DOI:
10.1039/C4RA09673H
(Paper)
RSC Adv., 2014,
4, 64336-64346
Synthesis and characterization of amino acid-modified adsorption resins and their adsorption properties in the purification of tabersonine from Voacanga africana seeds
Received
3rd September 2014
, Accepted 10th November 2014
First published on 11th November 2014
Abstract
A series of macroporous adsorption resins anchoring valine and glycine were synthesized and characterized. The structural characterization indicated that the Brunauer–Emmett–Teller (BET) surface area and pore volume of the resins decreased after functionalization with amino acids. The adsorption kinetics and adsorption isotherm experiments indicated that the pseudo second-order rate equation was more appropriate for characterizing the kinetic data and the Freundlich model was more suitable for fitting the equilibrium data. Moreover, the results also revealed that the maximum adsorption capacity of tabersonine on resins reduced to 14 mg g−1 from 254 mg g−1 after functionalization with valine. Causes for the low adsorption capacity of tabersonine on the modified resins were discussed. The modified resins were applied to purify tabersonine extracted from Voacanga africana seeds. The results showed that the modified resins were good for the adsorption of impurities but poor for the adsorption of tabersonine from the extracts of Voacanga africana seeds. Tabersonine was efficiently purified with purity above 90%. The reusability of the modified resins was also assessed and the modified resins exhibited considerable reusability.
Introduction
Voacanga africana is an evergreen tree or some would also argue a shrub, which reaches a height of up to 6 meters with widely spreading low crowns around it. It is mainly distributed in rainforests in the West Africa. Generally, its fruits are found in pairs, spherical shape, and contain several brown seeds that are irregular in shape.1 In addition, its bark and root are widely used in traditional medicines. Even though, its seeds are actually the most economical part for application in medicine industry. The seeds are considered as an important source of annual additional income for harvesters and exporters in West Africa.2 In order to efficiently utilize these seeds, Koroch et al. have reviewed its chemistry and pharmacological activities.2 However, there are still very few reports about these seeds. Therefore, more researches are worthy to be carried out for this important African medical plant.
In the past decades, several chemical components have been isolated from different organs of Voacanga species.3–5 Among them, tabersonine, a well known alkaloid, was isolated from Voacanga seeds.1,6 Its structure and properties have been disclosed in the prior art treatise.7 It is known to have an indole-like structure and is related to the Vinca alkaloids. Its content in Voacanga seeds is about 2.0%.8 The chemical structure of tabersonine is shown in Fig. 1. Many researchers have reported that tabersonine plays a significant role in lowering blood pressure and it can be used to semi-synthesize the cerebrally active eburnamine derivatives such as vincamine and the anti-cancer drug vinpocetine.9–11 In 1973, Poisson published a patent related to acquisition of tabersonine by repeated extraction and crystallization.8 However, this method has many disadvantages, such as the use of toxic extraction solvents and the low yield of tabersonine. In 2008, Zhang et al. provided another method to purify tabersonine. Tabersonine in the extracts of Voacanga seeds was purified by commercial macroporous adsorption resins (MARs).12 This is a promising method to obtain tabersonine due to its simple operation and considerable yield. However, this method also has some shortcomings that need to be improved. 10 kg of commercial MARs (D101) were required to purify tabersonine from 1 kg Voacanga seeds.13 The dosage of the commercial MARs is the key factor that would increase the cost of industrial production. This problem is mainly due to the fact that the commercial MARs (D101) lacks adsorption selectivity toward tabersonine, which leads to impurities in the extracts of Voacanga seeds. Thus, more MARs are required to increase the number of theoretical plate for separation.
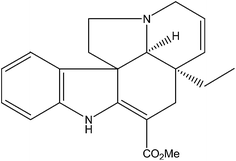 |
| Fig. 1 Chemical structure of tabersonine. | |
Many authors have reported that the commercial MARs exhibit unique adsorption properties and have advantages in purifying natural products.14–16 However, the mechanisms of adsorption of commercial MARs are mainly based on hydrophobic forces, such as the van der Waals force, which may result in low adsorption capacity and selectivity.17 In recent years, many researchers have showed significant interest in modifying MARs with functional groups to obtain new adsorbents with high adsorption capacity and selectivity.18–21 These researchers showed that the MARs modified with special functional groups, such as chloromethyl, amino, and phenylamino, can be potentially applied in forming special MARs for the purification of the target compound. It can be easily found that almost all these studies concentrate on the improvement of the adsorption capacity of MARs toward the target compound. However, to date, studies on modifying MARs, which are committed to decrease the adsorption capacity of the MARs toward the target compound and to maintain or increase the adsorption capacity of MARs toward the impurities, have rarely been carried out. In addition, there are no studies that have been carried out to functionalize MARs to purify tabersonine from Voacanga seeds.
Considering the importance of this African medical plant and the low efficiency of purification of tabersonine by commercial MARs, the aim of this work was to improve the efficiency of purification of tabersonine. In the present paper, a series of modified MARs were synthesized by introducing amino acids. The modified MARs exhibited a good ability to adsorb impurities but a poor ability to adsorb tabersonine from the extracts of Voacanga africana seeds. Tabersonine could be efficiently purified from the Voacanga seeds by modified MARs. The corresponding adsorption behaviors of the modified MARs toward tabersonine were systemically investigated and the reusability of the modified resins was also assessed.
Experimental
Chemical reagents, adsorbents, and samples
D101, nonpolar styrene co-divinylbenzene copolymer with no functional groups, was purchased from Chemical Factory of Nankai University (Tianjin, China). All the MARs were classified by sieves. The fraction of sizes between 0.45 and 0.60 mm were used in the experiments. Before the experiments, the resins were pretreated by soaking overnight in ethanol, and subsequent thorough washing with distilled water. Chloromethyl methyl ether was purchased from Henan wanxiang Technology & Trade Co., Ltd. (Henan, China). The reagents and chemicals (analytic grade unless stated otherwise) were purchased from Sinopharm Chemical Reagent Co., Ltd. and Tianjin Damao Chemical Reagent Co., Ltd (China). Tabersonine (purity > 98%) was purchased from Enjoye C & G Bioengineering Co., Ltd (Xiamen, China).
