DOI:
10.1039/C4RA09973G
(Paper)
RSC Adv., 2014,
4, 60702-60710
A magnetically recyclable Fe3O4@SiO2/Mn(III) chiral salen complex as a highly selective and versatile heterogeneous nanocatalyst for the oxidation of olefins and sulfides
Received
7th September 2014
, Accepted 4th November 2014
First published on 5th November 2014
Abstract
In this study, a new magnetically recoverable heterogeneous Mn(III) nanocatalyst (MHMC) was developed by covalent grafting of a homogeneous chiral Mn(III) salen complex (HMC) onto the surface of a Fe3O4/SiO2 core–shell. The structural and chemical nature of MHMC was characterized by different techniques. The catalytic activity of the MHMC and comparison with its homogeneous analogue were studied for the enantioselective epoxidation of various alkenes to epoxide compounds using tert-butyl hydroperoxide (TBHP) as an oxidant. The MHMC showed higher enantioselectivity and comparable catalytic reactivity to its homogeneous analogue with benefits such as facile recovery and recycling of the heterogeneous catalyst. The results showed that a higher enantioselectivity and yield were observed in the presence of N-methylmorpholine N-oxide (NMNO). The catalytic activity of this complex was also tested in the oxidation of sulfides using aqueous 30% H2O2 in excellent yields. The catalyst was reused for several runs without significant loss in activity and selectivity.
Introduction
Chiral Mn(III) salen complexes as versatile and useful catalysts have been used for oxidative transformations.1 In homogeneous systems, recycling of catalysts and separation of products from the reaction system is difficult. Because of the inherent advantages of heterogeneity, including efficient product purification and easy catalyst recovery, the heterogenization of chiral Mn(III) salen complexes has received much attention.2–6 Several different strategies have focused on the covalent binding of manganese(III) salen complexes onto insoluble solid supports such as mesoporous materials, zeolites, polymer supports, organic polymer–inorganic hybrid materials, clays, MCM-41, SBA-15 and activated silica.7–15 Compared to the reactions performed in the homogeneous phase, the covalent attachment procedures generally lead to a decrease in catalyst performance, but the heterogenized catalysts are stable against active phase leaching and could be recycled for several times.16–19 From economic point of view, the separation of ultra scaled heterogeneous catalysts from reaction system via routine methods such as free sedimentation, centrifugation and filtration is difficult, time-consuming and costly. To overcome this issue, the use of magnetic nanoparticles (MNPs) which can be easily separated by applying a simple external magnet without any significant loss of activity put forward a solution to this problem. Furthermore, magnetic nanoparticles are widely studied in the fields of biomedicine, magnetic resonance imaging (MRI), biotechnology, separation and catalysis.20–24
On the other hand, selective oxidation of organic compounds is a fundamental reaction in the synthesis of biologically active intermediates such as sulfoxides, sulfones and epoxides.25 Sulfoxide are well known as valuable intermediates for the synthesis of various useful compounds, including antibacterial, antifungal, antiulcer, and anti-hypertensive agents.26 Sulfones which are also synthesized by over-oxidation of sulfoxides, exhibit different biological activities and have been the subject of extensive investigation.27–29
Also, asymmetric epoxidation of alkenes into unique labile three-membered ether rings as an important fundamental organic transformation is of great attention in chemical and pharmaceutical industries.30–32
Now, in continuation of our ongoing research on the application of heterogeneous catalyst in organic synthesis33–35 and development of novel catalytic oxidation methods using environmentally benign oxidants, such as hydrogen peroxide (H2O2) and tert-butyl hydroperoxide (TBHP), we now report design and characterization of the covalent attachment of HMC onto Fe3O4/SiO2 NPs and its catalytic properties in the enantioselective epoxidation of alkenes using TBHP as an oxidant in dichloromethane. We also described selective oxidation of sulfides to sulfoxides and solfones using H2O2 as oxidant in water as a green media. By using this catalyst, the corresponding products were obtained in excellent yields and MHMC which is recovered from the reaction mixture by using external magnetic fields simplified the isolation of products.
Results and discussion
The immobilization of HMC on Fe3O4/SiO2 is illustrated in Scheme 1.
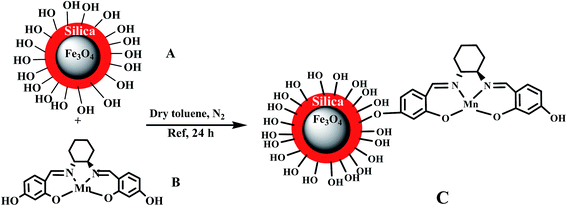 |
| Scheme 1 Schematic illustration for the preparation of MHMC catalyst. | |
Characterizations of catalyst
MHMC nanoparticle was simply synthesized by covalent grafting of HMC on Fe3O4/SiO2 (Scheme 1). The magnetic properties of the Fe3O4, Fe3O4/SiO2 and MHMC were studied by a vibrating sample magnetometer (VSM) at 300 K. As shown in Fig. 1, all of the nanoparticles have superparamagnetism at 300 K. Also, no hysteresis phenomenon was observed in Fig. 1. The saturation magnetization values for Fe3O4, Fe3O4/SiO2 and MHMC catalyst were 70.495, 38.30 and 34.30 emu g−1, respectively. Decreasing in the saturation magnetization values of Fe3O4/SiO2 and MHMC catalyst is depending on the immobilization of SiO2 and HMC onto Fe3O4, respectively. However, despite the considerably decrease of the magnetization of MHMC compared to Fe3O4, MHMC can still be separated from the solution by using an external magnetic field on the sidewall of the reactor.
