DOI:
10.1039/C4RA09784J
(Paper)
RSC Adv., 2014,
4, 58108-58115
Novel cold temperature active β-glucosidase from Pseudomonas lutea BG8 suitable for simultaneous saccharification and fermentation†
Received
4th September 2014
, Accepted 29th October 2014
First published on 30th October 2014
Abstract
Cold tolerant microbes play a pivotal role in the decomposition of cellulosic biomass in temperate environments. This study was aimed to evaluate β-glucosidase production from psychrotolerant microorganisms isolated from cold desert soil of the Kargil district, Himalayan region, India. A total of 12 morphotypes were isolated at 4 °C and, based on qualitative screening, seven isolates were selected for molecular identification and phenotypic microarray. Pseudomonas lutea BG8 was selected based on its β-glucosidase production potential. The characterization of β-glucosidase revealed the alkali-tolerant nature of the enzyme with a temperature optimum at 40 °C and ability to retain considerable activity over a broad pH range (5–10). The enzyme retained 95.15% activity even in the presence of 1000 mM glucose, indicating an absence of feedback inhibition and high glucose tolerance. The enzyme stability experiment revealed that the β-glucosidase enzyme retained 49.96% activity at pH 5 and 30 °C after 16 h of incubation. The Km and kcat of the β-glucosidase enzyme were found to be 0.636 ± 0.103 mM and 102.7 ± 0.004 s−1, respectively. The cold temperature active β-glucosidase from P. lutea BG8 was found to be highly efficient for bioconversion of cellobiose to ethanol along with S. cerevisiae by simultaneous saccharification and fermentation process with 91.42% (0.49 g ethanol per g cellobiose) fermentation efficiency at 4 °C. This novel β-glucosidase can lead to considerable energy savings due to its lower temperature optimum, as compared to commercial β-glucosidase.
Introduction
Cellulose is the most abundant (approx. 100 billion tons produced per year) and renewable carbohydrate in the biosphere which offers significant opportunities for generation of sustainable energy supplies through its bioconversion.1 Microbial cellulose degradation by cellulases and cellulosomes, represents a major carbon flow from fixed carbon sinks to atmospheric CO2.2 β-glucosidase (β-glucoside glucohydrolase) is an indispensable component of the cellulase multienzyme complex since it hydrolyzes the end dimer “cellobiose”, which is a key rate limiting step in the entire cellulose hydrolysis process.3
The Earth is a cold planet as snow covers three-quarters of the surface. About 85% of the biosphere is permanently exposed to temperatures below 5 °C. These environments extend from the Arctic, Antarctic, high-mountains to the deep ocean, and are colonized by a diverse group of microorganisms. Many studies have documented the isolation, selection and screening of psychrophilic/psychrotolerant microbial resources.4 Microbial diversity of such cold niches has grown in significance because of their unusual adaptation mechanisms including secretion and/or accumulation of enzymes with potential biotechnological applications.5 The role of hydrolytic enzymes especially β-glucosidase has been highlighted previously for cold adaption as it is directly correlated with the carbon efflux in such extreme environment.6 However, only few attempts have been made to study and evaluate cold active β-glucosidase enzymes7,8 and only limited information is available regarding its application. This prompted us to explore Himalayan cold desert of India for the efficient β-glucosidase producing cold tolerant microorganisms.
In many commercially available cellulase enzyme preparations, β-glucosidase enzyme is not effective in cellulosic hydrolysis, resulting in the accumulation of cellodextrins (cellobiose and cello-oligosaccharides) and slow release of monomeric sugars. β-Glucosidases also undergo product inhibition by glucose during hydrolysis.9 This end product inhibition can be substantially reduced in simultaneous saccharification and fermentation (SSF) process. However, unmatched incubation temperature requirements for saccharification (approx. 45–55 °C) and fermentation (approx. 30–35 °C) is a major bottleneck for this process. To overcome this obstacle, β-glucosidases from different origin have been cloned and expressed in yeast and other microbes for SSF, but its production is limited due to low efficiency.10 Therefore, efforts are needed towards exploring novel β-glucosidase to cope up with these problems.
The objectives of the current study were to explore the β-glucosidase producing microbial diversity from Kargil, a high altitude cold desert region of Himalaya (India). Further characterization of cold active β-glucosidase enzyme from the most promising isolate was undertaken to assess its cellobiose hydrolysis potential and application in the SSF process for ethanol production.
Experimental
Sample collection, enrichment and isolation of microorganisms
Soil samples were collected in the month of February (2013) from four different sites of cold desert soil of southeastern Himalayan region, Kargil district, India. The sampling sites were located at 34° 32′ North latitude and 76° 07′ East longitude at an elevation of 10
500 ft above sea level and the temperature ranged from −20 to 5 °C. The samples were kept in the dark, first at 4 °C during transportation and afterwards at −20 °C in the laboratory.