Synthesis of adsorbents with functional groups
The reaction scheme for the preparation of MARs with chloromethyl groups (PS-Cl) and MARs with amino acid (PS-amino acid) on the basis of D101 is shown in Fig. 2. Pretreated D101 copolymer beads (15 g) were allowed to swell in dichloromethane (100 mL) for 24 h. Then, zinc chloride (15 g), sodium chloride (20 g) and chloromethyl methyl ether (70 mL) were mixed with the beads soaked in a 500 mL three-necked round bottomed flask equipped with a mechanical stirrer, a reflux condenser, and a thermometer. The reaction mixture was stirred (100 rpm) at 323 K for 20 h. The synthetic MARs were filtered and washed with distilled water and methanol until no white precipitate formed while an aqueous solution of silver nitrate was added to the filtrate.19 The MARs with chloromethyl groups (PS-Cl) were obtained. The chloromethylated beads PS-Cl (6 g) were allowed to swell in 15 mL methanol for 24 h. Then, an aqueous solution of amino acid (0.05 mol) in 125 mL distilled water and pyridine (0.05 mol) was added. The mixture was stirred (100 rpm) and refluxed for 20 h. The amino acid linked polymer beads (PS-amino acid) were filtered, and then washed with water and methanol and dried under vacuum.22
 |
| Fig. 2 Reaction scheme for the preparation of PS-Cl and PS-amino acid on the basis of D101. | |
Characterization of MARs
Physical properties of the MARs were determined by N2 adsorption/desorption isotherms at 77 K using a ASAP 2020 automatic surface area and porosity analyzer. The BET surface area was obtained by the BET method. The pore volume and average pore diameter were obtained by the Barrett–Joyner–Halenda (BJH) method. The surface morphology of the MARs was observed by Field-emission scanning electron microscopy (FEI Sirion 200). FTIR spectra of MARs were obtained on a FTIR spectrophotometer in the 4000–400 cm−1 region using the KBr pellet method. The content of chloromethyl in the MARs was determined by an electrochemistry method, which was described by Song Lou et al.20 A known weight of the dry MARs (0.2000 ± 0.0020 g) was heat-dissolved in a nickel crucible at 873 K for 8 h with 0.5 g of melting sodium hydroxide. The melted masses were diluted in distilled water to 250 mL, and then the electric potential of the sample aqueous solution was determined using an electrochemical workstation (RST 5200, Zhengzhou, China). The content of the chloromethyl group was calculated from the following equation with a range of 0.01–100 mmol g−1 and R2 = 0.9997. |
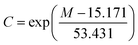 | (1) |
|
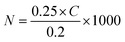 | (2) |
where C (mol L−1) refers to the concentrations of the sample aqueous solution, M (mV) is the electric potential of the sample aqueous solution, N is the content of chloromethyl group (mmol g−1 dry adsorbent). The content of amino acid in MARs was calculated according to the reduction of the content of the chloromethyl after the substitution of Cl with the amino acid moiety. The content of amino acid in MARs could also be obtained by determining the available content of free –COOH group in PS-amino acid, which could be confirmed by the method described by Valodkar et al.22 A known weight of the dry MARs (0.5000 ± 0.0020 g) in 30 mL distilled water was refluxed for 12 h in the presence of excess NaOH (10 mL, 0.1 N) containing a few drops of phenolphthalein indicator. Upon cooling, the solution was filtered and washed carefully with 2 × 5 mL portions of water, and then the filtrate was titrated with 0.1 N HCl. The content of amino acid in the PS-amino acid could be calculated from the titer value. The contents for all the functional groups were obtained by considering the mean value of triplicate assays.
Preparation of the extracts of Voacanga seeds
The extract of Voacanga seeds was prepared as follows: the dried powder of the seeds (100 g) was extracted with 90% methanol (v/v, 200 mL × 5), refluxing for 1.5 h. The extract was filtered and concentrated to remove the methanol under reduced pressure. The residue was dissolved in 250 mL 1.0% HCl solution (w/v). After filtering, an extract was obtained with 7.6 mg mL−1 tabersonine.
Preparation of the sample solution of tabersonine
In this work, the sample solutions of tabersonine were prepared as follows: tabersonine (purity > 98%) with a weight of 0.4000 ± 0.0010 g, 0.3000 ± 0.0010 g, 0.2000 ± 0.0010 g, 0.1000 ± 0.0010 g, 0.0500 ± 0.0010 g, and 0.0200 ± 0.0010 g were dissolved in 50 mL 1.0% HCl (w/v), and then diluted with 1.0% HCl (w/v) to 100 mL. The concentrations of tabersonine in the obtained sample solutions were 0.2, 0.5, 1.0, 2.0, 3.0 and 4.0 mg mL−1, respectively. In the experiments, tabersonine was dissolved in acidic solution. The ester group in the structure of tabersonine may be hydrolyzed to a carboxylic acid. Thus, the stability of the sample solutions was investigated. The changes in the contents of tabersonine in the sample solutions were determined by HPLC. The results showed that the sample solutions were stable up to 8 h at room temperature.
Calculation of adsorption capacity
The adsorption capacities of the MARs toward tabersonine were calculated according to the following equation: |
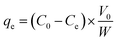 | (3) |
where qe is the adsorption capacity (mg g−1 dry resin) toward tabersonine at adsorption equilibrium. C0 and Ce are the initial and equilibrium concentrations of tabersonine solutions (mg mL−1). V0 is the volume of the tabersonine solutions. W is the weight of dry MARs used (g).
In our experiments, the coefficient of recovery (P) and the relative standard deviation (RSD) were employed to evaluate the accuracy and precision of our experimental methods. The values of P and RSD were 98.8% and 0.38%, respectively, which indicated that the accuracy and precision could satisfy the needs of our experiments.
Adsorption kinetics
In this paper, we attempted to decrease the adsorption capacity of the modified MARs toward tabersonine and to maintain or increase the adsorption capacity of the modified MARs toward the impurities in Voacanga seeds. Considering that the carboxyl groups in modified MARs cannot react with protonated tabersonine through acid–base interaction, all the adsorption experiments were carried out in acidic solutions (HCl solution).
The evaluation of kinetics is a benefit for the prediction of adsorption time and sample concentration. In this study, the adsorption kinetics curves of tabersonine on the MARs were measured in the following condition: 25 mL of tabersonine solution (C0 = 1.0 mg mL−1) was shaken with pretreated MARs (0.2000 ± 0.0020 g dry resin) in a 100 mL stoppered conical flask in a SHA-B incubator (100 rpm) at 298, 308 and 318 K. Subsequently, the concentration of tabersonine in the adsorption solution was determined at different times until equilibrium.
