DOI:
10.1039/C4RA09112D
(Paper)
RSC Adv., 2014,
4, 56112-56127
Investigation of synthesis, crystal structure and third-order NLO properties of a new stilbazolium derivative crystal: a promising material for nonlinear optical devices†
Received
22nd August 2014
, Accepted 8th October 2014
First published on 8th October 2014
Abstract
A new organic stilbazolium derivative crystal 2-[2-(3-hydroxy-4-methoxy-phenyl)-vinyl]-1-methyl-pyridinium naphthalene-2-sulfonate dehydrate (C25H23NO5S·2H2O) (VSNS) was synthesized successfully. Single crystals were grown in a mixed solvent of methanol–acetonitrile (1
:
1) using a slow evaporation method at room temperature. Solubility of the synthesized VSNS material was experimentally determined for various temperatures using a methanol–acetonitrile mixed solvent. A single crystal X-ray diffraction study confirmed the crystal structure and morphology of VSNS. The crystalline nature of the title material was analyzed by powder X-ray diffraction analysis, and the presence of expected functional groups and the molecular structure of VSNS was identified by FT-IR and 1H NMR spectroscopic studies. Optical absorption was recorded using UV-Vis-NIR spectral analysis, and linear optical constants such as the absorption coefficient, band gap, extinction coefficient, refractive index and reflectance were calculated. The luminescence property of the crystal grown showed green emission radiation. The thermal stability of the crystal was analyzed by TG–DTA studies, and the hardness, Meyer index, yield strength, and elastic stiffness constant were estimated using a Vickers microhardness tester. Layer growth pattern was observed in chemical etching studies using a Carl Zeiss optical microscope at 50× magnification. Laser damage threshold energy was measured using an Nd:YAG laser (1064 nm). Variation of the dielectric response of the grown crystal was studied at room temperature. The third-order nonlinear optical property of VSNS was investigated in detail using a Z-scan technique with He–Ne laser at 632.8 nm. The second-order molecular hyperpolarizability γ of the crystal grown was 7.986 × 10−34 esu. This encouraging result of the Z-scan studies suggests that the VSNS crystal is a candidate material for photonics devices, optical switches, and optical power limiting applications.
1. Introduction
Nonlinear optical (NLO) materials are a new frontier of science and development for technologies of photonics.1,2 These materials influence potential applications such as information processing, optical computing,3 telecommunication,4–6 3D optical data storage,7,8 lasers, and optical power limiting.9 Therefore, synthesis of new organic compounds with large nonlinear optical properties has attracted the interest of many research groups, with primary interest in the possibilities for reversibly switching NLO systems.10,11 Compared with inorganic materials, organic materials have properties such as short response time, small dialectic constant, and large electro-optic (EO) coefficient.12 Organic single crystals have delocalized π-electrons with conjugated double bond systems. Hence, they are a source of fast and large macroscopic nonlinearities, have high optical quality, superior long-term orientational, high packing densities, and larger photochemical stabilities.13,14 Effective NLO organic materials contain suitable electron donor and electron acceptor groups with π-conjugated electronic bridge flanking either end of the system.15 In particular, the strong delocalization of π-electrons causes large molecular hyperpolarizability, and the nonlinear susceptibility in the organic molecule can lead to significant enhancement of the third-order nonlinear optical properties of the materials.
For the past few years, third-order nonlinear optical materials have attracted attention because of their application in ultrafast optical switching and devices.16 Among the various techniques developed theoretically and experimentally for measurement of NLO properties, the Z-scan technique is a simple and effective experimental method to measure the intensity dependence of third-order optical nonlinearities of the materials.17,18 It is capable of measuring information not only on sign and magnitude, but also on the real (nonlinear refraction) and imaginary (nonlinear absorption) parts of the material. A high ratio of real and imaginary parts of third-order optical nonlinearities is essential for effective optical switching applications.19
In this paper, we report the growth of a new VSNS crystal from the stilbazolium family. The crystals grown were characterized using different instrumentation techniques to check their suitability for device fabrications. The crystal structure was confirmed by single crystal X-ray diffraction studies, and the crystals were also systematically characterized by X-ray powder diffraction, Fourier transform infrared, nuclear magnetic resonance, optical, luminescence, thermal properties, mechanical behavior, laser damage threshold dielectric, and chemical etching studies. In the last section, third-order nonlinear optical properties of the crystal were investigated using the Z-scan technique.
