was observed at pH 7.5–8.3 (18.10 mg g−1 at pH 7.5 and 17.65 mg g−1 at pH 8.3) but at pH below 6.0 or above 8.5, the adsorption capacity also reduced marginally owing to the solubility of aluminium fluoride and Al(OH)4− in aqueous phase. Al3+ ion anchors onto surface of graphene oxide effectively (%Al present in the adsorbent is almost same before and after adsorption of fluoride). Indeed, with an initial concentration of 10 mg L−1 of F− ion, over the pH range 7.0–8.5, the level of fluoride in the solution phase was within the permissible limits.
The probable mechanism for the adsorption of fluoride ion onto the surface of GO–Al–O(OH) adsorbent is shown in Fig. 5. According to the HSAB principle,53 Al3+ is a hard acid with small ionic radius (0.5 Å) and it could interact effectively with hard base F− to enhance the adsorption. In aqueous solution, Al3+ could exist as cationic hydroxides such as Al(OH)2+, Al(OH)2+ etc. and these species could interact with fluoride ions by electrostatic attractive forces. The XPS surveys of O1s for GO–Al–O(OH) adsorbent and spent adsorbents were used to explain clearly the fluoride ion adsorption mechanism. The high resolution O1s spectrum of adsorbent (prior and after fluoride ion adsorption) showed that the adsorption of fluoride would enhance by the surface hydroxyl groups present in the adsorbent. Fig. 3C shows the XPS peaks of Al–OH at 532.5 eV (31.11%) and Al–O2− at 530.9 eV (14.17%) respectively.48 The loss of Al–OH (almost 22%) content is due to the formation of Al–F bonds (Table 1) which indicates the adsorption process to involve ligand exchange between hydroxyl groups and fluoride ions. The presence of Al–F fluoride was confirmed by the XPS peak observed at 684.8 eV.51 Moreover, hydrogen bonding and electrostatic interaction would also enhance the adsorption of fluoride at different pH levels onto the surface of GO–Al–O(OH) material.
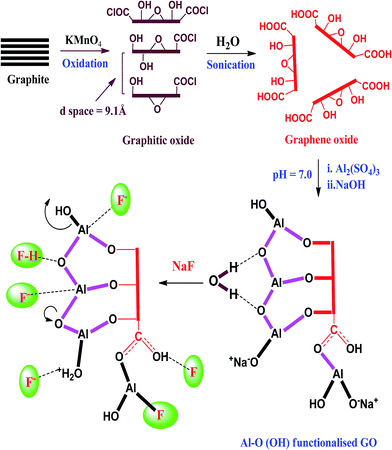 |
| Fig. 5 Preparation of GO and GO–Al–O(OH) adsorbent and the possible mechanism towards the fluoride ion adsorption. | |
Adsorption isotherm studies. The experimental data were analyzed by equilibrating varying initial concentrations of fluoride ion (5, 10, 20, 30, 40, 50 and 60 mg L−1) with a known weight (50 mg) of the adsorbent and fitted using various adsorption isotherms. Fig. 6A–F shows the different isotherm models that were applied to study adsorption behavior by measuring the amount of fluoride at equilibrium and concentration left in solution after adsorption onto GO–Al–O(OH) adsorbent surface. The various isotherm equations and parameters are given in Table 2. Generally, Langmuir54 and Freundlich isotherms55 are widely used to study adsorption. Various other empirical models (Table 2) also add considerable insight into the nature or mechanism of adsorption. The plot of Ce/qe against Ce, corresponding to Langmuir isotherm model gave a maximum adsorption capacity (q0) of 51.42 mg g−1. This indicates the admirable capability of GO–Al–O(OH) adsorbent towards F− ion adsorption. In addition to this, the separation factor (RL = 1/1 + bC0) found to be less than unity showing the applicability of Langmuir model to fit the data. For the Freundlich isotherm model, the intensity of adsorption (n) and the adsorption capacity (KF) were obtained from the plot of log
