DOI:
10.1039/C4RA09169H
(Paper)
RSC Adv., 2014,
4, 52100-52104
Tween20 surfactant effect on the electrocatalytic performance of porous carbon micro-spheres supported MnO2
Received
24th August 2014
, Accepted 7th October 2014
First published on 8th October 2014
Abstract
This study is concerned with the electrocatalytic evolution of oxygen at polyoxyethylene sorbitan monolaurate (Tween20) modified porous carbon micro-spheres (PCS) supported MnO2 catalyst in 0.1 M KOH solution. The Super P (SP) was used to improve the electrical conductivity of the catalyst. The electrochemical measurements revealed a significant enhancement of the electrocatalytic activity of the MnO2/PCS towards the oxygen evolution reaction (OER) upon the Tween20 modification. The onset potentials of the OER at the Tween20 modified MnO2/PCS particles electrode are more negative by about 0.162 V compared with the bare (i.e. unmodified) MnO2/PCS electrode. Tween20 is absorbed on the surface of MnO2/PCS and covers the entire surface of the MnO2/PCS particles homogeneously. The Tween20 plays a vital role as a catalytic mediator, which facilitates the ion transfer during the water oxidation into molecular oxygen and thus the OER is accomplished at less positive potentials.
1 Introduction
The development of an efficient catalyst for the oxidation of H2O to molecular oxygen is a critical component for solar fuel production systems, as it provides available source of electrons for hydrogen production or the direct conversion of carbon dioxide to liquid fuel.1–4 Europium, ruthenium and platinum as noble catalysts have higher catalytically activity and stability.5,6 However, these elements are expensive and scarce, thus making them unsuitable to be used on a large scale. So far a series of transition metal oxides such as Fe, Mn, Ni and Co have been reported to be catalytically active,7,8 but these transition metal oxides have low electronic conductivity and poor wettability. In order to improve the catalytic efficiency to a reasonably high extent, new catalyst supports are recently produced by different methods.9–15 Carbon materials (CNT and graphene), gold and other metals have been proposed as favorable electron conductors and ideal materials supporting nanosized metallic particles in the electrodes for electrocatalysis. The groups of Bell16 and Dai17 reported the OER activity of Co3O4 nanocrystals are significantly enhanced by anchoring them on graphene and Au support. Chen18 et al. reported the MnO2 nanorod arrays growing vertically directly on surface of Ti foil improved OER activity significantly.
The preceding discussions suggest that the transition metal oxides with improved conductivity can present high catalytic activity for OER. A simple way to achieve this goal would be to add conductive agent. However, electron transfer and ion diffusion are simultaneous in the process of OER. The ion can not rapidly reach the surface of catalyst resulting in great interface resistance between the electrolyte and catalyst due to the poor wettability of metal oxide.
Surfactant is a kind of organic compounds that can significantly reduce the surface tension and surface state of the system. The unique amphiphilic nature of surfactant makes it easy to occur at the electrode/solution interface adsorption, forming a layer of orienting thin film, and thus influence the diffusion process and electrochemical process of the material. The surfactants are widely used in the field of electrochemistry and electrical analysis chemistry.19,20 In order to improve the hydrophilic ability, the materials were treated by surfactants to achieve its surface modification and improve its wettability.
In this work, we added SP into catalyst ink to reduce the electron transfer resistance in OER process. Then, the surfactant Tween20 is introduced to modify MnO2/PCS to enhance the surface wettability of the catalyst in electrolyte.
2 Experimental
2.1. Synthesis of porous carbon micro-spheres (PCS)
Commercial food-grade corn starch was obtained from Zhongshan Mei WJ Co. Ltd. (Zhongshan, China), the other reagents were of analytical purity and were used without further purification. First, porous carbon micro-spheres were prepared by our previous method.21 The procedure of preparing the porous starch was similar with (ref. 22). 100 g corn starch was immersed in 150 mL HAc–NaAc buffer solution (pH ≈ 4.7) with stirring to form a starch suspension. Next, the complex enzyme of glucoamylase and α-amylase (2 g, 3
:
1, w/w) was added to the suspension. Following that, the mixture was kept stirring for 24 h at 43 °C in a water bath. Then the resultant porous starch was filtrated from the mixture and washed several times with distilled water, and freeze-dried. The dried corn porous starch and Na2SnO3·4H2O were uniformly blended with the composition ratio 5
:
2 (w/w), by impregnating some distilled water. The suspension was treated with vacuum freeze drying technology for 14 h. Then the mixture was placed in tube furnace, ramped at 2 °C min−1 to 600 °C in N2 atmosphere from room temperature, and kept at 600 °C for 3 h, then cooled down to room temperature naturally. The porous starch carbon was washed with dilute nitric acid, distilled water for several times until the pH of the solution was near 7, and then filtered and dried.