Adsorption isotherm
The equilibrium adsorption isotherms of tabersonine on the MARs were carried out as follows: solutions (25 mL) with different concentrations of tabersonine (C0 = 0.2, 0.5, 1.0, 2.0, 3.0 and 4.0 mg mL−1) were contacted with pretreated MARs (0.2000 ± 0.0020 g of dry resin) in conical flasks. The flasks were continually shaken for 6 h (100 rpm) at temperatures of 298, 308 and 318 K. Then, the concentrations of tabersonine in the adsorption solutions were determined.
Dynamic adsorption and desorption tests (purification of tabersonine from Voacanga seeds)
Dynamic adsorption and desorption tests were carried out in glass columns (15 mm × 200 mm) wet-packed with about 10 mL of the selected adsorbents. The weight of MARs was measured to be about 2.9 g. The extracts of Voacanga seeds passed through the column at a flow rate of 3 BV h−1 (1 BV = 10 mL). The effluent liquid of the extracts, which passed through the column, was collected. The purity of tabersonine in the effluent was determined by HPLC. The adsorption test was terminated when the purity of tabersonine reached the initial purity (37.6%). Then, the columns were washed with de-ionized water (2.5 BV), and then washed with 80% ethanol solution (5 BV). The composition of desorption solution was also determined by HPLC.
HPLC analysis
HPLC analysis was employed to determine the purities of tabersonine purified by different MARs. The samples were analyzed using a LC-2010A instrument (Shimadzu, Japan). A UV detector operated at 254 nm was applied. The column was a LiChrospher C18 column (4.6 mm × 250 mm, 5 μm). The temperature was 35 °C. The mobile phase was a 65
:
35 (v/v) mixture of acetonitrile and ultrapure water. The flow rate was set at 1.0 mL min−1. With the content of the standard substance (tabersonine) between 0.0088 mg mL−1 and 0.0440 mg mL−1, the peak area had a good linear relationship with the concentration and the equation of linear regression was “Y = 1.9313E + 8X + 148
969, R2 = 0.9999”. On the basis of the peak areas of tabersonine in the HPLC curves, the contents of tabersonine in the samples were calculated.
Results and discussion
Characterization of the MARs
Fig. 3 shows the adsorption/desorption isotherms of D101, PS-Cl, PS-glycine and PS-valine. The results showed that the isotherms of D101 and PS-Cl were similar, while that of PS-glycine and PS-valine were similar. This result indicated that the properties of MARs have been changed with anchored amino acids. Physical properties of D101, PS-Cl, PS-glycine and PS-valine are tabulated in Table 1. The BET surface areas of MARs followed the order of PS-Cl > D101 > PS-glycine > PS-valine. This order revealed that the BET surface area of MARs decreased after functionalization with amino acids. The average pore diameter of MARs had the following order: PS-glycine > PS-valine > PS-Cl > D101. It revealed that the average pore diameter of MARs increased after functionalization with amino acids. The pore volume of MARs had the following order: D101 > PS-Cl > PS-glycine > PS-valine. This result revealed that the pore volume of MARs decreased after functionalization with amino acids.
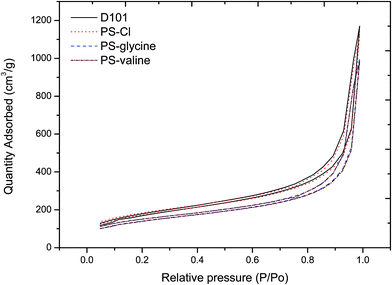 |
| Fig. 3 Nitrogen adsorption/desorption isotherms of D101, PS-Cl, PS-glycine and PS-valine. | |
Table 1 Physical Properties of D101, PS-Cl, PS-glycine and PS-valine
Resin series |
D101 |
PS-Cl |
PS-glycine |
PS-valine |
BET surface area (m2 g−1) |
607.5 |
608.1 |
499.2 |
495.6 |
Average pore diameter (nm) |
13.0 |
13.4 |
14.5 |
14.3 |
Pore volume (cm3 g−1) |
1.77 |
1.67 |
1.52 |
1.49 |
Particle size (mm) |
0.45–0.60 |
0.45–0.60 |
0.45–0.60 |
0.45–0.60 |
Fractal dimension D |
2.608 |
2.629 |
2.616 |
2.617 |
Fractal geometry is popularly applied in adsorption science. The fractal dimension D is the key quantity in fractal geometry. It can be used to measure the surface and structural irregularities of the given solid. The value of D can be determined on the basis of the Frenkel-Halsey-Hill (FHH) equation. It can be calculated as follows:19,23,24
|
ln q = const – (3 – D)ln A; A = RT ln(p0/p)
| (4) |
where
p0 and
p are the saturation and equilibrium vapor pressures (mmHg),
R is the universal gas constant (8.314 J (mol
−1 K
−1)),
T is the absolute temperature (K).
The values of D were calculated using the slope of ln q versus ln A and they are shown in Table 1. The values of D for D101, PS-Cl, PS-glycine and PS-valine were 2.608, 2.629, 2.616 and 2.617, respectively. It is reported that the lower limiting value of 2 corresponds to a perfectly regular smooth surface, whereas the upper limiting value of 3 relates to the maximum allowed complexity of the surface.25 In our experiments, the values of D had the following order: D101 < PS-glycine < PS-valine < PS-Cl. Moreover, the values of D between these MARs had small differences. The order of the values D meant that the surface of MARs became relatively irregular after functionalization. The small differences of D indicated that their degrees of regular smoothness almost remained constant. This is probably because the number of the functional groups in MARs are less and similar.26
Fig. 4 shows the SEM characterization of D101 (a) and PS-amino acid (b). Comparing image (a) with image (b), it shows that the irregular surface structure and large pores were observed on the surface of PS-amino acid (b), while a more uniform surface was observed on the surface of D101 (a). This result was consistent with the data shown in Table 1 (average pore diameter, the fractal dimension D). These differences in the surface may suggest that the amino acid groups existed in the modified MARs.