The isolation of psychrotolerant microorganisms was performed by using substrate specific enrichment technique by using a composite soil sample. In brief, 5 g of soil sample was incubated in 100 ml Erlenmeyer flask with 50 ml of Reese's minimal medium (RMM)
:
KH2PO4 2 g; (NH4)2SO4 1.4 g; MgSO4·7H2O 0.3 g; CaCl2·2H2O 0.3 g; FeSO4·7H2O 5 mg; MnSO4·2H2O 1.6 mg; ZnSO4·7H2O 1.4 mg; CoCl2·6H2O 2 mg; Distilled H2O 1000 ml; pH 7.0 ± 0.2 containing 1% carbon source (α-cellulose or cellobiose). The flasks were incubated at 4 °C with 100 rpm shaking for 7 days. After enrichment, isolation was carried out by spread plate technique on solid medium containing α-cellulose and/or cellobiose as a substrate. The plates were incubated at 4 °C for 10–15 days. The growth was scored according to pigmentation, approximate colony shape and size. The isolates representing different morphological characteristics were selected and purified by repeated streaking onto nutrient agar plates. The selected isolates were maintained on the nutrient agar slant and stored at 4 °C for further study.
Estimation of β-glucosidase and enzyme kinetics studies
β-Glucosidase (cellobiase; EC: 3.2.1.21) assay and kinetic parameters was performed using p-nitrophenyl-β-d-glucopyranoside as substrate.11 One unit of enzyme activity was expressed as the amount of enzyme required to release 1 μmol of end product per minute under assay conditions. Protein concentration in the filtrates was determined by the Bradford method using bovine serum albumin (BSA) as a standard.12 The reaction velocity was determined using 0.5–5.0 mM p-nitrophenyl-β-d-glucopyranoside in citrate buffer (50 mM) of pH 5.0. The kinetic constant Km and kcat were calculated by a nonlinear regression of Michaelis–Menten equation using GraphPad PRISM version 6.05 (trial version).
Qualitative screening of isolates for β-glucosidase production ability
β-Glucosidase production was tested qualitatively on RMM agar medium containing 0.5% esculin and 0.1% ammonium iron(III) citrate with or without 0.5% glucose as mentioned by Mattéotti et al.13 For inoculation, 2 μl of 48 h grown culture was spotted on esculin plates and incubated at 5 and 30 °C for 48 h. After growth, black zone formation was examined under the long-wave ultraviolet light using Gel Doc EZ Imager (Bio-Rad, Hercules, CA) and β-glucosidase producing strains were selected for further study.
Molecular identification and phylogenetic analysis of selected strains
The β-glucosidase producing strains were identified using standard molecular characterization approach based on ribosomal gene sequencing. Genomic DNA was isolated from selected strains using Zymo Research Fungal/Bacterial DNA MicroPrep™ following the manufacturer's standard protocol. For bacterial isolates, 16S rRNA gene of approximately 1.5 kb internal region was amplified using universal primer set of forward primer pA (5′ AGA GTT TGA TCC TGG CTC AG 3′) and reverse primer pH (5′ AAG GAG GTG ATC CAG CCG CA 3′), corresponding to positions 8–26 and 1504–1522, respectively, in the 16S rRNA gene sequence of Escherichia coli.14 For molecular identification of yeast isolate, the rRNA gene cluster, consisting of ITS-1, the 5.8S rDNA and ITS-2, was amplified with primers homologous to conserved sequences within the small subunit (SSU) rRNA gene. The ITS primers used were ITS-1 (5′ TCC GTA GGT GAA CCT GCG G 3′) and ITS-4 (5′ TCC TCC GCT TAT TGA TAT GC 3′) to amplify fragment of approximately 550 bp. The reaction mixture (100 μl) consisted of 3.5 U of Taq DNA polymerase, 0.2 mM dNTP, 10 μl of 10× PCR Buffer, 0.15 mM of each primer, and 10 ng of template DNA. The PCR program was as follows: initial incubation at 94 °C for 5 min, followed by 35 cycles (94 °C for 50 s, 55 °C for 1 min, and 72 °C for 90 s), and then by a final extension at 72 °C for 10 min. The amplified products were analyzed by electrophoresis at 60 V for 1 h in 1.2% agarose gel in 1× TAE buffer. The PCR products were outsourced to Xcelris Labs Ltd, Ahmedabad, India for DNA sequencing. The partial sequences of the isolates were compared with sequences available by the BLAST search in the National Center for Biotechnology Information (NCBI) database (http://www.nvbi.nlm.nih.gov) to identify the nearest taxa and were submitted to NCBI Genbank.
Multiple sequence alignments were performed using Clustal W version 1.8. To calculate evolutionary distances, phylogenetic dendrogram was constructed by neighbor-joining method based on 16S rRNA gene sequences and tree topologies were evaluated by performing bootstrap analysis of 1000 data sets using MEGA 5.0 (Molecular Evolutionary Genetics Analysis).
Phenotypic microarray analysis of selected strains
All selected strains were analyzed for growth phenotype microarray based on utilization of carbon substrate using the BIOLOG MicroPlates, PM1 and PM2 (Biolog, Inc., Hayward, California, USA). Each 96 well microtiter plate has 95 wells that contain a single carbon substrate with one negative control well without any substrate. Carbon substrate usage was evaluated colorimetrically based on redox technology, with cell respiration (NADH production) as a universal reporter.15 Strains were grown to mid-log phase then pelleted for 10 min at 10
000 rpm at 4 °C. The media supernatant was discarded and the pellets were washed a total of three times by resuspending in saline water (0.8% w/v) to remove nutrient medium, vortexing vigorously, and then pelleting the cells at 10
000 rpm for 10 min. After the final wash, cells were suspended in 15 ml of inoculation fluid (IF-0; Biolog) until a cell density of 85% transmittance was reached on a Biolog turbidimeter. Triplicate plates were used for each isolate and were incubated at 4 °C in sealed plastic bags. After 72 h of incubation, plates were inoculated with 100 μl of Biolog redox dye mix and further incubated at 37 °C until violet colour appeared.