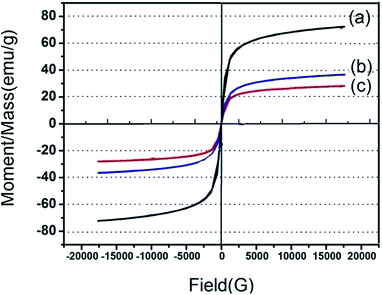 |
| Fig. 1 Magnetization curves of (a) Fe3O4 (b) Fe3O4/SiO2 (c) MHMC at 300 K. | |
The XRD pattern of Fe3O4, Fe3O4/SiO2 and MHMC were determined by powder X-ray diffraction (XRD). As shown in Fig. 2(a), the XRD pattern of Fe3O4 indicates a crystallized structure at 2θ: 30.2°, 35.4°, 43.3°, 53.6°, 57.5° and 63.1° which are assigned to the (2 2 0), (3 1 1), (4 0 0), (4 2 2), (5 1 1) and (4 4 0) crystallographic faces of magnetite. The XRD pattern of Fe3O4/SiO2 and MHMC show a broad peak at 2θ = 15–27° which is depend on amorphous silica (Fig. 2b and c). The size of these particles could be estimated from the Debye–Scherrer eqn (1) by determining the width of the (3 1 1) Bragg reflection (1).
|
 | (1) |
λ is the wavelength of the X-ray,
k is the Scherrer constant,
β is the half width of the peak and
θ is half of the Bragg angle. Therefore, the size (
D) of the particles could be easily estimated. The average diameter of Fe
3O
4 NPs was about 13 nm, while the diameter of Fe
3O
4/SiO
2 NPs was about 18 nm which is due to the agglomeration of Fe
3O
4 inside nanosphere and surface growth of silica on the shell. The IR spectrum of Fe
3O
4 shows important vibration bands in 560–590 and 3400 cm
−1, which are due to Fe–O and O–H, respectively (
Fig. 3a). According to the IR spectra of Fe
3O
4/SiO
2 in
Fig. 3(b), there are several important vibration bands in 560–590, 954, and 3400 cm
−1, which are due to Fe–O, Si–OH, and O–H, respectively. The vibration bands in 755 and 1100 cm
−1 are due to Si–O–Si. These vibration bands (755, 954 and 1100 cm
−1) confirmed coating of the silica shell on the surface of the Fe
3O
4 NPs. The IR spectra of HMC showed the strong band at 1590 cm
−1 which is assigned coordination of Mn through C
![[double bond, length as m-dash]](https://www.rsc.org/images/entities/char_e001.gif)
N functional groups (
Fig. 3d). The new stretching vibration bonds at 2930, 2880 and 1590 cm
−1 suggests the grafting of HMC on the Fe
3O
4/SiO
2 NPs (
Fig. 3c). The TGA curve of Fe
3O
4/SiO
2 shows a weight loss over the range of 90–160 °C of about 3%. This loss can be attributed to the loss of adsorb water and dehydroxylation of internal –OH groups. The second weight loss step is over the range 250–590 °C which can be ascribed to even further decomposition of the materials. The total weight losses are approximately 10% (
Fig. 4a). As shown in
Fig. 4b, the amount of water decreased after the incorporation of HMC onto the Fe
3O
4/SiO
2, indicating that the HMC has been successfully immobilized onto the Fe
3O
4/SiO
2. The TGA curve of MHMC shows a weight loss over the range of 90–160 °C and 250–650 °C which is similar to the TGA curve of Fe
3O
4/SiO
2. The total weight losses are approximately 12.5% (
Fig. 4b). The loading of HMC is 0.21 mmol g
−1 based on the difference in the weight losses for Fe
3O
4/SiO
2 and MHMC.
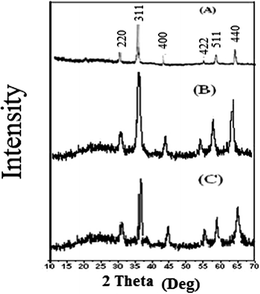 |
| Fig. 2 XRD pattern of (A) Fe3O4, (B) Fe3O4/SiO2 and (C) MHMC. | |
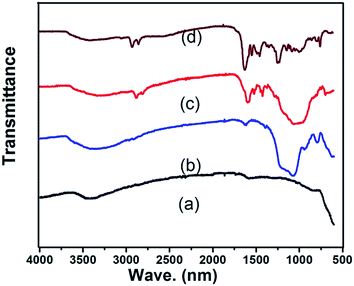 |
| Fig. 3 FT-IR spectra of fresh (a) Fe3O4 (b) Fe3O4/SiO2 (c) MHMC and (d) HMC. | |
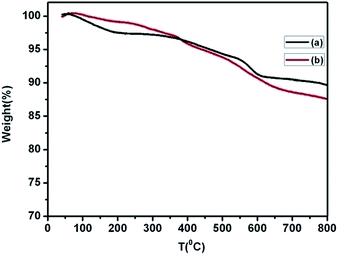 |
| Fig. 4 Thermogravimetric weight loss pattern of Fe3O4/SiO2NPs (a) and MHMC (b) with temperature raised of 10 °C min−1. | |
The TEM images of Fe3O4 and Fe3O4/SiO2 NPs are shown in Fig. 5(a) and (b). The results showed the average product size of Fe3O4 and Fe3O4/SiO2 NPs 15 and 20 nm similar to the results of XRD patterns. The TEM images of Fe3O4/SiO2 NPs indicate the successful coating of magnetic Fe3O4 NPs (Fig. 5b). Fig. 5c shows the TEM image of MHMC which assigned successful coating of MHC on the Fe3O4/SiO2 NPs.