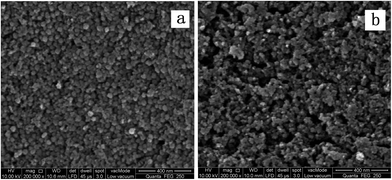 |
| Fig. 4 SEM characterization of MARs: (a) D101, (b) PS-amino acid. | |
Curves (a)–(d) in Fig. 5 are the FTIR spectra of D101, PS-Cl, PS-valine and PS-glycine, respectively. In the spectrum of PS-Cl (b), there was an absorption band in the vicinity of 678 cm−1, which was the stretching vibration band of the C–Cl bond. The absorption band at 1264 cm−1 was the in-plane bending vibrations adsorption of the
C–H bond of the benzene ring after binary substitution caused by the substitution of hydrogen atoms at the para position of benzene rings by –CH2Cl.27 In the spectrum of PS-valine (c) and PS-glycine (d), the amine group was disclosed by the adsorption peaks that appear at 3482 and 3444 cm−1, respectively. In addition, the absorption band in the vicinity of 678 cm−1 still existed in curves (c) and (d). This result indicated that the –Cl groups were not fully substituted by amino acid during the reaction. On the basis of the electrochemistry method, the content of chloromethyl in the PS-Cl was 1.74 mmol g−1 and the contents of amino acid in PS-valine and PS-glycine were 1.02 mmol g−1 and 1.09 mmol g−1, respectively. According to the method described by Valodkar et al.,22 the contents of amino acid in PS-valine and PS-glycine were 0.95 mmol g−1 and 1.03 mmol g−1, respectively, which were close to the result obtained by the electrochemistry method.
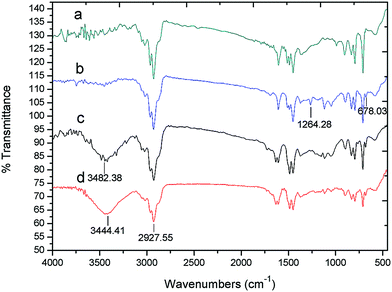 |
| Fig. 5 FTIR spectra of adsorbents D101 (a), PS-Cl (b), PS-valine (c) and PS-glycine (d). | |
Adsorption kinetics of tabersonine on MARs
Adsorption kinetics of tabersonine on MARs were studied at 298 K, 308 K, and 318 K, respectively. The adsorption kinetics curves are shown in Fig. 6. The results indicated that the adsorption capacities of tabersonine on MARs sharply increased in the first 40 min, and then became slow until equilibrium and all of the adsorptions reach equilibrium within 150 min. By comparison of curves at different temperatures, it can be concluded that higher temperature leads to lower adsorption capacity of the MARs at equilibrium. Compared with Fig. 6a, the adsorption capacities of tabersonine on PS-amino acid (Fig. 6c and d) were considerably smaller. The adsorption capacities of tabersonine on PS-amino acid were 9–16 mg g−1, which were considerably smaller compared to the adsorption capacity of tabersonine on D101 (about 110 mg g−1). The PS-amino acid exhibited a poor ability to adsorb tabersonine.
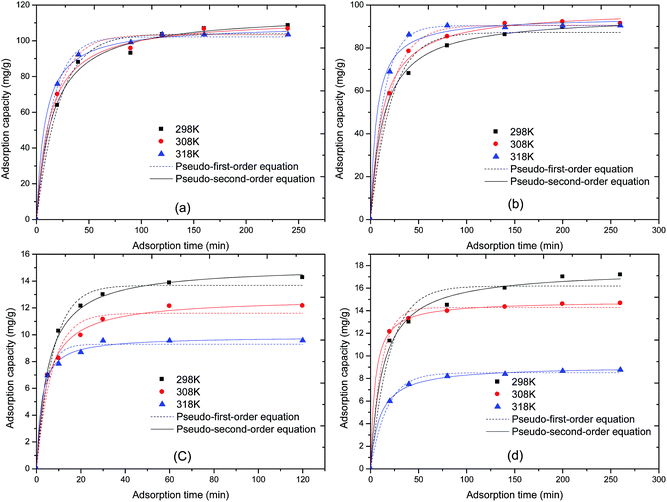 |
| Fig. 6 Adsorption kinetics curves of tabersonine on D101 (a), PS-Cl (b), PS-valine (c), and PS-glycine (d) at different temperatures. | |
In order to better illustrate the mechanisms of adsorption of tabersonine on MARs, two kinetics models (pseudo-first-order and pseudo-second-order kinetics models) were employed to fit the experimental data. The model which performed best was selected on the basis of the linear regression correlation coefficient values (R2) and the average absolute relative deviation (AARD). The kinetics equations are presented as follows:
Equation of pseudo-first-order kinetics model:
|
ln(qe − qt) = lnqe − k1t
| (5) |
Equation of pseudo-second-order kinetics model:
|
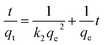 | (6) |
where
qe and
qt are the adsorption capacity of tabersonine on the MARs at equilibrium and at any time
t (mg g
−1 dry resin), respectively. The parameters
k1 (min
−1) and
k2 (g (mg
−1 min
−1) are the rate constants of the pseudo-first-order and pseudo-second-order models for the adsorption process, respectively.
In the calculations, the adjustable parameters are obtained by fitting the model against the experimental data, and the average absolute relative deviation (AARD) between the experimental adsorption capacities and calculated values was employed to evaluate the models. The equation of AARD is shown as follows:
|
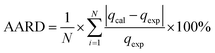 | (7) |
where
N is the number of experimental data points. The
qcal and
qexp are the calculated and experimental values, respectively.
The kinetic data in Fig. 6 were fitted by these two models. It was found that the pseudo second-order kinetic equation was more appropriate for the adsorption. The values of kinetic parameters are shown in Table 2. The calculated results of the pseudo-first-order rate equation showed that the values of R2 were almost less than 0.99 for all the MARs, showing the bad quality of linearization. In contrast, the values of R2 for the pseudo-second-order rate equation were all larger than 0.99. In addition, the values of AARD for the pseudo-second-order rate equation were almost smaller compared to the pseudo-first-order rate equation. Thus, the adsorption of tabersonine on D101, PS-Cl, PS-valine and PS-glycine better fit the pseudo-second-order model. The calculated qe decreased with increasing temperature, which is in accordance with the phenomenon discussed above.