qe against log
Ce and if Freundlich constant n lies between 1and10, it indicates the favourable adsorption of F− ion onto the Al–O(OH) modified GO adsorbent. The high values of n and KF as shown in Table 2 signify the effective uptake of F− ion onto the GO–Al–O(OH) adsorbent surface. The Dubinin–Radushkevich isotherm (D–R)56 has similarity to Langmuir model and it gives the adsorption energy (β) and adsorption mechanism involved in the interaction between fluoride ion and the GO–Al–O(OH) adsorbent surface. The adsorption energy, E can also be expressed as −(2β)−0.5 and the positive value of E (+1.4924 kJ mol−1) indicates that the interaction between the fluoride anion and GO–Al–O(OH) adsorbent is endothermic and hence higher temperatures are favourable for adsorption. In addition to this, Temkin57 isotherm obtained from the plot of qe against ln
Ce accounts that the heat of adsorption decreases linearly with coverage due to F−–GO–Al–O(OH) interactions with uniform binding energy distribution. The value of b (kJ mol−1) was found to 0.243 which shows the electrostatic interaction between fluoride ion and GO–Al–O(OH) adsorbent. The exponent g obtained from Redlich–Peterson (R–P) isotherm model58 was found to be 0.75 and this explains the fact that adsorption of fluoride could be described satisfactorily through the Langmuir model. Comparing the regression coefficient values, the adsorption of fluoride onto the surface of GO–Al–O(OH) adsorbent follows the order, Langmuir = D–R > R–P > Temkin > Freundlich model.
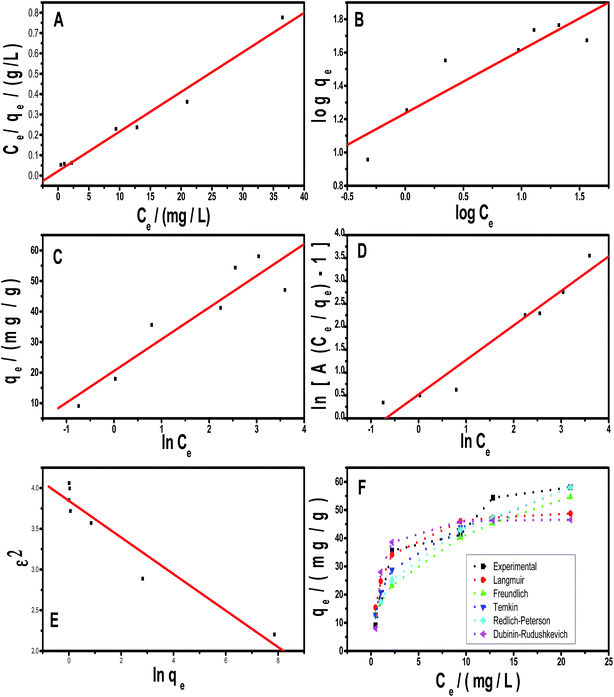 |
| Fig. 6 Isotherm models (A) Langmuir plot (B) Freundlich plot (C) Temkin plot (D) Redlich–Peterson (R–P) plot (E) Dubinin–Radushkevich (D–R) plot (F) Plot of qe vs. Ce. | |
Table 2 Isotherm parameters for the adsorption of fluoride
Linear equation & isotherm |
Parameters |
Langmuir54 |
q0 (mg g−1) |
b (L mg−1) |
RL |
r2 |
χ2 |
51.41 |
0.8971 |
0.1003 |
0.97 |
1.1905 |
Freundlich55 |
KF (mg1−1/n g−1 L1/n) |
n |
r2 |
χ2 |
17.18 |
2.6305 |
0.79 |
2.3679 |
ln qe = ln qm − βε2 Dubinin Radushkevich56 |
qm (mg g−1) |
β (mol2 kJ−2) |
E (kJ mol−1) |
r2 |
χ2 |
46.67 |
0.2245 |
1.4924 |
0.97 |
1.4287 |
qe = B1ln KT + B1ln Ce Temkin57 |
KT |
B |
r2 |
b (kJ mol−1) |
χ2 |
7.2095 |
10.3819 |
0.83 |
0.243 |
1.0835 |
Redlich–Peterson58 |
g |
A (L g−1) |
r2 |
χ2 |
0.7537 |
46.1255 |
0.93 |
1.4562 |