2.2. Synthesis of MnO2/PCS (MP) hybrid materials
In a typical synthesis of MnO2/PCS hybrid nanomaterials, 11.55 g 50% Mn(NO3)2·H2O was dissolved 40 mL ethanol. Afterwards, 1 g porous starch carbon were dispersed in the above solution by ultrasonication for 10 min. This mixture was then transferred into a 200 mL beaker kept stirring for 12 h at 25 °C in a water bath. This mixture then filtered and natural dried. The dry powder then transferred into beaker for 1 h at 150 °C.
2.3. Synthesis of Tween20 modified MnO2/PCS particles (TMP) hybrid materials
The MnO2/PCS powder was dispersed in 1% of a Tween20/ethanol solution and stirring for 2 h. Afterwards, this mixture then filtered and dried at 80 °C for 5 h to obtain Tween20 modified MnO2/PCS particles (TMP).
2.4. Electrode preparation and electrochemical measurements
The electrocatalyst powders, polyvinylidene fluoride (PVDF) and SP were dispersed in N-methyl-2-pyrrolidone (NMP). The weight ratios (electrocatalyst
:
PVDF
:
SP = 2
:
1
:
1). The PVDF was used as binder, SP as conductive. Stirred the mixture evenly and then the catalyst ink was deposited on the surface of on the spectrum of pure graphite electrodes with a geometric area of 0.28 cm2 and dried at 80 °C for 1 h. To facilitate the distinction, containing SP and Tween20 of electrode was named ST, containing SP and MP of electrode was named SMP, containing SP and TMP of electrode was named STMP. The electrode of MP was not contained SP.
The electrocatalyst loading on the spectrum of pure graphite electrodes was normally controlled at 0.1 mg cm−2. The experiments were carried out at 25 °C controlled by a water-bath thermostat. All electrochemical measurements were carried out in a three-electrode cell using IM6 electrochemical workstation (Zahner-Elektrik, Germany). A platinum foil and Hg/HgO (0.1 M KOH) were used as the counter and reference electrodes.
2.5. Characterization
The crystallographic information of the products was investigated by powder X-ray diffraction (XRD, Rigaku, D/max 2500v/pc) with Cu-Ka1 radiation (l¼ 1.5406 Å). The 2θ range used in the measurements was from 5° to 90°. The structure and morphology of the products were investigated by a field emission scanning electron microscopy (SEM, Philips, FEI Quanta 200FEG). The surface area of the samples were investigated using physical adsorption of nitrogen at the liquid-nitrogen temperature (77 K) on an automatic volumetric sorption analyzer (SA3100 Beckman Coulter, USA). Fourier transform-infrared spectra (FT-IR) were measured between 4000 and 200 cm−1 using a KBr disc technique with a model FT-IR 310 Jasco spectrometer (Japan). In all spectra, the absorbance was represented versus wavenumber (cm−1).