The NTSYSpc version 2.0 was used to compare the similarity for carbon substrate usage across the representative strains. Clustering was based on binary data (usage/nonusage) for the total 190 substrates in PM1 and PM2 plates and dendrogram was constructed using the simple matching (SSM) coefficient measured with UPGMA linkage.
Quantitative screening of selected isolates for β-glucosidase production
Esculin hydrolyzing strains were further screened for their β-glucosidase enzyme production potential. All selected strains were tested using different saccharides (Avicel PH101, Sigmacell 101, α-cellulose and cellobiose). Submerged fermentation was carried out using RMM containing 1% saccharides as a sole carbon source. For inoculation, 1% (v/v) of 48 h grown culture in nutrient broth medium was used. The flasks were incubated under shaking condition (150 rpm) at 5 and 30 °C for 48 h. All the experiments were carried out in triplicate. The crude extracellular enzyme was collected by centrifugation at 10
000 g for 10 min at 4 °C and assayed for activity of β-glucosidase enzyme.
Effect of temperature, pH and glucose concentration on β-glucosidase activity
The crude enzyme preparation was further investigated to study the effect of temperature on β-glucosidase enzyme between 10 to 60 °C. The optimal pH was analyzed by estimating the activities between pH 3.0–10.0, using 50 mM citrate (pH 3.0–6.0), sodium phosphate (pH 6.0–9.0), and glycine NaOH (pH 9.0–10.0) buffers. The inhibitory effect of glucose on β-glucosidase activity was measured by adding different D-glucose concentration (0–1000 mM). For the determination of temperature and pH stability, the enzyme was pre-incubated in the temperature range of 20, 30, 40, 50 and 60 °C for 0–16 h, or in the initial pH range 3–7 at 30 °C, and assayed for β-glucosidase activity.
Cellobiose hydrolysis by extracellular β-glucosidase enzyme
The crude extracellular enzyme by P. lutea BG8 was further evaluated for cellobiose hydrolysis. The crude extracellular enzyme was concentrated using acetone precipitation. The precipitate was dissolved in citrate buffer (50 mM) of pH 5.0 to concentrate by ten-fold. The β-glucosidase enzyme (5 IU ml−1) was added with 2% (w/v) cellobiose in 50 mM citrate buffer (pH 5.0) and incubated at 30 °C for 16 h. Samples were withdrawn at 0, 2, 4, 6, 8 and 16 h. The cellobiose and glucose concentration in the hydrolysate were determined by high performance liquid chromatography (Waters pump 515 model) equipped with Waters 2414 refractive index (RI) detector. The Aminex HPX-87H column was operated with 5 mM H2SO4 as mobile phase at a flow rate of 0.5 ml min−1 and the oven temperature was kept at 45 °C.
Evaluation of β-glucosidase efficiency for simultaneous saccharification and fermentation (SSF) of cellobiose
After quantification of cellobiose hydrolysis efficiency, β-glucosidase enzyme from P. lutea BG8 was further evaluated in a SSF experiment using Saccharomyces cerevisiae LN1.16 The yeast inoculum was raised in MGYP medium containing malt extract (3 g l−1), yeast extract (3 g l−1), peptone (5 g l−1) and glucose (10 g l−1) at pH 6.8 at 30 °C under shaking condition (150 rpm). Fermentation experiment was carried out in screw capped plastic bottles with medium containing KH2PO4 (0.1%), (NH4)2SO4 (0.5%), MgSO4·7H2O (0.5%) and cellobiose (2%) at pH 5.0. Simultaneously, crude β-glucosidase enzyme (5 IU ml−1) from P. lutea BG8 was supplemented with this medium. Yeast cells grown upto mid-exponential phase were used as a primary inoculum (10% v/v) for fermentation step. A similar experiment was also conducted without supplementing the β-glucosidase enzyme to observe the cellobiose hydrolysis efficiency of yeast culture. The bottles were incubated in static condition at 30 °C for 16 h. Samples were collected periodically and reaction was stopped by placing the samples in a water bath for 5 min. The samples were filtered through 0.22 μm syringe filters and HPLC analysis was carried out to estimate cellobiose, glucose and ethanol concentration in fermented broth. All experiments were performed in triplicate. The theoretical ethanol yield (%) or cellobiose conversion (%) was calculated as described by NREL LAP-008 protocol17 by using the following formula:
Where, EtOH: ethanol concentration at the end of the fermentation (mg ml−1); 0.51: Conversion factor for glucose to ethanol based on stoichiometric biochemistry of yeast; 1.052: Converts cellobiose to equivalent glucose; Cellobiose: cellobiose concentration at the beginning of the fermentation (mg ml−1).