Table 2 Values of kinetic parameters for adsorption of tabersonine on MARs
Adsorbent |
Temperature (K) |
Pseudo-first-order equation |
Pseudo-second-order equation |
k1 (min−1) |
qe (mg g−1) |
R2 |
AARD (%) |
k2 (g mg−1 min−1) |
qe (mg g−1) |
R2 |
AARD (%) |
D101 |
298 |
0.0454 |
103.8 |
0.9844 |
3.18 |
0.000536 |
116. |
0.9943 |
2.66 |
308 |
0.0556 |
103.3 |
0.9899 |
2.86 |
0.000679 |
113.6 |
0.9971 |
1.44 |
318 |
0.0652 |
102.1 |
0.9971 |
1.83 |
0.001390 |
107.5 |
0.9986 |
1.13 |
PS-Cl |
298 |
0.047 |
87.2 |
0.9797 |
5.19 |
0.00068 |
97.0 |
0.9973 |
1.65 |
308 |
0.057 |
90.5 |
0.9967 |
1.56 |
0.00105 |
96.1 |
0.9959 |
2.03 |
318 |
0.073 |
90.4 |
0.9998 |
0.425 |
0.00278 |
92.5 |
0.9924 |
2.8 |
PS-valine |
298 |
0.134 |
13.6 |
0.9919 |
3.18 |
0.0126 |
15.1 |
0.9970 |
2.18 |
308 |
0.146 |
11.6 |
0.9662 |
7.23 |
0.0166 |
12.7 |
0.9933 |
3.16 |
318 |
0.243 |
9.2 |
0.9814 |
4.75 |
0.0457 |
9.8 |
0.9955 |
2.06 |
PS-glycine |
298 |
0.0504 |
16.1 |
0.9677 |
6.36 |
0.00445 |
17.6 |
0.9928 |
3.01 |
308 |
0.0901 |
14.2 |
0.9948 |
2.13 |
0.0147 |
14.8 |
0.9998 |
0.33 |
318 |
0.0583 |
8.5 |
0.9957 |
2.23 |
0.0113 |
9.1 |
0.9986 |
1.15 |
Adsorption isotherms of tabersonine on the MARs
The equilibrium adsorption isotherms of tabersonine on MARs were carried out at 298 K, 308 K and 318 K, respectively. The results are shown in Fig. 7. It is obvious that the adsorption capacities of all the MARs increased with increasing concentration of tabersonine, while decreased with increase in temperature. Comparing the adsorption capacities between Fig. 7a, c and d, it can be concluded that the maximum adsorption capacity of tabersonine on MARs significantly decreased after modification.
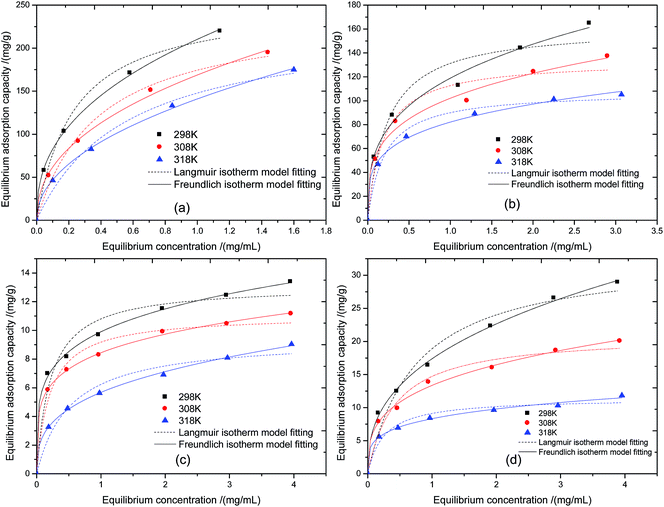 |
| Fig. 7 Adsorption isotherms of tabersonine on D101 (a), PS-Cl (b), PS-valine (c), and PS-glycine (d) at different temperatures. | |
In order to understand the mechanism of adsorption of tabersonine on MARs, two isotherm equations have been employed to explain the process of adsorption equilibrium. In the present paper, the kinetic curves were fitted with the isotherm models of Langmuir and Freundlich, and the results are shown in Table 3.
Table 3 Langmuir and Freundlich Isotherm Parameters for the Adsorption Process of Tabersonine on MARs at 298 K, 308 K and 318 K
Adsorbent |
Temperature (K) |
Langmuir |
Freundlich |
qm (mg g−1) |
KL (mL mg−1) |
R2 |
AARD (%) |
KF ((mg g−1) (mL mg−1)1/n) |
1/n |
R2 |
AARD (%) |
D101 |
298 |
254.2 |
0.23 |
0.9814 |
12.33 |
210.4 |
0.406 |
0.9991 |
2.80 |
308 |
241.2 |
0.38 |
0.9867 |
5.37 |
168.8 |
0.437 |
0.9972 |
3.58 |
318 |
231.0 |
0.56 |
0.9864 |
8.86 |
141.0 |
0.477 |
0.9989 |
3.12 |
PS-Cl |
298 |
161.8 |
0.233 |
0.9830 |
8.13 |
118.9 |
0.507 |
0.9959 |
3.53 |
308 |
133.8 |
0.188 |
0.9814 |
9.96 |
102.1 |
0.437 |
0.9934 |
4.01 |
318 |
107.9 |
0.199 |
0.9810 |
8.33 |
82.1 |
0.414 |
0.9909 |
4.35 |
PS-valine |
298 |
13.1 |
0.519 |
0.9667 |
7.31 |
9.7 |
0.224 |
0.9991 |
1.13 |
308 |
11.1 |
0.215 |
0.9782 |
5.51 |
8.3 |
0.213 |
0.9989 |
1.17 |
318 |
9.5 |
0.205 |
0.9680 |
7.85 |
5.6 |
0.334 |
0.9989 |
1.21 |
PS-glycine |
298 |
32.9 |
0.287 |
0.9735 |
8.7 |
17.4 |
0.383 |
0.9987 |
1.75 |
308 |
21.0 |
0.213 |
0.9742 |
7.83 |
13.3 |
0.303 |
0.9956 |
2.48 |
318 |
11.4 |
0.21 |
0.9663 |
7.25 |
8.3 |
0.234 |
0.9957 |
1.95 |
The Langmuir isotherm equation and Freundlich isotherm equation are represented as eqn (8) and (9), respectively:
|
Ce/qe = KL/qm + Ce/qm
| (8) |
|
lg qe = (1/n)lg Ce + lg KF
| (9) |
where
qe and
qm are the equilibrium and maximum adsorption capacity (mg g
−1 dry resin), respectively.
Ce is the equilibrium concentration of tabersonine solution (mg mL
−1).
KL is the parameter related to the adsorption energy (mL mg
−1).