Adsorption kinetics and thermodynamics. The kinetics were analyzed through the pseudo first order,59 second order,60 and intraparticle diffusion rate equations61 (Table 3) for the adsorption of F− ion onto the GO–Al–O(OH) adsorbent. The various kinetic plots applied for F− ion adsorption and the corresponding kinetic parameters are shown in Fig. 7A–C and Table 3. In Weber–Morris61 intraparticle diffusion model, a plot of qt versus t 0.5 would be linear and if the plot passes through the origin then we could infer that intraparticle diffusion is the only rate-determining step. The plot was linear in this adsorption process giving the intraparticle rate constant (kint) and a non-zero intercept (Fig. 7C) pointing to the fact that boundary layer phenomenon could also direct the adsorption of F− ion onto the GO–Al–O(OH) adsorbent surface. The value of regression coefficient (r2) for the pseudo-second-order adsorption model was almost unity. Also, the calculated qe value through this model matched well with experimental (qe) value. Hence, the pseudo second-order kinetic model is more suitable to describe the kinetic behavior of F− ion adsorption onto GO–Al–O(OH) adsorbent surface.
Table 3 Kinetic parameters for the adsorption of fluoride onto Al–O(OH) incorporated graphene oxide
Concentration of F− ion (mg L−1) |
qe mg g−1 |
Pseudo first order kinetic model59 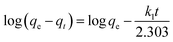
|
Pseudo second order kinetic model60 
|
Intraparticle diffusion model61 qt = kintt0.5 |
k1 min −1 |
q1 mg g−1 |
r2 |
k2 g mg min−1 |
q2 mg g−1 |
r2 |
kint g mg−1 (min0.5)−1 |
r2 |
5.0 |
9.048 |
0.0445 |
2.9117 |
0.78 |
0.0178 |
10.521 |
0.99 |
0.7897 |
0.86 |
10.0 |
17.940 |
−1.7960 |
0.9722 |
0.88 |
0.0200 |
17.141 |
0.99 |
0.8064 |
0.92 |
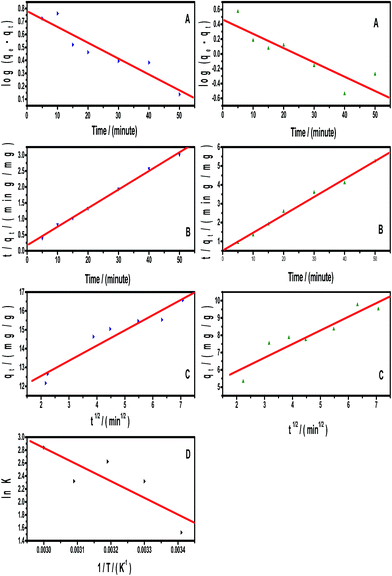 |
| Fig. 7 Kinetic plots obtained from the (A) pseudo first-order equation (B) pseudo second-order equation (C) intra-particle diffusion kinetics equation with 5.0 mg L−1 and 10.0 mg L−1 F− ion concentrations (D) Van't Hoff isotherm plot obtained for the adsorption of F− ion onto GO–Al–O(OH) adsorbent surface. | |
The F− ion adsorption was tested at different temperatures using 10 mg L−1 of fluoride to ascertain the spontaneity from the equilibrium constant (K) values. Adsorption free energy (ΔG0), adsorption enthalpy (ΔH0) and adsorption entropy (ΔS0) were calculated from the Gibbs isotherm equation (ΔG° = −RT ln K) and classic Van't Hoff plot of ln
K against 1/T (Fig. 7D). Also, the activation energy (Ea) required for favourable F− ion adsorption was obtained using the expression Ea = ΔH0ads + RT. The adsorption free energy shows negative values (−6.094 kJ mol−1) which elucidates the spontaneity of F− ion adsorption process onto the GO–Al–O(OH) adsorbent surface. Adsorption enthalpy (ΔH0) is normally lower than 80 kJ mol−1 for physical adsorption process and Table 4 shows the adsorption enthalpy (+21.384 kJ mol−1) and the average activation energy (+23.986 kJ mol−1) as positive thereby supporting the fact the adsorption of F− ion could involve ligand exchange by physical adsorption process. Entropy is an extensive thermodynamic property and the positive value of ΔS0 (87.71 J mol−1 K−1) also testifies the feasibility and favorable adsorption of F− ion onto GO–Al–O(OH) adsorbent surface.