3 Results and discussion
X-ray diffraction (XRD) patterns of the PCS (a) and MP composite (b) are shown in Fig. 1. Fig. 1(a) illustrates the XRD pattern of PCS prepared at 600 °C, there is a broad and weak peak at 26°, which indicates a highly disordered carbon system.23,24 The peaks of SnO2 are also indexed at 34°, which is the result of the reduction of the Na2SnO3 at 600 °C. The pattern of the a MP indicates that it is a typical amorphous structure, five weak peaks were assigned in 26°, 37°, 42.9°, 52.7° and 66°, and a strong peak in 37°, which was determined as pure MnO2 (JCPDS no. 44-0141).25
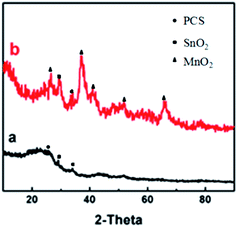 |
| Fig. 1 X-ray powder diffraction patterns of the samples, (a) PCS, (b) MP. | |
Fig. 2(a)–(c) and (d)–(f) are the SEM images of the PCS and MP composite, respectively. As seen in Fig. 2(a) and (b), the PCS show irregularly globular shape, possessing lots of micro-sized pores. The pore size of PCS decreased from about 0.91 μm to 0.53 μm, being measured from the SEM pictures of porous starch and porous carbon sphere by an electronic ruler. The macroporous structure can accept ion more easy than the micro–macroporous. Fig. 1(c) show the broke of PCS through high-energy ball milling is a hollow structure. The surface of the PCS can be increased by porous and hollow structure. The specific surface area of PCS is 8.6 m2 g−1. It can be obviously observed in Fig. 2(d)–(f), there are numberless manganese oxide particles about 30 nm (measured by an electronic ruler) dispersed uniformly onto the surface of PCS. The nanoparticles of MnO2 are not only existing on the surface of PCS but also going deep into internal surface as show the broke of ball (Fig. 1(e)) through high-energy ball milling, there by increasing more active site for OER.
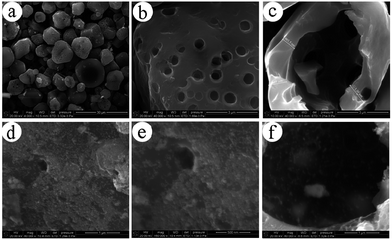 |
| Fig. 2 SEM images of the porous carbon micro-spheres (PCS) (a–c) and MP hybrid (d–f). | |
The vibrational spectroscopy is considered to be a very useful technique for detection of chemical and structural changes. Fig. 3 show the FT-IR spectra of various sample. The band at 1107 cm−1 can be assigned to the C–O–C stretching modes of the hydrophilic polyoxyethylene chain of Tween20, and the bands at 1250 cm−1 attributed to hydrophobic groups C–O stretching vibration and 1736 cm−1 to the C
O stretching vibration (Fig. 3(a)). As shown in Fig. 3(c) the bands at 1107 cm−1 can be attributed to the C–O–C stretching modes of the hydrophilic polyoxyethylene chain of Tween20, which does not exist in the FTIR spectrum of bare MP (Fig. 3(b)). This result suggests that hydrophobic group of the Tween20 surfactant molecules bonds with the atoms on the surface of the MP particles. The surface layer coating on the MP particles surface enhance the wettability of MP, and then promote ion diffusion during the OER process.
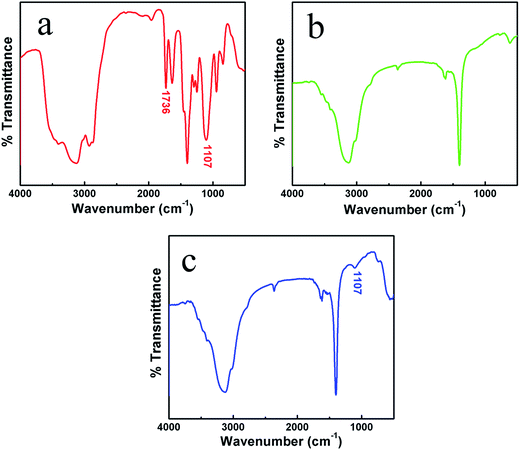 |
| Fig. 3 FT-IR spectra of samples: (a) Tween20, (b) MP, (c) TMP. | |
Fig. 4 shows CV curves of pure carbon, pure SP, MP and STMP electrode in 0.1 M KOH. The sweep rate is 0.005 V s−1 in the potential range from −0.1 to 1 V. There are no OER happens on the pure carbon, bare SP blank electrode MP electrode. However, one crucial condition for OER is the catalytic activity of the catalyst; another essential aspect is that the electrons can arrived rapidly at the surface of the catalyst. High current densities for OER on the SMP electrode, which indicates that SP can drive electrons rapidly transport to the surface of catalyst and electronic transmission is rate determining step of happen.