Results and discussion
Isolation and identification of psychrotolerant microorganisms
In the present investigation, the research was focused on the isolation of potent psychrotolerant microorganisms capable of producing cold active β-glucosidase from Kargil, a high altitude cold desert of Himalaya (India). Since, cellulolytic enzymes have a temperature optima of 50 °C, the main aim of this study was to prospect for psychrophilic β-glucosidase producers with low temperature optima, to save energy input during saccharification. Many efforts have been made to describe the culturable microbial diversity from different cold deserts.5 Although the culturable approach has its limitations but it is essential to get pure isolates for physiological and biochemical studies. The enrichment with α-cellulose and/or cellobiose was adopted to ascertain the isolation of promising microbes with potentially β-glucosidase production at 4 °C. The total viable count (TVC) of psychrotolerant microbes was very low and ranged from 102 to 103 CFU (colony forming unit) g−1 of soil. A total of 12 isolates were selected based on the colony morphology. Among them, seven isolates were selected as target strains capable of producing β-glucosidase enzyme based on the formation of dark brown zone due to esculin hydrolysis (see ESI Fig. 1†). The hydrolysis was clearly visible in all seven isolates at 30 °C whereas only two isolates (BG6 and BG8) showed the zones at 5 °C. The 16S rRNA gene was successfully amplified (about 1500 bp) from the six isolates, whereas amplification was not observed for one isolate (BG1). The eukaryotic specific primer was used for this isolate to amplify rRNA gene cluster, consisting of ITS-1, the 5.8S rDNA and ITS-2 and the resulting amplification product was about 550 bp. The sequencing of amplified products and NCBI-BLAST search showed that the seven isolates belong to the two different phyla (prokaryote and eukaryote). The prokaryotes comprised five different genera including Bacillus (Bacillus altitudinis BG11, GenBank accession no. KJ997742), Pseudomonas (Pseudomonas graminis BG6, GenBank accession no. KJ997739 and Pseudomonas lutea BG8, GenBank accession no. KJ997740), Pantoea (Pantoea vagans BG5, GenBank accession no. KJ997738), Stenotrophomonas (Stenotrophomonas rhizophila BG9, GenBank accession no. KJ997741) and Arthrobacter (Arthrobacter nitroguajacolicus BG4, GenBank accession no. KJ997737) which lie in three taxonomic divisions: Firmicute, γ-Proteobacteria and Actinomycetales. Molecular identification of selected bacterial isolates revealed the dominance of proteobacteria belonging to genera Pseudomonas, Pantoea and Stenotrophomonas. However, genus Bacillus and Arthrobacter was also isolated. These are some of the common genera known to occur in cold environments.18 Interestingly, one yeast isolate belonging to the Phylum Basidiomycota and genus Holtermanniella (Holtermanniella watticus BG1, GenBank accession no. KJ997743) was also isolated. However, majority of the microorganisms from cold environments belong to the eubacteria and yeasts represent a small proportion of total microbial population, but their role in nutrient recycling through enzymatic reactions is well established.19 The phylogenetic relationship of each isolate with their respective nearest neighbour is presented in Fig. 1.
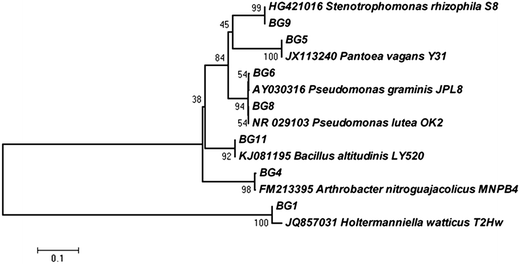 |
| Fig. 1 Phylogenetic trees based on ribosomal gene sequences showing the relationship of all seven strains obtained from Kargil region, with their nearest phylogenetic relatives. | |
Phenotypic microarray based carbon utilization pattern
After identifying the isolates using molecular tools, the phenotypic microarray studies were also undertaken in conjunction with metabolic profiling of isolates. The link between genetic and phenotypic functional diversity in cold environment is still not explored. Here, for the first time, the carbon substrate utilization patterns of identified cold tolerant isolates were analyzed using BIOLOG PM Plates at 4 °C. There are two operational modes to characterize the carbon utilization patterns which include increased growth by assimilation of carbon sources (classical method) or oxidation of substrates via an electron transport chain (BIOLOG method). The phenotypic microarray (PM) based method was evaluated to discriminate the carbon substrate (n = 190) utilization patterns of all the seven isolates at 4 °C. The results revealed the broad variability in metabolic potential even among the phylogenetically close isolates. Among all the seven isolates, 20.53% similarity in utilized (20/190) and non utilized (19/190) carbon sources by all the isolates (see ESI Fig. 2†) was observed. Cellobiose utilization by all isolates confirmed the presence of β-glucosidase enzyme. The carbon utilization pattern portrayed that isolates prefer simple sugars like xylose, arabinose, ribose and some other carbon sources like cellobiose and glucosamine (see ESI Fig. 2†). The isolates preferred to utilize these carbon sources which were similar to earlier reports from microbial community analyses of the Arctic region.20 H. watticus BG1 was able to metabolize 60% of the substrates, while B. altitudinis BG11 used minimum number of substrates (73/190). The yeast H. watticus BG1 showed phylogenetically distinct linkages with other bacterial isolates (Fig. 1), and its phenotypic analysis revealed its divergence based on its capacity to utilize most (114/190) and assorted carbon sources as compared with other bacterial isolates (Fig. 2). The UPGMA dendrogram revealed that three closely related isolates P. vagans BG5, P. lutea BG8 and P. graminis BG6 were also clustered closely on the basis of carbon utilization pattern (Fig. 2). Surprisingly, S. rhizophila BG9 which also belongs to the γ-Proteobacteria taxonomic group showed a highly diverse metabolic profile in both number and type of carbon substrates utilized. This incongruity between phylogenetic and phenotypic diversity was observed among bacterial isolates throughout UPGMA dendrogram. This metabolic diversity can be explained by the transcriptional regulation or genetic differences of each strain towards their specific metabolic requirements.21 Additionally, PM plates also contain 17 primary amino acids and their usage as sole carbon source was analysed. L-Isoleucine and L-leucine was utilized by all the isolates whereas L-methionine was not used by any isolate. Among the 17 amino acids, H. watticus BG1 utilized maximum (n = 13) while B. altitudinis BG11 was able to use only 4 amino acids. Alternatively, 20.53% similarity in utilized and non utilized carbon sources by all the isolates suggested substrate based micro environment adaptation in the selected isolates. These results also provide evidence that some metabolic pathways may be conserved among the strains belonging to similar environmental niche.22 This experiment represented high metabolic potential and ecological adaptation of these fascinating microbes in cold environments.