Table 4 Adsorption thermodynamic parameters
Temperature/kelvin |
Van't Hoff equation 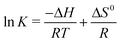
|
ΔGo/kJ mol−1 |
ΔHo/kJ mol−1 |
ΔSo/J K−1 mol−1 |
Ea/kJ mol−1 |
293 |
−3.72 |
+21.384 |
+87.71 |
+23.986 |
303 |
−5.84 |
313 |
−6.82 |
323 |
−6.23 |
333 |
−7.86 |
Comparison and laboratory column studies. The effectiveness of this novel GO–Al–O(OH) adsorbent was compared with other adsorbents towards the defluoridation of water.14,62–67 The maximum adsorption capacity values in Table 5 show that the prepared adsorbent has good ability for defluoridation in field applications.
Table 5 Evaluation of adsorption capacity obtained from the present work against other sorbents
Sl. no. |
Adsorbent |
Adsorption capacity (mg g−1) |
Reference |
1 |
Nano AlOOH |
3.26 |
62 |
2 |
Lanthanum impregnated activated alumina |
16.90 |
63 |
3 |
Zirconium–carbon hybrid adsorbent |
17.70 |
64 |
4 |
CTAB functionalized CNTs |
20.10 |
65 |
5 |
Al2O3/CNTs |
28.70 |
66 |
6 |
Basic aluminum sulfate@graphene hydrogel |
33.40 |
14 |
7 |
Zirconium ion impregnated coconut fiber carbon |
40.01 |
67 |
8 |
Al–O(OH) incorporated graphene oxide |
51.41 |
Present study |
About 2.0 g of GO–Al–O(OH) adsorbent was packed into a short glass column for checking the applicability on a laboratory scale. A known volume (2.0 L) of 5 mg L−1 F− ion solution prepared in Milli Q water was fed to the column. The fluoridated solution passed through the adsorbent column at a flow rate of 6 mL min−1, the out coming F− ion concentration present in water was monitored frequently. The concentration of fluoride after treatment was found to be within the permissible limits (1.0–1.5 mg L−1). The column could be re-used more than three times by desorption with 30 mL of 1.0 mol L−1 NH4OH as ammonium fluoride in the eluate.
Conclusions
This work has described well the interaction between the graphene oxide modified Al–O(OH) adsorbent and fluoride. The maximum adsorption capacity (qmax) of this novel GO–Al–O(OH) adsorbent is 51.42 mg g−1 and the adsorption could be explained satisfactorily through Langmuir and Dubinin–Radushkevich isotherm models. FT-IR and Raman spectroscopic techniques proved to be a valuable tool to comprehend the adsorption mechanism. Ligand exchange and physical adsorption are mostly favored for the adsorption of fluoride and this was corroborated from the XPS studies. In addition to this, the thermodynamic feasibility and pseudo second order kinetic model augurs this adsorption process. With 2.0 g of the adsorbent packed on laboratory scale column, it is possible to treat 2000 mL of 5.0 mg L−1 fluoride solution at pH 7.0 thereby bringing the concentration within the permissible limits. This is possible at the normal pH existing in water and hence the Al–O(OH) impregnated graphene oxide adsorbent could demonstrate its efficacy for field applications.
Acknowledgements
We acknowledge the financial support from Department of Science and Technology (DST), New Delhi, India (Project no: DST/TM/WTI/2K11/350(G)). Thanks to Central Electrochemical Research Institute (CECRI) Karaikudi, India, Sophisticated Analytical Instrumentation Facility, Cochin, India and Mrs Park Jin Hee, Centre for the Development of Fine Chemicals at Chonnam National University, South Korea, for their valuable assistance in characterization.