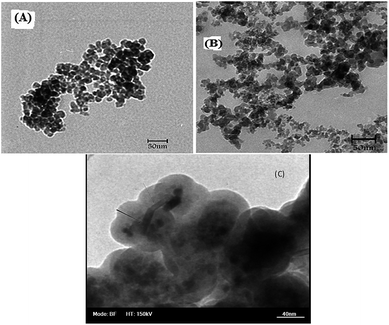 |
| Fig. 5 TEM image of Fe3O4 (a), TEM image of Fe3O4/SiO2 (b) and TEM image of MHMC (c). | |
The BET specific surface area of the MHMC which is determined at room temperature by nitrogen physisorption was 18.5 m2 g−1. The loading of HMC (B) in the MHMC was 0.18 mmol g−1 based on Mn-element analysis by AAS.
Catalytic activity studies
Asymmetric epoxidation of unfunctionalized olefins. The catalytic activity of MHMC was investigated for the asymmetric epoxidiaton of unfunctionalized olefins. In an initial attempt, the reaction of α-methylstyrene as a model substrate with TBHP as an oxidant and PNO as an additive was performed at 0 °C in the presence of MHMC nanoparticle in dichloromethane (DCM) media and the effects of different solvents, additives and the amount of catalyst were studied for this reaction. In DCM media, the Lewis acidity of Mn(III) center, which is withdraw electron from the peroxidic oxygen and susceptible to be attacked by nucleophilic olefin is one of the most important characteristics that determines catalytic performance in the olefin epoxidation. In order to elucidate the role of the MHMC catalyst, at first, the reaction was performed in the absence of catalyst and only 8% yield was obtained (Table 1, entry 1). Also, the reaction was performed in the absence of additive pyridine N-oxide (PNO) and an obvious decrease in the yield (75%) and enantioselectivity (50%) were observed (not listed). To optimize the amount of catalyst, the reaction was carried out in the presence of different amount of MHMC (0.4–2.8 mol% based on Mn element) at 0 °C in 3 ml DCM. The best result was achieved by using of 2.4 mol% (based on Mn element) of MHMC (Table 1, entry 7). Decreasing the amount of catalyst resulted in lower yields under the same conditions (Table 1, entries 2–6). On the other hand, higher amounts of catalyst did not improve the yield or reaction time (Table 1, entry 8). For comparison, the homogeneous chiral Mn(III) complex (B) was also investigated under the same reaction conditions, but shortening the reaction time to 3 h (Table 1, entry 9). The catalytic activity of the Fe3O4 and Fe3O4/SiO2 NPs was also investigated in the epoxidation of α-methylstyrene with TBHP as an oxidant and low yields were observed (30 and 45%, respectively). Blank experiment showed that Fe3O4, Fe3O4/SiO2 and MHMC alone are inactive towards the α-methylstyrene epoxidation.
Table 1 Optimization of different amount of MHMC nanocatalyst on the reaction of α-methylstyrene with TBHP as a model reactiona
Entry |
Catalyst amount (mol%) |
Yieldb (%) |
Reaction conditions: substrate (1 mmol), DCM (3 ml), oxidant (2 mmol) and MHMC (2.4 mol% based on Mn) at 0 °C and duration 6 h. Determined by GC with a Shimadzu CBP5 column (30 m × 0.32 mm × 0.25 mm). |
1 |
0 |
7 |
2 |
0.4 |
20 |
3 |
0.8 |
32 |
4 |
1.2 |
47 |
5 |
1.6 |
63 |
6 |
2.0 |
81 |
7 |
2.4 |
97 |
8 |
2.8 |
98 |
9 |
Homogeneous chiral Mn(III) complex (2.4 mol%) |
98 |
Moreover, the effect of different solvents was investigated in the reaction under the same conditions. It seems that, the polarity of solvent has a great influence on catalytic activity of MHMC catalyst (Table 2). By decreasing the polarity of solvents from water to CHCl3, the yields were increased from 15 to 50% (Table 2, entries 1–7). However, the excellent yield of the product was observed in DCM (Table 2, entry 9).
Table 2 The effect of different solvents on the reaction of α-methylstyrene with TBHP catalyzed by MHMC as a model reactiona
Entry |
Solvent |
Catalyst amount (mol%) |
Yieldb (%) |
Reaction conditions: substrate (1 mmol), oxidant (2 mmol) and MHMC (2.4 mol% based on Mn) at 0 °C and duration 6 h. Determined by GC with a Shimadzu CBP5 column (30 m × 0.32 mm × 0.25 mm). |
1 |
H2O |
2.4 |
15 |
2 |
EtOH–H2O |
2.4 |
20 |
3 |
MeOH |
2.4 |
24 |
4 |
EtOH |
2.4 |
30 |
5 |
THF |
2.4 |
35 |
6 |
CHCl3 |
2.4 |
50 |
7 |
CH3CN |
2.4 |
60 |
8 |
EtOAc |
2.4 |
75 |
9 |
DCM |
2.4 |
98 |
The effect of different oxidants/additives in the epoxidation of α-methylstyrene. The effect of various oxidants such as NaIO4, m-CPBA, urea hydrogen peroxide (UHP), H2O2, oxone, PhIO, PhI(OAc)2 and TBHP was investigated in the epoxidation of α-methylstyrene. As can be seen from Table 3, when the reaction was carried out in the absence of oxidant, no yield was observed even after 12 h (Table 3, entry 1). This result showed the efficiency of oxidant in the epoxidation of alkenes. As shown in Table 3, oxidants such as UHP, H2O2, PhIO, PhI(OAc)2 gave low yields and enantioselectivity (Table 3, entries 2–5), while oxone and NaIO4 (For NaIO4, the reaction was carried out in the 2
:
1 mixture of acetonitrile
:
water) gave better yields and enantioselectivity (Table 3, entries 6 and 7). Finally, m-CPBA and TBHP gave excellent yield, but TBHP showed better enantioselectivity (Table 3, entries 8 and 9, respectively). In addition, different equivalents of the oxidant were tested, and the best catalytic activity was obtained with 2 equivalents of the oxidant which provides moderate source of oxygen for the catalytic reaction (not listed). Consequently, the optimum molar ratio of olefin to oxidant is 1
:
2.