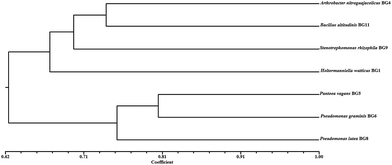 |
| Fig. 2 Metabolic diversity of selected isolates on the basis of 190 carbon substrate utility. The simple matrix (SSM) coefficient and UPGMA clustering were used to prepare the dendrogram. | |
Evaluation of selected isolates for β-glucosidase enzyme production using saccharides as carbon source
Incubation temperature is very important factor for enzyme production by any microbes. Therefore, β-glucosidase production was determined at 5 °C to 30 °C. The selected microbes were further explored for the quantitative estimation of β-glucosidase induced by different saccharides. All isolates were able to produce β-glucosidase enzyme at 5 and 30 °C, but higher enzyme production was observed at 30 °C. This might be due to the involvement of compensatory cellular biochemical mechanisms such as reduced affinity of enzymes for their substrates, decreased thermal energy and reaction rate at low temperature.23 Therefore, enzyme production was carried out at an incubation temperature 30 °C for further experimentation (Fig. 3). No microbial growth was observed at 40 °C. Among all saccharides, cellobiose was found to induce maximum β-glucosidase activity in all isolates. P. lutea BG8 showed highest β-glucosidase production as compared to other isolates and the activity ranged between 0.26 to 0.55 IU g−1 for all four selected saccharides. The result helped us to select P. lutea BG8 as the best isolate for β-glucosidase enzyme production among the seven isolates using cellobiose as a sole carbon source. As per literature, only few studies have been reported for β-glucosidase enzyme production from genus Pseudomonas24,25 and induction of β-glucosidase enzyme by cellobiose as a carbon source.26 On the basis of this experiment, P. lutea BG8 was selected for further studies.
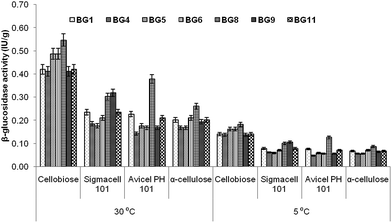 |
| Fig. 3 Effect of different carbon sources on the production of β-glucosidase by selected isolates at different temperature. | |
Effect of temperature, pH and glucose on β-glucosidase activity
Extracellular β-glucosidase activity was checked for thermal stability at temperature range of 10–60 °C (Fig. 4a). The enzyme remained active in a wide range of temperatures with highest activity at 40 °C. Any further increase in temperature resulted in rapid loss of activity. However, β-glucosidase enzyme retained 93.13%, 59.40% and 54.73% of their activity at 30, 20 and 10 °C, respectively. The temperature optimum (40 °C) is comparatively low as compared with reported temperature of most of the β-glucosidase produced by mesophilic microorganisms.27 This activity profile revealed that the β-glucosidase from P. lutea BG8 was a cold active enzyme since the optimal temperature for this enzyme is 10 °C below the optimal temperature of commercial enzymes. This might result into tremendous energy saving during saccharification. The β-glucosidase enzyme also retained 54.73% of its activity at 10 °C confirming that this enzyme is active in a broad range of temperatures. Furthermore, the stability experiment suggested that β-glucosidase enzyme retained 49.96% activity after 16 h of incubation at 30 °C (Fig. 5a). Such an enzyme with low temperature optima and high stability can provide an additional advantage during SSF with ethanologenic yeast.28
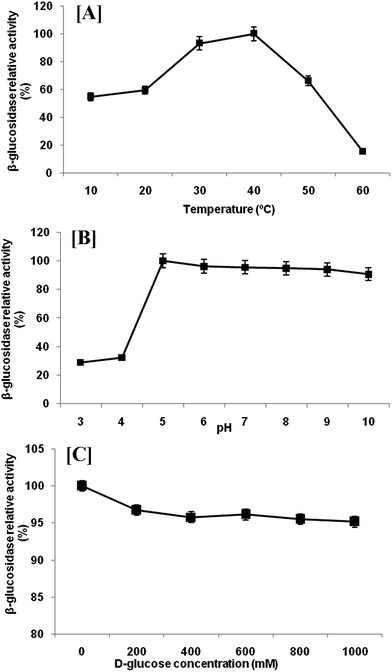 |
| Fig. 4 Effect of temperature [A], pH [B] and glucose concentration [C] on the β-glucosidase enzyme activity from P. lutea BG8. | |
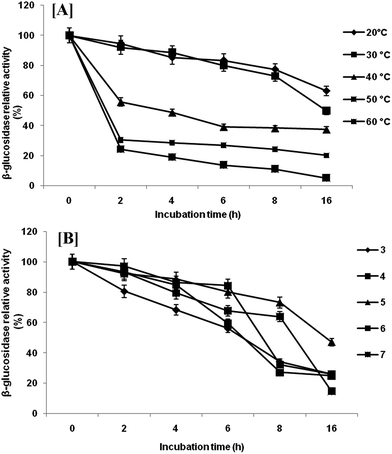 |
| Fig. 5 Temperature [A] and pH [B] stability of β-glucosidase activity from P. lutea BG8. | |
Highest β-glucosidase activity was observed at 5.0 pH, but the enzyme was active upto pH 10.0, retaining 90.61% of the activity (Fig. 4b). The enzyme was active over a broad range of pH from 5.0 to 10.0 which illustrated the alkali-tolerant nature of β-glucosidase enzyme. Alkaliphilic enzymes are widely used in various industrial applications such as alkaline pulping and detergent additives.29 Similar results have been reported by other researchers where cold active β-glucosidase from psychrotolerant or psychrophilic bacteria was active under comparable range of pH.30,31 The pH stability was also checked at 30 °C and β-glucosidase enzyme retained 49.96% activity at pH 5 after 16 h of incubation (Fig. 5b).