References
- Guidelines for drinking-water quality, World Health Organization (WHO), Geneva, 4th edn, 2011, http://whqlibdoc.who.int/publications/2011/9789241548151_eng.pdf Search PubMed
. - H. Lounici, L. Addour, D. Belhocine, H. Grib, S. Nicolas, B. Bariou and N. Mameri, Desalination, 1997, 114, 241–251 CrossRef CAS
. - M. G. Sujana, H. K. Pradhan and S. Anand, J. Hazard. Mater., 2009, 161, 120–125 CrossRef CAS PubMed
. - S. Jagtap, M. K. Yenkie, N. Labhsetwar and S. Rayalu, Chem. Rev., 2012, 112, 2454–2466 CrossRef CAS PubMed
. - Y. H. Li, S. Wang, X. Zhang, J. Wei, C. Xu, Z. Luan and D. Wu, Mater. Res. Bull., 2003, 38, 469–476 CrossRef CAS
. - P. Chingombe, B. Saha and R. J. Wakeman, Carbon, 2005, 43, 3132–3143 CrossRef CAS
. - D. R. Dreyer, S. Park, C. W. Bielawski and R. S. Ruoff, Chem. Soc. Rev., 2010, 39, 228–240 RSC
. - K. S. Subrahmanyam, S. R. C. Vivekchand, A. Govindaraj and C. N. R. Rao, J. Mater. Chem., 2008, 18, 1517–1523 RSC
. - Y. Li, P. Zhang, Q. Du, X. Peng, T. Liu, Z. Wang, Y. Xia, W. Zhang, K. Wang, H. Zhu and D. Wu, J. Colloid Interface Sci., 2011, 363, 348–354 CrossRef CAS PubMed
. - T. Poursaberi, M. R. Ganjali and M. Hassanisadi, Talanta, 2012, 101, 128–134 CrossRef CAS PubMed
. - X. Luo, C. Wang, L. Wang, F. Deng, S. Luo, X. Tu and C. Au, Chem. Eng. J., 2013, 220, 98–106 CrossRef CAS
. - E. Zong, D. Wei, H. Wan, S. Zheng, Z. Xu and D. Zhu, Chem. Eng. J., 2013, 221, 193–203 CrossRef CAS
. - Y. Li, Q. Du, J. Wang, T. Liu, J. Sun, Y. Wang, Z. Wang, Y. Xia and L. Xia, J. Fluorine Chem., 2013, 148, 67–73 CrossRef CAS
. - Y. Chen, Q. Zhang, L. Chen, H. Bai and L. Li, J. Mater. Chem. A, 2013, 1, 13101–13110 CAS
. - M. Bai, J. Wang, W. Wu, X. Zeng and J. Chen, Mater. Lett., 2014, 116, 178–181 CrossRef CAS
. - M. U. Sankar, S. Aigal, S. M. Maliyekkal, A. Chaudhary, Anshup, A. A. Kumar, K. Chaudhari and T. Pradeep, Proc. Natl. Acad. Sci. U. S. A., 2013, 110, 8459–8464 CrossRef CAS PubMed
. - Y. Chen, L. Chen, H. Bai and L. Li, J. Mater. Chem. A, 2013, 1, 1992–2001 CAS
. - D. C. Marcano, D. V. Kosynkin, J. M. Berlin, A. Sinitskii, Z. Sun, A. Slesarev, L. B. Alemany, W. Lu and J. M. Tour, ACS Nano, 2010, 4, 4806–4814 CrossRef CAS PubMed
. - V. H. Pham, T. V. Cuong, S. H. Hur, E. W. Shin, J. S. Kim, J. S. Chung and E. J. Kim, Carbon, 2010, 48, 1945–1951 CrossRef CAS
. - X. Sun, Z. Liu, K. Welsher, J. Robinson, A. Goodwin, S. Zaric and H. Dai, Nano Res., 2008, 1, 203–212 CrossRef CAS PubMed
. - S. Eigler, S. Grimm, M. Enzelberger-Heim, P. Muller and A. Hirsch, Chem. Commun., 2013, 49, 7391–7393 RSC