Table 3 The effect of different oxidants on the asymmetric epoxidation of α-methylstyrene catalyzed by MHMCa
Entry |
Oxidant |
Yield (%) |
eeb (%) |
Reaction conditions: α-methylstyrene (1 mmol), oxidant (2 mmol), NMNO (0.5 mmol) and MHMC (2.4 mol% based on Mn) at 0 °C and duration 6 h. Determined by GC with chiral column (HP 19091G-B213, 30 m × 0.25 mm × 0.25 μm). |
1 |
— |
0 |
0 |
2 |
H2O2 |
20 |
50 |
3 |
PhIO |
25 |
51 |
4 |
PhI(OAc)2 |
22 |
60 |
5 |
UHP |
35 |
55 |
6 |
NaIO4 |
50 |
55 |
7 |
Oxone |
65 |
57 |
8 |
TBHP |
97 |
80 |
9 |
m-CPBA |
98 |
60 |
The choice of additives is crucially important in the asymmetric epoxidation of olefins. The effect of various additives such as NMNO, PNO, NH4OAc, MI, Py and imidazole was investigated in the epoxidation of α-methylstyrene in DCM/TBHP system (Table 4). Usually, additive such as N-methylmorpholine N-oxide (NMNO), bind to the Mn(III) center prior to the epoxidation reaction, reported to improve epoxidation yields and enentioselectivity and our finding is in accordance with those reported in literature.36 However, some study reported that NMO, decrease the catalytic activities.37 The results showed that higher conversion and ee were observed in the presence of NMNO (Table 4, entry 6). When NH4OAc is used in DCM, low yield (13%) with only 30% ee is obtained (Table 4, entry 1). Under optimized conditions, the reaction was carried out in the presence of homogeneous chiral Mn(III) complex (B) and α-methylstyrene is converted to corresponding epoxide in less than 2.5 h with 97% yield and 20% enentioselectivity (not listed). Nevertheless, homogeneous chiral Mn(III) complex (B) showed higher activity than the corresponding heterogeneous analogues, lower enentioselectivity was observed. The higher enentioselectivity in heterogeneous catalyst may also be attributed to the microenvironment effect and confinement the effect.38
Table 4 The effect of different additives on the asymmetric epoxidation of α-methylstyrene catalyzed by MHMCa
Entry |
Additive |
Yield (%) |
eeb (%) |
Reaction conditions: α-methylstyrene (1 mmol), TBHP (2 mmol), additive (0.5 mmol) and MHMC (2.4 mol% based on Mn) at 0 °C. Determined by GC with chiral column (HP 19091G-B213, 30 m × 0.25 mm × 0.25 μm). |
1 |
NH4OAc |
13 |
30 |
2 |
Py |
50 |
50 |
3 |
MI |
60 |
64 |
4 |
Imidazole |
75 |
66 |
5 |
PNO |
97 |
70 |
6 |
NMNO |
97 |
80 |
The reactions were also performed at different temperatures (−40, 40 and 60 °C). Under the same conditions, an obvious decrease in the conversion of the reaction (65%) was observed at −40 °C, but enantioselectivity did not improve. At 25 °C, the reaction was performed in faster rates (3.5 h) and higher TOF (17.75–32.74 h−1) with any drop in the selectivity of product. Despite better yield and faster reaction rate at high temperature (40 and 60 °C), obvious decreasing in enantioselectivity was observed. Therefore, we employed the optimized conditions (MHMC, 2.4 mol%), TBHP (2 mmol) and NMNO (0.5 mmol) in 3 ml DCM at 0 °C for the conversion of several olefins into the corresponding products. Table 5 lists a group of alkenes that were investigated by MHMC. The catalyst showed excellent activity toward olefins oxidation with an average isolation yield of 95%. Conditions used: injector temperature, 220 °C; detector temperature, 250 °C, injection volume, 0.2 μl, and column, isothermal 90 °C for styrene and 80 °C for α-methylstyrene.