The inhibition of β-glucosidase by glucose is frequently a rate limiting factor during enzymatic hydrolysis of cellulose. Therefore, the inhibitory effect of glucose on β-glucosidase activity from P. lutea BG8 was also investigated. For this experimentation, different amount of glucose (0–1000 mM) was added to observe the relative activity of β-glucosidase enzyme (Fig. 4c). The result showed a very slight decrease in enzyme activity with increasing glucose concentration. The β-glucosidase retained 95.15% of enzyme activity even at 1000 mM of glucose concentration.
Kinetic parameters of β-glucosidase enzyme from P. lutea BG8
The Km and kcat of the β-glucosidase enzyme was found to be 0.636 ± 0.103 mM and 102.7 ± 0.004 s−1, respectively. The catalytic efficiency (kcat/Km) was comparable with previous reports.32,33
Efficiency of cellobiose hydrolysis by cold active β-glucosidase enzyme from P. lutea BG8
To execute these noteworthy properties of β-glucosidase enzyme from P. lutea BG8, cellobiose hydrolysis was examined at 30 °C. Cellobiose digestion rate revealed the significant impact of crude β-glucosidase enzyme on its hydrolysis resulting in the release of glucose, within 2 h of incubation (Fig. 6). The cellobiose (2%) was saccharified completely even at 30 °C within 16 h of incubation. This result also confirms the absence of feedback inhibition by glucose on β-glucosidase enzyme and very low inhibition (4.85%) was observed at high glucose concentration (1000 mM). The result confirms the unique nature of β-glucosidase enzyme since cellobiose was completely hydrolysed at low temperature without being affected by the feedback inhibition due to glucose accumulation during the hydrolysis process as reported earlier.34
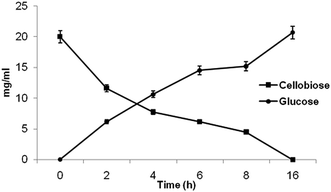 |
| Fig. 6 The time course assay for cellobiose hydrolysis and glucose release by cold active β-glucosidase from P. lutea BG8. | |
Application of cold active β-glucosidase enzyme for simultaneous saccharification and fermentation (SSF)
Further, an experiment was conducted using SSF approach with cellobiose as substrate along with crude β-glucosidase enzyme from P. lutea BG8 and ethanologenic yeast S. cerevisiae LN1. Alternatively a fermentation experiment with only S. cerevisiae LN1 along with 2% cellobiose as a sole carbon source without β-glucosidase supplementation, revealed negligible conversion of cellobiose to glucose or ethanol even after 16 h of incubation confirming the absence of β-glucosidase activity in yeast strain. In another set of experiment, cellobiose (2%) supplemented with β-glucosidase was co-inoculated with S. cerevisiae LN1 to produce ethanol (Fig. 7). After 2 h of incubation, 17.15 mg ml−1 of glucose was liberated, much higher than the amount of glucose liberated in previous experiment (6.20 mg ml−1). In SSF experiment, MGYP medium was used to prepare the primary inoculum which was used @10%v/v during fermentation. The MGYP is a complex medium containing peptone, yeast extract and malt extract which contains different metal cofactors that could have enhanced the enzyme activity of β-glucosidase in SSF experiment.