. - A. S. K. Kumar and N. Rajesh, RSC Adv., 2013, 3, 2697–2709 RSC
. - X. Li, G. Zhang, X. Bai, X. Sun, X. Wang, E. Wang and H. Dai, Nat. Nanotechnol., 2008, 3, 538–542 CrossRef CAS PubMed
. - Y. Lin, S. Xu and J. Li, Chem. Eng. J., 2013, 225, 679–685 CrossRef CAS
. - T. F. Yeh, J. M. Syu, C. Cheng, T. H. Chang and H. Teng, Adv. Funct. Mater., 2010, 20, 2255–2262 CrossRef CAS
. - Y. Xu, H. Bai, G. Lu, C. Li and G. Shi, J. Am. Chem. Soc., 2008, 130, 5856–5857 CrossRef CAS PubMed
. - T. Rattana, S. Chaiyakun, N. Witit-anun, N. Nuntawong, P. Chindaudom, S. Oaew, C. Kedkeaw and P. Limsuwan, Procedia Eng., 2012, 32, 759–764 CrossRef CAS
. - A. B. Kiss, G. Keresztury and L. Farkas, Spectrochim. Acta, Part A, 1980, 36, 653–658 CrossRef
. - Y. Feng, W. Lu, L. Zhang, X. Bao, B. Yue, Y. lv and X. Shang, Cryst. Growth Des., 2008, 8, 1426–1429 CAS
. - M. Barathi, A. Santhana Krishna Kumar and N. Rajesh, J. Environ. Chem. Eng., 2013, 1, 1325–1335 CrossRef CAS
. - A. C. Ferrari, J. C. Meyer, V. Scardaci, C. Casiraghi, M. Lazzeri, F. Mauri, S. Piscanec, D. Jiang, K. S. Novoselov, S. Roth and A. K. Geim, Phys. Rev. Lett., 2006, 97, 187401 CrossRef CAS PubMed
. - I. Calizo, A. A. Balandin, W. Bao, F. Miao and C. N. Lau, Nano Lett., 2007, 7, 2645–2649 CrossRef CAS PubMed
. - G. Sun, X. Li, Y. Qu, X. Wang, H. Yan and Y. Zhang, Mater. Lett., 2008, 62, 703–706 CrossRef CAS
. - A. C. Ferrari, Solid State Commun., 2007, 143, 47–57 CrossRef CAS
. - F. Tuinstra and J. L. Koenig, J. Chem. Phys., 1970, 53, 1126–1130 CrossRef CAS
. - M. A. Pimenta, G. Dresselhaus, M. S. Dresselhaus, L. G. Cancado, A. Jorio and R. Saito, Phys. Chem. Chem. Phys., 2007, 9, 1276–1290 RSC
. - C. Zhu, S. Guo, Y. Fang and S. Dong, ACS Nano, 2010, 4, 2429–2437 CrossRef CAS PubMed
. - R. D. Martínez-Orozco, H. C. Rosu, S. W. Lee and V. Rodríguez-González, J. Hazard. Mater., 2013, 263, 52–60 CrossRef PubMed
. - Z. Q. Li, C. J. Lu, Z. P. Xia, Y. Zhou and Z. Luo, Carbon, 2007, 45, 1686–1695 CrossRef CAS
. - H. Sun, S. Liu, G. Zhou, H. M. Ang, M. O. Tadé and S. Wang, ACS Appl. Mater. Interfaces, 2012, 4, 5466–5471 CAS
. - S. You, S. Luzan, J. Yu, B. Sundqvist and A. V. Talyzin, J. Phys. Chem. Lett., 2012, 3, 812–817 CrossRef CAS PubMed
. - G. Parthasarathy, B. Sreedhar and T. R. K. Chetty, Curr. Sci., 2006, 90, 995–1000 CAS
. - D. Yang, A. Velamakanni, G. Bozoklu, S. Park, M. Stoller, R. D. Piner, S. Stankovich, I. Jung, D. A. Field, C. A. Ventrice Jr and R. S. Ruoff, Carbon, 2009, 47, 145–152 CrossRef CAS
. - J. Filik, P. W. May, S. R. J. Pearce, R. K. Wild and K. R. Hallam, Diamond Relat. Mater., 2003, 12, 974–978 CrossRef CAS