KF reflects the adsorption capacity of MARs ((mg g
−1) (mL mg
−1)
1/n). The parameter
n represents the adsorption affinity of the adsorbent for MARs.
The equilibrium data in Fig. 7 were fitted by these two isotherm models. It was found that the Freundlich model was more appropriate for describing the experimental data. The corresponding parameters of Langmuir model and Freundlich model were calculated and are tabulated in Table 3. The values of R2 and AARD were accordant with the results of the data fitting in Fig. 7. The qm calculated from Langmuir model indicated that the maximum adsorption capacities of tabersonine on D101, PS-Cl, PS-valine and PS-glycine were about 254 mg g−1, 161 mg g−1, 14 mg g−1, and 34 mg g−1, respectively. The values of qm had the following order: D101 > PS-Cl > PS-glycine > PS-valine. The PS-amino acid exhibited a poor ability to adsorb tabersonine.
Adsorption thermodynamics of tabersonine on MARs
In order to obtain in-depth information on inherent energetic changes associated with the adsorption process, adsorption thermodynamics of tabersonine on MARs were investigated. KL, the Langmuir isotherm equilibrium constant, can be used to examine the thermodynamic parameters. The free energy of adsorption (ΔG0) is related to the equilibrium constant. Enthalpy (ΔH0) and entropy (ΔS0) changes are calculated based on the Van't Hoff equation. Thermodynamic parameters, such as ΔG0, ΔH0, and ΔS0, associated with the adsorption process can be estimated using the following equations:
|
ΔG0 = −RT ln K
| (10) |
ln K = −ΔH0/RT + ΔS0/R |
where M is the molecular weight of tabersonine (336.42 g mol−1) and T is the absolute temperature (K). The plot of ln
K versus 1/T showed a straight line, and the values of ΔH0 and ΔS0 were calculated from the slope and intercept, respectively.
As shown in Table 4, the values of ΔG0 were all negative, which indicated that the adsorption processes were spontaneous. The value of ΔH0 for D101 was negative, indicating that the adsorption process was exothermic. The positive values of ΔH0 for PS-Cl, PS-valine and PS-glycine indicated that the adsorption processes were endothermic in nature. The value of ΔS0 for D101 was negative, which indicated that the randomness decreased in the adsorption process. The positive values of ΔS0 for PS-Cl, PS-valine and PS-glycine indicated that randomness at the solid–liquid interface increased during the adsorption process.28 In the section Characterization of the MARs, the results showed that the surface of MARs became relatively irregular after functionalization. The surface of D101 was more regular and tabersonine molecules were arranged from the irregular state to the regular state at the solid–liquid interface during the adsorption process. Thus, the value of ΔS0 for D101 was negative. For PS-Cl, PS-valine and PS-glycine, their surfaces became relatively irregular after functionalization. Thus, the values of ΔS0 for them were positive.
Table 4 Thermodynamic parameters for the adsorption process of tabersonine on MARs at different temperatures
Adsorbent |
ΔG0 (kJ mol−1) |
ΔH0 (kJ mol−1) |
ΔS0 (J (mol−1 K−1)) |
298 K |
308 K |
318 K |
D101 |
−18.0 |
−17.4 |
−16.9 |
−34.9 |
−57 |
PS-Cl |
−18.0 |
−19.2 |
−19.7 |
6.5 |
81 |
PS-valine |
−17.1 |
−18.2 |
−18.9 |
9.6 |
97 |
PS-glycine |
−17.5 |
−18.8 |
−19.4 |
12.2 |
100 |
Reasons for the low adsorption capacity of tabersonine on the PS-amino acid
The effective interactions between adsorbate and adsorbent include van der Waals forces, hydrogen bonding, hydrophobic interaction, and electrostatic interaction.19 D101 is a nonpolar styrene-co-divinylbenzene copolymer with no functional groups. The π–π interaction between the phenyl rings of D101 and tabersonine was maybe one of the main driving forces for the adsorption. Compared with D101, PS-Cl possessed a chloromethyl group, which hardly had any effect on the adsorption of tabersonine. However, by introducing a chloromethyl group into D101, the steric resistance increased so that the adsorption capacities for tabersonine slightly decreased. When an amino acid was introduced into the MARs, the steric resistance was further increased and adsorption capacities were correspondingly decreased. In the section Characterization of the MARs, the structural characterization revealed that the BET surface area and pore volume of MARs decreased after functionalization with amino acids. Such changes in the physical properties could also result in the low adsorption capacities for PS-amino acid. More important, the modified MARs contained carboxyl groups. Tabersonine is an alkaloid and it was dissolved in HCl solution. Tabersonine was protonated and cannot react with the carboxyl group on the adsorbent matrix through acid–base interaction. Therefore, the adsorption capacity of tabersonine on modified MARs was low in the acidic pH conditions.
Dynamic adsorption and desorption tests (purification of tabersonine from Voacanga seeds)
The dynamic test is important for judging the efficiency of purification of tabersonine by PS-amino acid. Fig. 8 presents the continuous column adsorption curves of D101 and PS-amino acid columns toward tabersonine. The initial concentration of tabersonine in the extracts was 7.6 mg mL−1. In this study, the residual concentration of tabersonine in the effluent was recorded until it reached the initial concentration. The results showed that the residual concentration of tabersonine in the effluent of D101, PS-valine and PS-glycine reached the initial concentration at the volume of effluent were 8.5 BV, 0.6 BV and 0.7 BV, respectively. This result meant that the dynamic adsorption capacities of tabersonine on D101, PS-valine and PS-glycine were about 222.3 mg g−1, 15.7 mg g−1 and 18.3 mg g−1, respectively. The result confirmed that PS-amino acid has low adsorption capacity toward tabersonine.