Table 5 Epoxidation of different olefins using TBHP catalyzed by MHMCa
Entry |
Alkene |
T (°C) |
Time (h) |
Conversion (%) |
Selectivityb (%) |
Yieldc (%) |
eed (%) |
TONe |
TOFf × 10−4 (S−1) |
Reaction conditions: substrate (1 mmol), DCM (3 ml), oxidant (2 mmol), NMNO (0.5 mmol) and MHMC (2.4 mol% based on Mn). Selectivity based on alkenes conversion. Determined by GC with a Shimadzu CBP5 column (30 m × 0.32 mm × 0.25 mm). ee% was performed by GC with a Agilent HP column (19091G-B213, 30 m × 0.25 mm × 0.25 μm). %ee = [A(major enantiomer) − A(minor enantiomer)] × 100/[A(major enantiomer) + A(minor enantiomer)]. TON = mmol of converted alkene/mmol Mn. TOF = TON/time of reaction. |
1 |
 |
0 |
6 |
99 |
100 |
98 |
80 |
41.25 |
19.10 |
25 |
3.5 |
99 |
99 |
98 |
80 |
41.25 |
32.74 |
2 |
 |
0 |
6 |
99 |
100 |
97 |
70 |
41.25 |
19.10 |
25 |
3.5 |
99 |
100 |
96 |
68 |
41.25 |
32.74 |
3 |
 |
0 |
6 |
95 |
>99 |
94 |
75 |
39.58 |
18.33 |
25 |
4 |
96 |
99 |
95 |
74 |
40 |
27.78 |
4 |
 |
0 |
6 |
95 |
99 |
94 |
65 |
39.58 |
18.33 |
25 |
4 |
97 |
99 |
95 |
64 |
40.42 |
28.07 |
5 |
 |
0 |
6 |
96 |
>99 |
95 |
69 |
40 |
18.52 |
25 |
3.5 |
98 |
99 |
96 |
68 |
40.83 |
32.41 |
6 |
 |
0 |
6 |
94 |
>99 |
92 |
45 |
39.17 |
18.13 |
25 |
3.5 |
95 |
99 |
93 |
44 |
39.58 |
31.42 |
7 |
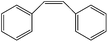 |
0 |
6 |
93 |
100 |
92 |
80 |
38.75 |
17.94 |
25 |
3.5 |
95 |
100 |
94 |
80 |
39.58 |
31.42 |
8 |
 |
0 |
6 |
92 |
100 |
91 |
81 |
38.33 |
17.75 |
25 |
4 |
94 |
100 |
93 |
80 |
39.17 |
27.20 |
9 |
 |
0 |
6 |
96 |
>99 |
95 |
45 |
40 |
18.52 |
25 |
4 |
98 |
>99 |
96 |
44 |
40.83 |
28.36 |
Oxidation of sulfides to sulfoxides
Initially, the oxidation of thioanisole was selected as a model reaction in water with different oxidants (TBHP, UHP & H2O2) in the presence of MHMC at room temperature (Scheme 2). The results showed that H2O2 is the best oxidant for oxidation of sulfides as higher conversion was obtained.
 |
| Scheme 2 Catalytic oxidation of sulfides. | |
Also, different amounts of catalyst were tested and the results showed that the yield and selectivity of the reaction were affected crucially by the amount of catalyst (Table 6). As the results shown, methyl phenyl sulfioxide was achieved with excellent yield in the presence of 0.003 mmol catalyst within 1 h using 2.0 eq. H2O2. Increasing the amount of catalyst and the time of the reaction leads to the further oxidation of sulfoxide to sulfone. Sulfone's selectivity increased significantly and reached its maximum to 99% in the presence of 0.006 mmol catalyst within 3 h. It should be noted that, in the absence of catalyst no significant sulfoxidation was observed under similar reaction conditions, and only a low conversion was observed in the presence of 2 eq. of H2O2 after prolonged reaction time (Table 6, entry 7). Also, various H2O2/substrate molar ratios were studied for the investigation of the effect of oxidant on the yield and selectivity of product in the presence of 0.003 mmol catalyst. Various amounts of H2O2 affected the conversion of products and the best conversion and selectivity was achieved with 2 eq. H2O2. Increasing the molar ration of H2O2 leads to a decrease in the selectivity of corresponding sulfoxide, but H2O2 has less effect compared to the catalyst.
Table 6 The effect of varying H2O2/catalyst amounts and time on the oxidation of thioanisole catalyzed by MHMC in water
Entry |
H2O2 (mmol) |
Procedure Aa (0.003 mmol catalyst) |
Procedure Bb (0.006 mmol catalyst) |
Sulfoxidec (%) |
Sulfonec (%) |
Sulfoxidec (%) |
Sulfonec (%) |
Reactions were run under air at room temperature for 1 h using 1.0 mmol thioanisole. Reactions were run under air at room temperature for 3 h using 1.0 mmol thioanisole. GC yields based on the toluene as the internal standard. The reaction was carried out in the absence of MHMC for 6 h. |
1 |
1 |
50 |
0 |
20 |
40 |
2 |
1.5 |
78 |
0 |
10 |
55 |
3 |
2 |
97 |
3 |
0 |
99 |
4 |
2.5 |
88 |
12 |
0 |
99 |
5 |
3 |
75 |
25 |
0 |
100 |
6 |
3.5 |
65 |
35 |
0 |
100 |
7d |
2 |
10 |
— |
|
|
The effect of different solvents (water, EtOAc, CHCl3, THF, DCM, CH3CN, EtOH, MeOH and EtOH–water (1
:
1)) was also investigated and results showed that water is the best solvent in the term of conversion and selectivity. The effect of different PH (2, 3, 4, 5, 6, 7, 8, 9 and 10) was also studied on the conversion and selectivity of products and no obvious changes in the conversion and selectivity were observed (not listed). Moreover, the reaction was carried out in the presence of homogeneous chiral Mn(III) complex (B) and methyl phenyl sulfide is converted to corresponding sulfoxide in 35 min with 97% yield (not listed).