. - G. Venugopal, K. Krishnamoorthy, R. Mohan and S.-J. Kim, Mater. Chem. Phys., 2012, 132, 29–33 CrossRef CAS
. - W. Wan, Z. Zhao, H. Hu, Y. Gogotsi and J. Qiu, Mater. Res. Bull., 2013, 48, 4797–4803 CrossRef CAS
. - M. V. Kuznetsov, J. F. Zhuravlev and V. A. Gubanov, J. Electron Spectrosc. Relat. Phenom., 1992, 58, 169–176 CrossRef CAS
. - J. T. Kloprogge, L. V. Duong, B. J. Wood and R. L. Frost, J. Colloid Interface Sci., 2006, 296, 572–576 CrossRef CAS PubMed
. - I. Balchev, N. Minkovski, T. Marinova, M. Shipochka and N. Sabotinov, J. Mater. Sci. Eng. B, 2006, 135, 108–112 CrossRef CAS
. - S. Thomas and P. M. A. Sherwood, Anal. Chem., 1992, 64, 2488–2495 CrossRef CAS
. - X. Wu, Y. Zhang, X. Dou, B. Zhao and M. Yang, Chem. Eng. J., 2013, 223, 364–370 CrossRef CAS
. - A. E. Ofomaja and Y.-S. Ho, Bioresour. Technol., 2008, 99, 5411–5417 CrossRef CAS PubMed
. - R. G. Pearson, J. Am. Chem. Soc., 1963, 85, 3533–3539 CrossRef CAS
. - I. Langmuir, J. Am. Chem. Soc., 1918, 40, 1361–1403 CrossRef CAS
. - H. M. F. Freundlich, Z. Phys. Chem., 1906, 57A, 385–470 Search PubMed
. - M. M. Dubinin, Chem. Rev., 1960, 60, 235–241 CrossRef CAS
. - M. I. Temkin, Zh. Fiz. Khim., 1941, 15, 296–332 CAS
. - O. Redlich and D. L. Peterson, J. Phys. Chem., 1959, 63, 1024 CrossRef CAS
. - S. Lagergren, K. Sven. Vetenskapsakad. Handl., 1898, 24, 1–39 Search PubMed
. - Y. Nie, C. Hu and C. Kong, J. Hazard. Mater., 2012, 233–234, 194–199 CrossRef CAS PubMed
. - W. J. Weber and J. C. Morris, J. Sanit. Eng. Div., Am. Soc. Civ. Eng., 1963, 89, 3–60 Search PubMed
. - S. G. Wang, Y. Ma, Y. J. Shi and W. X. Gong, J. Chem. Technol. Biotechnol., 2009, 84, 1043–1050 CrossRef CAS
. - Q. Shi, Y. Huang and C. Jing, J. Mater. Chem. A, 2013, 1, 12797–12803 CAS
. - L. H. Velazquez-Jimenez, R. H. Hurt, J. Matos and J. R. Rangel-Mendez, Environ. Sci. Technol., 2013, 48, 1166–1174 CrossRef PubMed
. - N. Sankararamakrishnan, N. Singh and A. Gupta, RSC Adv., 2013, 3, 22421–22429 RSC
. - Y.-H. Li, S. Wang, A. Cao, D. Zhao, X. Zhang, C. Xu, Z. Luan, D. Ruan, J. Liang, D. Wu and B. Wei, Chem. Phys. Lett., 2001, 350, 412–416 CrossRef CAS
. - R. S. Sathish, S. Sairam, V. G. Raja, G. N. Rao and C. Janardhana, Sep. Sci. Technol., 2008, 43, 3676–3694 CrossRef CAS
.
Footnote |
† Present address: Department of Chemistry, National Sun Yat-sen University, Kaohsiung City, Taiwan. |
|
This journal is © The Royal Society of Chemistry 2014 |
Click here to see how this site uses Cookies. View our privacy policy here.