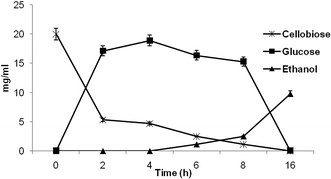 |
| Fig. 7 Performance of cold active β-glucosidase from P. lutea BG8 during simultaneous saccharification and fermentation (SSF) of cellobiose for bioethanol production by S. cerevisiae LN1. | |
After 4 h of incubation, 18.91 mg ml−1 of glucose was generated by cellobiose hydrolysis, but no ethanol production was observed during initial hours of fermentation indicating a log period in the beginning of fermentation experiment. The ethanol production was initiated only after 6 h of incubation and simultaneously the glucose was also metabolized with reduction in glucose concentration. After 16 h of incubation, 9.81 mg ml−1 of ethanol was produced with 91.42% (0.49 g ethanol per g cellobiose) of ethanol yield which proves the suitability of the enzyme for SSF process. Similarly, the HPLC chromatogram also indicated the absence of cellobiose and glucose after 16 h of incubation revealing the complete metabolism of cellobiose along with its conversion to ethanol. This experiment revealed high cellobiose conversion efficiency of cold active β-glucosidase enzyme from P. lutea BG8 during SSF process. Earlier most researchers have focused their attention on development of genetically engineered microbial strains to accomplish the efficient SSF process (Table 1).35 But the stability and efficiency of these engineered strains for industrial scale production is still questionable.36 The supplementation of this β-glucosidase with other glycosyl hydrolases may improve the SSF process of lignocellulosic biomass for bioethanol production.7,37 Hence, indigenous systems such as supplementation of cold active β-glucosidase enzyme from P. lutea BG8 during SSF process may help to address and overcome many of these challenges.
Table 1 Comparison of cellobiose fermentation efficiency of genetically engineered microbes or by supplementation of β-glucosidase from exogenous source
Ethanologenic organism |
β-Glucosidase enzyme |
Incubation temperature (°C) |
Cellobiose concentration (mg ml−1) |
Conversion rate (g ethanol per g cellobiose) |
Reference |
Genetic engineered S. cerevisiae Pc ST/Tt BG |
Heterologous expression from Thielavia terrestris (Tt BG) |
30 |
40 |
0.37 |
37 |
Genetic engineered S. cerevisiae |
Heterologous expression of cellodextrin transporters and β-glucosidase from Neurospora crassa DCDT-1G |
30 |
40 |
0.42 |
38 |
Genetic engineered S. cerevisiae |
Heterologous expression from Saccharomycopsis fibuligera |
30 |
70 |
0.54 |
10 |
Metabolic engineering of Geobacillus thermoglucosidasius |
Metabolic engineering by mutation |
60 |
30 |
0.47 |
39 |
SSF with Kluyveromyces marxianus KY3 |
β-glucosidase enzyme from Neocallimastix patriciarum |
40 |
20 |
∼0.3 |
40 |
SSF with S. cerevisiae LN1 |
β-glucosidase from P. lutea BG8 |
30 |
20 |
0.49 |
Present study |
Conclusions
In conclusion, the phylogenetic and phenotypic divergence indicated the preponderance of bacterial communities in such extreme conditions. Enzyme activity based qualitative and quantitative screening led to the selection of P. lutea BG8 for novel cold active β-glucosidase enzyme production. The cellobiose hydrolysis and ethanol production during SSF process proved that this enzyme could be used for the SSF process along with other cold active enzymes. In future, structural elucidation, catalytic mechanism of this novel enzyme needs to be deciphered to expand the knowledge about biocatalysis in extreme conditions.
Acknowledgements
Authors are thankful to National Fund for Basic, Strategic and Frontier Application Research in Agriculture (ICAR-NFBSFARA) for funding the research activities for biofuel project (NFBSFARA/AE-2006/2010-11).
References
- M. Jarvis, Nature, 2003, 426, 611 CrossRef CAS PubMed.
- R. A. Berner, Nature, 2003, 426, 323 CrossRef CAS PubMed.
- J. R. Ketudat Cairns and A. Esen, Cell. Mol. Life Sci., 2010, 67, 3389 CrossRef CAS PubMed.
- R. Margesin and V. Miteva, Res. Microbiol., 2011, 162(3), 346 CrossRef PubMed.
- R. Margesin and G. Feller, Environ. Technol., 2010, 31, 835 CrossRef CAS PubMed.
- S. Shipkowski and J. E. Brenchley, Appl. Environ. Microbiol., 2005, 71, 4225 CrossRef CAS PubMed.
- A. Wierzbicka-Woś, P. Bartasun, H. Cieśliński and J. Kur, BMC Biotechnol., 2013, 13, 22 CrossRef PubMed.
- H. X. Fan, L. L. Miao, Y. Liu, H. C. Liu and Z. P. Liu, Enzyme Microb. Technol., 2011, 49(1), 94 CrossRef CAS PubMed.
- R. R. Singhania, A. K. Patel, R. K. Sukumaran, C. Larroche and A. Pandey, Bioresour. Technol., 2013, 127, 500 CrossRef CAS PubMed.
- L. Gurgu, Á. Lafraya, J. Polaina and J. Marín-Navarro, Bioresour. Technol., 2011, 102, 5229 CrossRef CAS PubMed.
- T. M. Wood and K. M. Bhat, Methods Enzymol., 1988, 160, 87 CAS.
- M. M. Bradford, Anal. Biochem., 1976, 72, 248 CrossRef CAS.
- C. Mattéotti, P. Thonart, F. Francis, E. Haubruge, J. Destain, C. Brasseur, J. Bauwens, E. De Pauw, D. Portetelle and M. Vandenbol, Microbiol. Res., 2011, 166, 629 CrossRef PubMed.