Under optimized conditions, the applicability of catalyst was tested for oxidation of various sulfides (Table 7). Several sulfides undergo selective oxidation to produce the corresponding sulfoxides/sulfones. As the results shown in Table 7, all substrates could be converted to sulfoxides (procedure A) and sulfones (procedure B) with high conversion rates, and excellent selectivities were obtained under mild conditions. Under given conditions, the catalytic oxidation of sulfides to corresponding sulfoxides showed excellent efficiency in the terms of conversion (91–99%), TOF (310–330 h−1) and selectivity (99–100). Furthermore, the presence of either electron withdrawing or releasing groups, present in the phenyl ring of sulfides did not affect the synthesis of sulfoxide considerably (Table 7, entries 3–6).
Table 7 Oxidation of different sulfides using H2O2 catalyzed by MHMCa
Entry |
Substrate |
Sulfoxidea |
Sulfoneb |
Convc.c (%) |
Select. (%) |
TOFd (h−1) |
Convc.c (%) |
Select. (%) |
TOFd (h−1) |
Reaction conditions: sulfide (1 mmol), 30% H2O2 (2 equiv.), catalyst (0.003 mmol based on Mn element), 1 h, rt. Reaction conditions: sulfide (1 mmol), 30% H2O2 (2 equiv.), catalyst (0.006 mmol based on Mn element), 3 h, rt. Conversion and selectivity were determined by GC, conversion = [(initial moles of substrate − final moles of substrate)/initial moles of substrate] × 100, selectivity to sulfoxide = [sulfoxide%/(sulfoxide% + sulfone%)] × 100. TOF = number of moles of substrate converted per mole of catalyst per hour. |
1 |
 |
98 |
100 |
330 |
99 |
100 |
55 |
2 |
 |
90 |
100 |
310 |
91 |
99 |
50.6 |
3 |
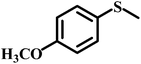 |
98 |
100 |
323 |
97 |
100 |
54 |
4 |
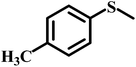 |
99 |
99 |
326 |
99 |
99 |
55 |
5 |
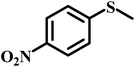 |
96 |
99 |
323 |
95 |
99 |
52.8 |
6 |
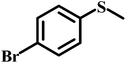 |
97 |
99 |
320 |
94 |
99 |
52.2 |
7 |
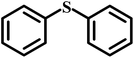 |
98 |
100 |
316 |
97 |
100 |
53.8 |
8 |
 |
96 |
99 |
320 |
97 |
100 |
53.8 |
We also investigated the possibility of reusing of recovered catalyst for new oxidation reactions. Therefore, the MHMC catalyst was separated by external magnet, washed with EtOH, dried and reused directly for a subsequent round of reaction without further purification with no significant loss of activity which validates its recyclability. In each run, no manganese was detected in the filtrates by AAS. As shown in Fig. 6, it is obvious that the catalyst worked well for asymmetric epoxidation of α-methylstyrene up to five cycles with no considerable decrease in reactivity and enantioselectivity (yield: from 97% to 92%; ee: from excess 80–77%). The decreases in the activity and enantioselectivity of products maybe due to the leaching of silica or the aggregation of Fe3O4. Also, the reusability of MHMC was also investigated for the oxidation of methyl phenyl sulfide and no obvious decrease in yields was observed (Fig. 6).
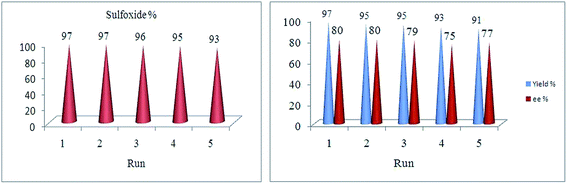 |
| Fig. 6 The recycle of the catalyst for oxidation of thioanisol (left) using H2O2 (2 mmol) in water (2 ml) catalyzed by MHMC (0.003 mmol) at room temperature and asymmetric epoxidation of α-methylstyrene (right), TBHP (2 mmol) and NMNO (0.25 mmol) in 3 ml DCM catalyzed by MHMC (2.4 mol% based on Mn) at 0 °C. | |
Conclusions
In summary, the novel type of MHMC was synthesized by grafting of the HMC onto Fe3O4/SiO2 NPs. The new MHMC showed high catalytic activity in the oxidation of organic sulfides and epoxidation of simple alkenes. In the epoxidation reactions, the influences of oxidants and additives with considering yields and enantioselectivity on the activity of MHMC were explored at length and TBHP and NMNO were chosen as the best oxidant and additives, respectively. The MHMC exhibited comparable enantioselectivity than that of homogeneous counterpart for the asymmetric epoxidation of several alkenes. The MHMC is an efficient nanocatalyst for environmentally friendly oxidation of various sulfides into both sulfoxides and sulfones at room temperature in water using 30% H2O2. In addition, no side reactions, short reaction times, mild reaction conditions, easy and simple work up and excellent selectivity and yields are some advantages of this nanocatalyst. Moreover, the catalyst is cheap, easily synthesized and recovered by external magnetic field and reused without any noticeable loss of activity after several times, thus indicating that the complexes are stable, which prevents leaching of the active phase and its deactivation.
Experimental and methods
Materials
Tetraethoxysilane (TEOS), FeCl3·6H2O, FeCl2·4H2O, styrene, 4-chlorostyrene, cyclohexene, α-methyl styrene, indene, cis & trans stilbene, cyclooctene, 1-octene, m-chloroperbenzoic acid (m-CPBA), pyridine N-oxide (PNO), pyridine (Py), N-methylmorpholine N-oxide (NMNO), 1-methyl imidazole (MI), imidazole (IM), dichloromethane (DCM), ethyl acetate (EtOAc), toluene, ethanol (EtOH), CH3CN, CHCl3, THF, NaIO4, NH4OAc, TBHP, PhI (OAc)2, H2O2 (30%), (R,R)-1,2-cyclohexanediamine and oxone were purchased from Merck Company and used without purification.