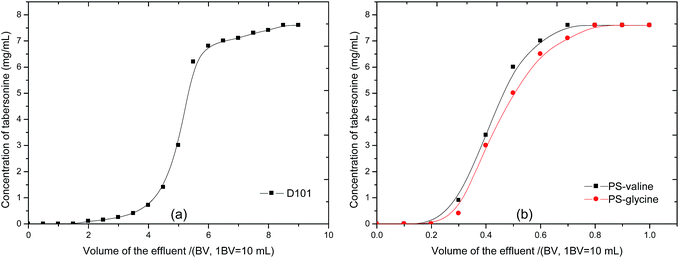 |
| Fig. 8 Residual concentration of tabersonine in the effluent of D101 (a) and PS-amino acid (b). | |
The aim of this work was to obtain high purity of tabersonine. The investigation of the purity of tabersonine in the effluent is more important to evaluate the performance of PS-amino acid. Fig. 9 shows the purities of tabersonine at different volumes of effluent. In this study, the breakthrogh point was that the purity of tabersonine was 90% and the maximum volumes of extracts that could be purified by PS-valine and PS-glycine were 2.8 BV and 2.3 BV, respectively. This result revealed that high purity of tabersonine (>90%) could be obtained in the effluent and impurities were adsorbed on PS-amino acid. In our experiments, 250 mL extract was obtained from 100 g Voacanga seeds. Thus, the required dosages of PS-valine and PS-glycine, which were used to purify tabersonine from 1 kg of Voacanga seeds, were about 268 g and 326 g, respectively. Moreover, this result also revealed that impurities from 3.73 g and 3.06 g seeds could be sufficiently adsorbed on 1.0 g PS-valine and 1.0 g PS-glycine, respectively. These data indirectly revealed the adsorption capacities of impurities on PS-valine and PS-glycine. The curve of D101 in Fig. 9 showed that the purity of tabersonine had a slight decrease after adsorption. This result indicated that the adsorption ability of tabersonine on D101 is slightly stronger than that of impurities. The commercial MARs D101 showed no apparent adsorption selectivity toward tabersonine and impurities in the extract. Thus, a large number of D101 was required to separate them.
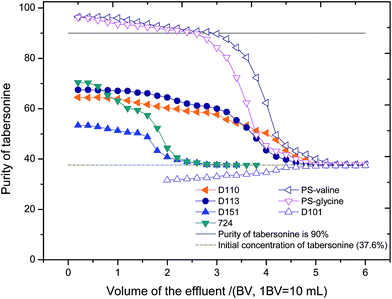 |
| Fig. 9 Purity of tabersonine in the effluent. | |
The desorption tests for removing the impurities on PS-amino acid were also investigated. The result revealed that impurities could be desorbed by washing with 5 BV 80% ethanol (v/v). This result suggested that PS-amino acid could be regenerated using 5 BV 80% ethanol.
Surface morphology of tabersonine adsorbed on PS-amino acid (tabersonine solution with 7.6 mg mL−1 concentration was passed through the PS-amino acid column), and impurities adsorbed on PS-amino acid (the extracts of Voacanga seeds passed through the PS-amino acid column, and then washed with de-ionized water) were observed by SEM and the results are shown in Fig. 10a and b, respectively. Comparing Fig. 4b with 10, it can be seen that the adsorbent surface became dense and pores decreased after the adsorption of tabersonine or impurities. This result proved that the adsorbates were adsorbed into its pores. Comparing Fig. 10a with 10b, it is clear that the pores in Fig. 10b decreased more remarkably. This result revealed that the adsorption capacity of impurities on PS-amino acid was higher compared to tabersonine. PS-amino acid exhibited a good ability to adsorb impurities but a poor ability to adsorb tabersonine in the extracts of Voacanga seeds.
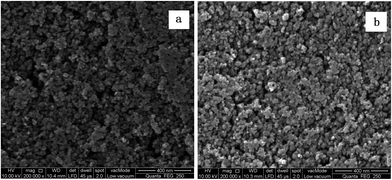 |
| Fig. 10 SEM characterization of MARs: (a) tabersonine absorbed on PS-amino acid and impurities absorbed on PS-amino acid (b). | |
The adsorption behaviors of tabersonine on commercial weak acid MARs
In this paper, the modified MARs contained carboxyl groups. Some properties of modified MARs are similar to the commercial weak acid MARs. In order to understand the differences between modified MARs and commercial weak acid MARs, four types of commercial weak acid MARs with carboxyl groups (D110, D113, D151, 724) have been applied to purify tabersonine from Voacanga seeds. The adsorption capacities of tabersonine on these commercial MARs were investigated and the results are shown in Fig. 11. It can be seen that the adsorption capacities of tabersonine on these commercial weak acid resins were lower compared to modified MARs. This is probably because the commercial weak acid MARs contains more carboxyl groups, which can not react with protonated tabersonine through acid–base interaction.
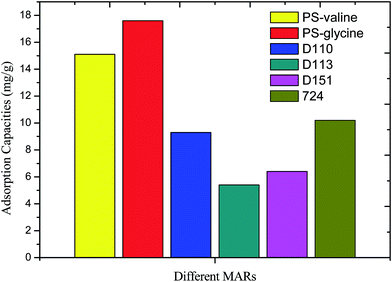 |
| Fig. 11 Adsorption capacities of tabersonine on different weak acid MARs. | |
The dynamic test of these commercial weak acid MARs was also investigated. The purity of tabersonine in the effluent was recorded and shown in Fig. 9. It can be seen that the purities of tabersonine purified by commercial weak acid MARs were considerably lower compared to those purified by modified MARs. This result indicated that the commercial weak acid MARs showed low adsorption selectivity toward tabersonine and impurities in the extracts of Voacanga seeds. The efficiency of purification of tabersonine by these commercial MARs was low. Although the content of carboxyl group or the synthetic cost of the commercial weak acid MARs is superior to the modified MARs synthesized in this article, the low adsorption selectivity of the commercial weak acid MARs leads to the high cost of purification. Compared with the commercial weak acid MARs, the modified MARs synthesized in this paper was a good MARs to purify tabersonine.
The modified MARs showed high adsorption selectivity toward tabersonine and impurities in the extracts of Voacanga seeds. This is probably because the modified MARs contained amino acid groups and featured special structures. As the components of impurities in the extracts were complex, the adsorption mechanism of impurities on modified MARs was not fully understood. Further investigation is proceeding to clarify the adsorption mechanism of impurities on modified MARs.
Recycling ability of the MARs
The PS-valine and PS-glycine were repeatedly used five times for the continuous adsorption and desorption of extracts of Voacanga seeds. After the adsorption, 50 mL of 80% of ethanol (v/v) was applied as the desorption reagent. The leaking volumes (purity of tabersonine > 90%) of the extracts on PS-amino acid for every time are shown in Fig. 12. After reusing the PS-amino acid five times, the leaking volumes of the extracts on PS-valine and PS-glycine decreased to approximately 2.4 BV and 2.0 BV, respectively. The results revealed that the PS-amino acid exhibited considerable recyclability.