- U. Edwards, T. Rogall, H. Blöcker, M. Emde and E. C. Böttger, Nucleic Acids Res., 1989, 17, 7843 CrossRef CAS PubMed.
- B. R. Bochner, P. Gadzinski and E. Panomitros, Genome Res., 2001, 11, 1246 CrossRef CAS PubMed.
- L. R. Nain and R. S. Rana, J. Microb. Biotechnol., 1987, 2(2), 77 CAS.
- N. Dowe and J. McMillan, Laboratory Analytical Procedure (LAP): SSF Experimental Protocols—Lignocellulosic Biomass Hydrolysis and Fermentation, National Renewable Energy Laboratory, Golden, CO, USA, 2001 Search PubMed.
- P. V. Vardhan Reddy, S. S. Shiva Nageswara Rao, M. S. Pratibha, B. Sailaja, B. Kavya, R. R. Manorama, S. M. Singh, T. N. Radha Srinivas and S. Shivaji, Res. Microbiol., 2009, 160, 538 CrossRef PubMed.
- I. Vaca, C. Faúndez, F. Maza, B. Paillavil, V. Hernández, F. Acosta, G. Levicán, C. Martínez and R. Chávez, World J. Microbiol. Biotechnol., 2013, 29, 183 CrossRef CAS PubMed.
- P. Singh, S. M. Singh and P. Dhakephalkar, Extremophiles, 2014, 18, 229 CrossRef CAS PubMed.
- S. Sabet, L. Diallo, L. Hays, W. Jung and J. G. Dillon, Extremophiles, 2009, 13, 643 CrossRef PubMed.
- E. G. Biondi, E. Tatti, D. Comparini, E. Giuntini, S. Mocali, L. Giovannetti, M. Bazzicalupo, A. Mengoni and C. Viti, Appl. Environ. Microbiol., 2009, 75, 5396 CrossRef CAS PubMed.
- P. Amato and B. C. Christner, Appl. Environ. Microbiol., 2009, 75, 718 CrossRef PubMed.
- C. L. Cheng and J. S. Chang, Bioresour. Technol., 2011, 102, 8628 CrossRef CAS PubMed.
- G. P. Hazlewood and H. J. Gilbert, Prog. Nucleic Acid Res. Mol. Biol., 1998, 61, 211 CAS.
- M. I. Rajoka, S. Khan, F. Latif and R. Shahid, Appl. Biochem. Biotechnol., 2004, 117, 75 CrossRef CAS.
- Y. H. Percival Zhang, M. E. Himmel and J. R. Mielenz, Biotechnol. Adv., 2006, 24, 452 CrossRef CAS PubMed.
- Y. Sun and J. Cheng, Bioresour. Technol., 2002, 83, 1 CrossRef CAS.
- K. Horikoshi, Microbiol. Mol. Biol. Rev., 1999, 63, 735 CAS.
- G. Zhang, X. Ma, F. Niu, M. Dong, H. Feng, L. An and G. Cheng, Extremophiles, 2007, 11, 415 CrossRef CAS PubMed.
- E. A. Zvereva, T. V. Fedorova, V. V. Kevbrin, T. N. Zhilina and M. L. Rabinovich, Extremophiles, 2006, 10, 53 CrossRef CAS PubMed.
- T. Uchiyama, K. Miyazaki and K. Yaoi, J. Biol. Chem., 2013, 288, 18325 CrossRef CAS PubMed.
- C. Riou, J. M. Salmon, M. J. Vallier, Z. Günata and P. Barre, Appl. Environ. Microbiol., 1998, 64, 3607 CAS.
- G. Stephanopoulos, Science, 2007, 315, 801 CrossRef CAS PubMed.
- J. L. Fortman, S. Chhabra, A. Mukhopadhyay, H. Chou, T. S. Lee, E. Steen and J. D. Keasling, Trends Biotechnol., 2008, 26, 375 CrossRef CAS PubMed.
- T. Arimori, A. Ito, M. Nakazawa, M. Ueda and T. Tamada, J. Synchrotron Radiat., 2013, 20, 884 CrossRef CAS PubMed.
- Y. H. Bae, K. H. Kang, Y. S. Jin and J. H. Seo, J. Biotechnol., 2014, 169, 34 CrossRef CAS PubMed.
- H. Kim, W. H. Lee, J. M. Galazka, J. H. Cate and Y. S. Jin, Appl. Microbiol. Biotechnol., 2014, 98, 1087 CrossRef CAS PubMed.
- R. E. Cripps, K. Eley, D. J. Leak, B. Rudd, M. Taylor, M. Todd, S. Boakes, S. Martin and T. Atkinson, Metab. Eng., 2009, 11, 398 CrossRef CAS PubMed.
- H. L. Chen, Y. C. Chen, M. Y. Lu, J. J. Chang, H. T. Wang, H. M. Ke, T. Y. Wang, S. K. Ruan, T. Y. Wang, K. Y. Hung, H. Y. Cho, W. T. Lin, M. C. Shih and W. H. Li, Biotechnol. Biofuels, 2012, 5, 24 CrossRef CAS PubMed.
Footnote |
† Electronic supplementary information (ESI) available: For qualitative estimation of β-glucosidase and phenotypic microarray. See DOI: 10.1039/c4ra09784j |
|
This journal is © The Royal Society of Chemistry 2014 |
Click here to see how this site uses Cookies. View our privacy policy here.