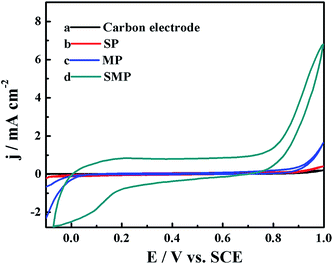 |
| Fig. 4 The CV curves of the anodes in 0.1 M KOH: (a) pure carbon, (b) SP blank, (c) MP and (d) SMP. The sweep rate was 0.005 V s−1 in the potential range from −0.1 to 1 V. | |
Fig. 5 shows CV curves of SMP and STMP electrode. The current has a sharply increase at 0.643 V on the STMP electrode lower than that of SMP (0.805 V). The result reveals that Tween20 can make OER easier for water split. However, the weak signal of OER on the ST electrode proves the Tween20 just enhances the OER instead of catalysis. The reverse sweep curve of TMP indicate it have a good stability in the process of OER. The STMP electrode shows a much higher current density than the MP in the whole potential range from −0.1 to 1 V due to Tween20 accelerating the diffusion of ions.
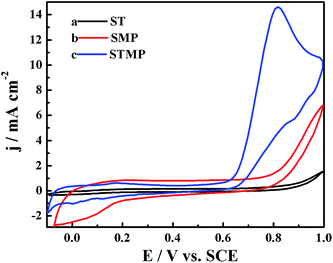 |
| Fig. 5 The CV curves of MP anodes in 0.1 M KOH: (a) ST electrode, (b) SMP and (c) STMP electrode. The sweep rate was 0.005 V s−1 in the potential range from −0.1 to 1 V. | |
To illustrate the enhancement effect of Tween20 in the OER process, we simulated the OER process, as indicated in Scheme 1. Firstly, the hydrophobic groups of surfactant Tween20 molecules is adsorbed on the surface of the MP particles and hydrophilic groups stretch outward into the water, as shown in the Scheme 1a and b. The hydrophilic group has a good affinity with water and then the wettability of MP is improved. As a result, the interface resistance between electrode and electrolyte is reduced and the current density of the OER is enhanced. The Tween20 molecules are absorbed on the surface and enhance the wettability of MP, which promotes the migration of ion to the MP surface and impels the OER proceeding.19
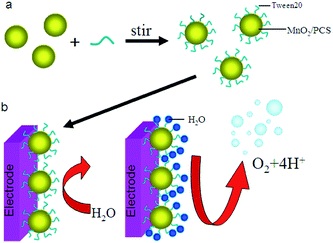 |
| Scheme 1 The mechanism of enhancement effect of Tween20 on the OER. | |
The stability of OER on the STMP electrode was investigated with chronoamperometry. The chronoamperometric curves are shown in Fig. 6. At the end of the test, the oxidation currents on the STMP and SMP electrodes are larger than that the bare MP electrode. Compared to the STMP, the current density on the bare MP and SMP anodes decay more quickly and tends to stabilize at 0.03 mA cm−2 and 0.06 mA cm−2 respectively at the end of the test. However, the current density on the STMP electrode decays slowly at the began of OER and retain 0.1 mA cm−2 after 30 min. The current decay indicates a deactivation of the electrodes. The results show that STMP has a high activity compared to the bare MP and a good stability for OER and the surfactant Tween20 enhances the MP activity.
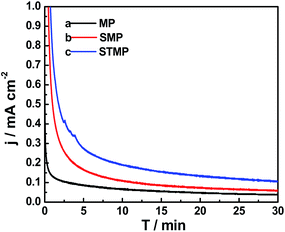 |
| Fig. 6 Chronoamperometric curves of anodes (a) MP, (b) SMP and (c) STMP in 0.1 M KOH with a potential of 0.7 V at 25 °C. | |
4 Conclusion
The electrical conductivity of catalysts is improved by added SP, and then the catalyst present catalyst activity of OER. MP modified with Tween20 resulted in a significant decrease in the anodic polarization required to sustain a specific current density compared with the bare MP in alkaline media. That is, a shift of the CV by about 0.643 V to the negative direction was obtained on the STMP, while a shift of about 0.805 V was achieved on the bare MnO2/PCS. Tween20 has been considered to enhance the generation of the oxygen gas via facilitating the ion transfer at various steps of the reaction pathway.
Acknowledgements
The authors would like to thank financial support from the National Natural Science Foundation of China (51364004), Guangxi Natural Science Foundation (2012GXNSFAA053214, 2013GXNSFDA019027 and 2013GXNSFAA019032) and Guangxi Experiment Centre of Science and Technology (LGZXKF201105).