Measurements
The resulting Fe3O4/SiO2 and MHMC were characterized by IR, TEM, TGA, VSM and XRD patterns. The IR experiments were carried out on a Perkin-Elmer 783 Infrared spectrophotometer in a KBr pellet, scanning from 4000 to 600 cm−1 at room temperature. The XRD measurements were carried out by using a Bruker D8-advance X-ray diffractometer with Cu Kα radiation (k = 1.5406 Å). The transmission electron microscopy (TEM) images measurement were obtained using Philips CM10 instrument. Magnetization measurements were carried out at 300 K on a vibrating sample magnetometer (VSM Leak shore 7200). Thermogravimetric analysis (TGA) was performed by heating the samples in an Argon flow at a rate of 100 ml min−1 using a Perkin-Elmer Diamond TG/DTA thermal analyzer with a heating rate of 10 °C min−1. The conversion of products was determined by GC-17A Shimadzu with capillary column (Shimadzu, CBP5, 30 m× 25 mm× 0.25 μm). The ee was determined in a chiral GC column (HP 19091G-B213, 30 m × 0.25 mm × 0.25 μm).
Synthesis of MHMC (C)
The black magnetic Fe3O4 NPs,39 Fe3O4/SiO2 NPs,40 (R,R)-N,N′-bis(2,4-di-hydroxybenzaldehyde)-1,2-cyclohexanediamine41 and Mn(III) salen complex42 were prepared as described in literature. The synthesized homogeneous Mn(III) salen complex (B) has been chemically anchored to the Fe3O4/SiO2 (A). Briefly, Fe3O4/SiO2 (2 g) was dispersed in 100 ml dry toluene for 20 min. Then, 0.5 g of homogeneous Mn(III) complex was added to the stirring mixture (Scheme 1). The resulting suspension was refluxed for 24 h under inert atmosphere. After the completion of the reaction, the MHMC (C) was washed with dry toluene, EtOH and extracted repeatedly on a Soxhlet extractor with methanol and dichloromethane until the washing becomes colorless. The solid was dried at 70 °C under vacuum for 6 h. The MHMC was characterized by XRD, TEM, FT-IR, BET, VSM and TGA.
General procedure for the asymmetric epoxidation of alkenes
In a typical procedure, MHMC as catalyst (2.4 mol% based on Mn element) was dispersed in 3 ml of dichloromethane for 20 min and cooled to 0 °C followed by addition of α-methylstyrene as substrate (1 mmol), toluene (internal standard, 40 μl), TBHP (2 mmol) as oxidant and NMNO (0.5 mmol) as additive. The mixture was magnetically stirred at 0 °C for appropriate times. The progress of the reaction was monitored by TLC. After the completion of the reaction, the catalyst was separated by external magnet. The solution was washed with 1 M NaOH (8 ml) and brine (8 ml) and dried over MgSO4. Then, the solution was concentrated by rotary evaporator to 1 ml. Finally, the conversion and ee values of products were determined by GC. The catalyst was washed twice with ethanol and reused.
General procedure for the oxidation of sulfides to sulfoxides/sulfones
An appropriate amount of MHMC as catalyst (0.003 & 0.006 mmol based on Mn element for sulfoxide and sulfone, respectively) was ultrasonicated for 20 min in water (2 ml). Then, sulfide (1.0 mmol), toluene (40 μl, as the internal standard) and hydrogen peroxide (2.0 mmol 30%, 200 μl) were added to the mixture and the mixture was stirred at room temperature for the required time (Scheme 2). The progress of the reaction was monitored by TLC. After the completion of the reaction, the product was extracted with ethyl acetate, washed with brine, dried over anhydrous Na2SO4, and the yield of products was determined by GC analysis using toluene as the internal standard. The heterogeneous catalyst was washed with EtOH, dried and used for the next runs.
Acknowledgements
The authors are grateful to the University of Birjand for financial support.
References
- L. Canali and D. C. Sherrington, Chem. Soc. Rev., 1999, 28, 85–93 RSC.
- M. J. Sabater, A. Corma, A. Domenech, V. Fornes and H. Garcia, Chem. Commun., 1997, 1285–1286 RSC.
- X.-G. Zhou, X.-Q. Yu, J.-S. Huang, S.-G. Li, L.-S. Li and C.-M. Che, Chem. Commun., 1999, 1789–1790 RSC.
- G.-J. Kim and J.-H. Shin, Tetrahedron Lett., 1999, 40, 6827–6830 CrossRef CAS.
- L. Frunza, H. Kosslick, H. Landmesser, E. Hoft and R. Fricke, J. Mol. Catal. A: Chem., 1997, 123, 179–187 CrossRef CAS.
- C. Li, H. Zhang, D. Jiang and Q. Yang, Chem. Commun., 2007, 547–558 RSC.
- W. Zhang, J. L. Loebach, S. R. Wilson and E. N. Jacobsen, J. Am. Chem. Soc., 1990, 112, 2801–2803 CrossRef CAS.
- W. Zhang and E. N. Jacobsen, J. Org. Chem., 1991, 56, 2296–2298 CrossRef CAS.
- H. Zhang, Y. M. Wang, L. Zhang, G. Gerritsen, H. C. L. Abbenhuis, R. A. Van Santen and C. Li, J. Catal., 2008, 256, 226–236 CrossRef CAS PubMed.
- R. I. Kureshy, I. Ahmad, N. H. Khan, S. H. R. Abdi, K. Pathak and R. V. Jasra, J. Catal., 2006, 238, 134–141 CrossRef CAS PubMed.