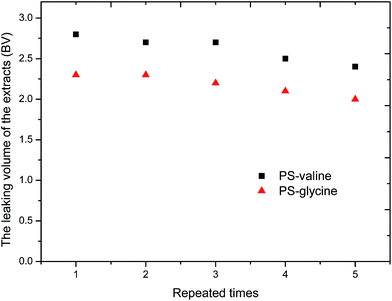 |
| Fig. 12 Effect of the regeneration cycles on the leaking volume of the extracts on PS-amino acid. | |
Conclusion
In summary, high purity of tabersonine was obtained using MARs functionalized with amino acids. The adsorption behaviors of tabersonine on MARs were investigated. The results indicated that the pseudo second-order rate equation was more appropriate for characterizing the kinetic data and the Freundlich model was more suitable for fitting the equilibrium data. Moreover, the results also revealed that the maximum adsorption capacity of MARs towards tabersonine reduced to 14 mg g−1 from 254 mg g−1 after functionalization with valine. Reasons for the low adsorption capacity of tabersonine on PS-amino acid were discussed. The results of dynamic adsorption and desorption tests, SEM images, and HPLC analysis revealed that the PS-amino acid had high adsorption selectivity toward tabersonine and impurities in the extracts of Voacanga seeds, and they were feasible to purify tabersonine from Voacanga africana seeds. Tabersonine was efficiently purified with the purity above 90%. Further investigation is proceeding to clarify the mechanism of adsorption of impurities on modified MARs. The reusability of the PS-amino acid was investigated and the PS-amino acid exhibited considerable reusability. This work provided a promising method to purify tabersonine.
References
- M. I. Maurice, Handbook of African Medicinal Plants, CRC Press, Inc., Florida, 1993 Search PubMed.
- A. R. Koroch, H. R. Juliani, D. Kulakowski, H. Arthur, J. Asante-Dartey and J. E. Simon, Voacanga africana: Chemistry, Quality and Pharmacological Activity, 2010, pp. 363–380 Search PubMed.
- G. A. Agbor, D. Kuate and J. E. Oben, Pak. J. Biol. Sci., 2007, 10, 537–544 CrossRef CAS.
- L. Tona, K. Kambu, N. Ngimbi, K. Cimanga and A. J. Vlietinck, J. Ethnopharmacol., 1998, 61, 57–65 CrossRef CAS.
- J. Gangoue-Pieboji, D. E. Pegnyemb, D. Niyitegeka, A. Nsangou, N. Eze, C. Minyem, J. N. Mbing, P. Ngassam, R. G. Tih, B. L. Sodengam and B. Bodo, Ann. Trop. Med. Parasitol., 2006, 100, 237–243 CrossRef CAS PubMed.
- Y. Rolland, N. Kunesch and J. Poisson, J. Org. Chem., 1976, 41, 3270–3275 CrossRef CAS.
- R. H. F. Manske, The Alkaloids: Chemistry and Physiology. the indole alkaloids, Academic Press, Inc, New York, 1965 Search PubMed.
- J. E. Poisson, US Pat., 3758478A, 1973.
- A. Nemes, C. Szantay Jr, L. Czibula and I. Greiner, Heterocycles, 2007, 71, 2347–2362 CrossRef CAS.
- A. Vas and B. Gulyas, Med. Res. Rev., 2005, 25, 737–757 CrossRef CAS PubMed.
- X. Liu, D.-L. Yang, J.-J. Liu, J. Zhou and N. Zhao, Chem. Pap., 2014, 68, 816–822 CrossRef CAS.
- Z. Xiaoyan, Z. Yan, L. Yan, S. Wanping and X. Xiaoqin, China Pat., CN101250188A, 2008.
- Z. Zhizhong, J. Lixin, C. Yi, G. Quanming and L. Zhihua, China Pat., CN 102050822A, 2011.
- K. Xu, D. L. Yang, J. J. Liu, X. Liu and C. Liu, Chem. Eng., 2012, 40, 64–68 CAS.
- Y. Li, J. Huang, J. Liu, S. Deng and X. Lu, J. Chem. Eng. Data, 2013, 58, 1271–1279 CrossRef CAS.
- H. Zhou, R. Jian, J. Kang, X. Huang, Y. Li, C. Zhuang, F. Yang, L. Zhang, X. Fan, T. Wu and X. Wu, J. Agric. Food Chem., 2010, 58, 12717–12721 CrossRef CAS PubMed.
- J. C. Crittenden, S. Sanongraj, J. L. Bulloch, D. W. Hand, T. N. Rogers, T. F. Speth and M. Ulmer, Environ. Sci. Technol., 1999, 33, 2926–2933 CrossRef CAS.
- P. Ren, X. Zhao, J. Zhang, R. Shi, Z. Yuan and C. Wang, React. Funct. Polym., 2008, 68, 899–909 CrossRef CAS PubMed.
- Y. Liu, Q. Bai, S. Lou, D. Di, J. Li and M. Guo, J. Agric. Food Chem., 2012, 60, 1555–1566 CrossRef CAS PubMed.
- S. Lou, Z. Chen, Y. Liu, H. Ye and D. Di, Ind. Eng. Chem. Res., 2012, 51, 2682–2696 CrossRef CAS.
- X. Geng, P. Ren, G. Pi, R. Shi, Z. Yuan and C. Wang, J. Chromatogr. A, 2009, 1216, 8331–8338 CrossRef CAS PubMed.
- V. B. Valodkar, G. L. Tembe, M. Ravindranathan, R. N. Ram and H. S. Rama, J. Mol. Catal. A: Chem., 2003, 202, 47–64 CrossRef CAS.
- D. Avnir and M. Jaroniec, Langmuir, 1989, 5, 1431–1433 CrossRef CAS.
- B. Sahouli, S. Blacher and F. Brouers, Langmuir, 1997, 13, 4391–4394 CrossRef CAS.
- M. Jaroniec, Langmuir, 1995, 11, 2316–2317 CrossRef CAS.
- S. Lou, Z. Chen, Y. Liu, H. Ye and D. Di, Langmuir, 2011, 27, 9314–9326 CrossRef CAS PubMed.
- S. Lou, Z. Chen, Y. Liu, H. Ye and D. Di, Langmuir, 2011, 27, 9314–9326 CrossRef CAS PubMed.
- D. Baybas and U. Ulusoy, J. Hazard. Mater., 2011, 187, 241–249 CrossRef CAS PubMed.
|
This journal is © The Royal Society of Chemistry 2014 |