References
- A. Fujishima and K. Honda, Nature, 1972, 238, 37–38 CrossRef CAS.
- A. J. Nozik, Annu. Rev. Phys. Chem., 1978, 29, 189–222 CrossRef CAS.
- A. J. Bard and M. A. Fox, Acc. Chem. Res., 1995, 28, 141–145 CrossRef CAS.
- N. S. Lewis and D. G. Nocera, Proc. Natl. Acad. Sci. U.S.A., 2006, 103, 15729–15735 CrossRef CAS PubMed.
- E. Fabbri, A. Habereder, K. Waltar, R. Kötz and T. J. Schmidt, Catal. Sci. Technol., 2014, 4, 3800–3821 CAS.
- A. R. Zeradjanin, A. A. Topalov, Q. Van Overmeere, S. Cherevko, X. X. Chen, E. Ventosa, W. Schuhmannc and K. J. J. Mayrhofer, RSC Adv, 2014, 4, 9579–9587 RSC.
- A. Sartorel, M. Carraro, F. M. Toma, M. Prato and M. Bonchio, Energy Environ. Sci., 2012, 5, 5592–5603 CAS.
- C. Xu, M. Lu, Y. Zhana and J. Y. Lee, RSC Adv., 2014, 4, 25089–25092 RSC.
- A. Sartre, M. Phaner, L. Porte and G. N. Sauvion, Appl. Surf. Sci., 1993, 70, 402–406 CrossRef.
- X. Q. Tong, M. Aindow and J. P. G. Tarr, Electroanal. Chem., 1995, 395, 117–126 CrossRef.
- A. M. Polcaro and S. Palmas, Electrochim. Acta, 1991, 36, 921–926 CrossRef CAS.
- C. K. Lai, Y. Y. Wang and C. C. Wan, Electroanal. Chem., 1992, 322, 267–278 CrossRef CAS.
- L. R. Radovic and F. Rodriguez-Reinoso, Carbon Materials in Catalysis, in Chemistry and Physics of Carbon, 1997, vol. 25, pp. 243–358 Search PubMed.
- D. J. Guo and H. L. Li, Electroanal. Chem., 2004, 573, 197–202 CAS.
- D. J. Guo and H. L. Li, J. Colloid Interface Sci., 2005, 286, 274–279 CrossRef CAS PubMed.
- B. S. Yeo and A. T. Bell, J. Am. Chem. Soc., 2011, 133, 5587–5593 CrossRef CAS PubMed.
- Y. Liang, Y. Li, H. Wang, J. Zhou, J. Wang, T. Regier and H. Dai, Nat. Mater., 2011, 10, 780–786 CrossRef CAS PubMed.
- S. Chen, T. Zhai, C. X. H. Lu, M. Z. Zhang, Z. Y. Li, W. Xu and Y. X. Tong, Int. J. Hydrogen Energy, 2012, 37, 13350–13354 CrossRef CAS PubMed.
- M. Plavsic, D. Krznaric and B. Cosovic, Electroanalysis, 1994, 6, 469–474 CrossRef CAS.
- S. Hu, K. Wu and H. Yi, Anal. Chim. Acta, 2002, 464, 209–216 CrossRef CAS.
- X. Y. Wang, H. Q. Wang, Q. F. Dai, Q. Y. Li, J. H. Yang, A. N. Zhang and Z. X. Yan, Colloids Surf., A, 2009, 346, 213–215 CrossRef CAS PubMed.
- W. R. Yao and H. Y. Yao. CN Patent 1546529, 2004.
- Z. H. Yi, Y. G. Liang, X. F. Lei, C. W. Wang and J. T. Sun, Mater. Lett., 2007, 61, 4199–4203 CrossRef CAS PubMed.
- Q. Wang, F. Y. Cao, Q. W. Chen and C. L. Chen, Mater. Lett., 2005, 59, 3738–3741 CrossRef CAS PubMed.
- R. R. Jiang, T. Huang, J. L. Liu, J. H. Zhuang and A. S. Yu, Electrochim. Acta, 2009, 54, 3047–3052 CrossRef CAS PubMed.
|
This journal is © The Royal Society of Chemistry 2014 |