- R. I. Kureshy, I. Ahmad, N. H. Khan, S. H. R. Abdi, K. Pathak and R. V. Jasra, Tetrahedron: Asymmetry, 2005, 16, 3562–3569 CrossRef CAS PubMed.
- H. Zhang, S. Xiang, J. Xiao and C. Li, J. Mol. Catal. A: Chem., 2005, 238, 175–184 CrossRef CAS PubMed.
- V. Mirkhani, M. Moghadam, S. Tangestaninejad, B. Bahramian and A. Mallekpoor- Shalamzari, Appl. Catal., A, 2007, 321, 49–57 CrossRef CAS PubMed.
- K. Smith and C. H. Liu, Chem. Commun., 2002, 886–887 RSC.
- X. C. Zou, X. K. Fu, Y. D. Li, X. B. Tu, S. D. Fu, Y. F. Luo and X. Wu, Adv. Synth. Catal., 2010, 352, 163–170 CrossRef CAS.
- N. E. Leadbeater and M. Marco, Chem. Rev., 2002, 102, 3217–3273 CrossRef CAS PubMed.
- Q.-H. Fan, Y.-M. Li and A. S. C. Chan, Chem. Rev., 2002, 102, 3385–3466 CrossRef CAS PubMed.
- Q.-H. Xia, H.-Q. Ge, C.-P. Ye, Z.-M. Liu and K.-X. Su, Chem. Rev., 2005, 105, 1603–1662 CrossRef CAS PubMed.
- C. Li, Catal. Rev., 2004, 46, 419–492 CAS.
- M. B. Gawande, P. S. Branco and R. S. Varma, Chem. Soc. Rev., 2013, 42, 3371–3393 RSC.
- R. G. Chaudhuri and S. Paria, Chem. Rev., 2012, 112, 2373–2433 CrossRef PubMed.
- L. Guerrini and D. Graham, Chem. Soc. Rev., 2012, 41, 7085–7107 RSC.
- L. H. Reddy, J. L. Arias, J. Nicolas and P. Couvreur, Chem. Rev., 2012, 112, 5818–5878 CrossRef CAS PubMed.
- V. Polshettiwar, R. Luque, A. Fihri, H. Zhu, M. Bouhrara and J.-M. Basset, Chem. Rev., 2011, 111, 3036–3075 CrossRef CAS PubMed.
- J. E. Backvall, Modern Oxidation Methods, Wiley-VCH, Weinheim, Germany, 2nd edn, 2010 Search PubMed.
- J. Drabowski, P. Kielbasinski and M. Mikolajcyk, Synthesis of Sulfoxides, John Wiley and Sons, New York, 1994 Search PubMed.
- A.-N. R. Alba, X. Companyó and R. Rios, Chem. Soc. Rev., 2010, 39, 2018–2033 RSC.
- O. O. Shyshkina, K. S. Popov, O. O. Gordivska, T. M. Tkachuk, N. V. Kovalenko, T. A. Volovnenko and Y. M. Volovenko, Chem. Heterocycl. Compd., 2011, 47, 923–945 CrossRef CAS.
- R. Ettari, C. Bonaccorso, N. Micale, C. Heindl, T. Schirmeister, M. L. Calabrò and M. Zappalà, ChemMedChem, 2011, 6, 1228–1237 CrossRef CAS PubMed.
- Q. H. Fan, Y. M. Li and A. S. C. Chan, Chem. Rev., 2002, 102, 3385–3465 CrossRef CAS PubMed.
- W. Zhang, J. L. Loebach, S. R. Wilson and E. N. Jacobsen, J. Am. Chem. Soc., 1990, 112, 2801–2803 CrossRef CAS.
- W. Zhang and E. N. Jacobsen, J. Org. Chem., 1991, 56, 2296–2298 CrossRef CAS.
- M. A. Nasseri, A. Allahresani and A. A. Esmaeili, Lett. Org. Chem., 2014, 11, 91–96 CrossRef CAS.
- M. A. Nasseri, A. Allahresani and H. Raissi, Iran. J. Catal., 2014, 4, 33–40 CAS.
- M. A. Nasseri, A. Allahresani and H. Raissi, RSC Adv., 2014, 4, 26087 RSC.
- K. A. Campbell, M. R. Lashley, J. K. Wyatt, M. H. Nantz and R. D. Britt, J. Am. Chem. Soc., 2001, 123, 5710–5719 CrossRef CAS PubMed.
- Y. Yang, S. Hao, Y. Zhang and Q. Kan, Solid State Sci., 2011, 13, 1938–1942 CrossRef CAS PubMed.
- R. F. Bai, X. K. Fu, H. B. Bao and W. S. Ren, Catal. Commun., 2008, 9, 1588–1594 CrossRef CAS PubMed.
- Y. Liu and L. Jia, Microchem. J., 2008, 89, 72–76 CrossRef CAS PubMed.
- C. Wu, H. He, H. Gao, G. Liu, R. Ma, Y. An and L. Shi, Sci. China: Chem., 2010, 53, 514 CrossRef CAS PubMed.
- J. Lopez, S. Liang and X. R. Bu, Tetrahedron Lett., 1998, 39, 4199–4202 CrossRef CAS.
- H. Vezin, E. Lamour and J. P. Catteau, J. Inorg. Biochem., 2002, 92, 177–182 CrossRef CAS.
|
This journal is © The Royal Society of Chemistry 2014 |
Click here to see how this site uses Cookies. View our privacy policy here.