2. Experimental procedures
2.1. Material synthesis
All high-purity commercially available reagents in Scheme 1 were purchased and used without further purification. VSNS was synthesized via a two-step synthesis route. In the first step, 2-[2-(3-hydroxy-4-methoxyphenyl)-vinyl]-1-methyl-pyridinium iodide (A) was synthesized (condensation reaction method) by mixing a stoichiometry ratio of 1,2-dimethyl pyridinium iodide and isovanillin in hot methanol, with piperidine (few drops) added as a catalyst. The resulting solution was refluxed for 10 h to form a yellow salt. The resultant A was filtered off and washed with diethyl ether to remove the unreacted material, then purified by recrystallization in methanol. In the second step, VSNS was synthesized by counter-anion exchange reaction of A with sodium 2-naphthalene sulfonate as shown in Scheme 1. The resulting material (A) was dissolved in deionized water (40 mL), treated with a saturated solution of sodium 2-naphthalene sulfonate and further heated for 1 h at 80 °C. A precipitate appeared when the solution was cooled to ambient temperature, and this was isolated by filtration and dried. The purity of the title salt was increased three times successively by recrystallization using methanol solution.
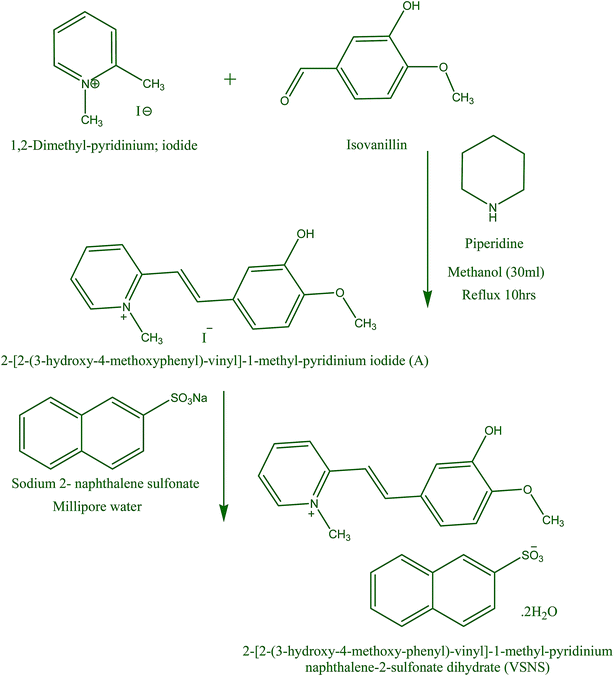 |
| Scheme 1 Synthesis of VSNS. | |
2.2. Solubility, crystal growth, and morphology
Solubility study is an important parameter to help achieve desired concentration of material, growth method, and the growth rate of crystal, dependent on temperature. Thermodynamically, this means that in a saturated solution the chemical potential of the pure solid is equal to the chemical potential of the same solute.20 The solubility of VSNS using purified salt was measured by dissolving the solute in 100 mL of mixed solvent of methanol–acetonitrile (1
:
1) at different temperatures in the range 30–45 °C with 5 °C intervals. A solubility study was carried out in an ultra-cryostat bath with a control accuracy of ±0.01 °C. Initially, the cryostat was maintained at 30 °C and continuously stirred with a motorized magnetic stirrer. The purified salt was added step by step to 100 mL of methanol–acetonitrile mixed solvent in an airtight container on the bath until supersaturation was attained. The equilibrium concentration of the supernatant liquid of the solute was estimated gravimetrically. In this experiment, the masses of the solvent and solute were weighed using an analytical balance (accuracy ±0.0001 g). The equilibrium concentration of the amount of solute dissolved in 100 mL at 30–45 °C was estimated in the same manner. The solubility curve of VSNS at different temperatures shows it has good solubility in methanol–acetonitrile mixed solvent (Fig. 1). It was observed that solubility of VSNS increases dramatically with increasing temperature.
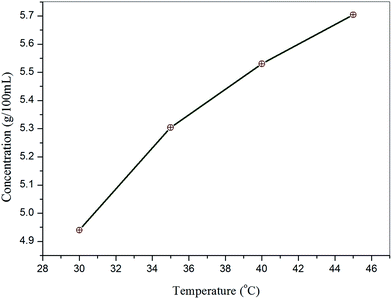 |
| Fig. 1 Solubility curve of VSNS as a function of temperature. | |
A saturated solution of the purified salt of VSNS at 40 °C was prepared using the mixed solvent of methanol–acetonitrile (1
:
1) according to the determined solubility data. The prepared solution was filtered and transferred to a 150 mL beaker, which was tightly covered with aluminum foil to control the rate of evaporation of the solvent. The beaker was kept in a constant temperature water bath at 40 °C. Crystallization was allowed by the temperature of the bath by reducing the temperature at a rate of 0.20 °C per day. After 35 days, good quality single crystals of VSNS with dimensions 7 × 4 × 2.5 mm3 were obtained. A photograph of the as-grown crystals of VSNS is shown in Fig. 2. The morphology of VSNS was indexed by the WinXMorph software program using XRD data from a single crystal (cif format) as input.21 The indexed morphology of VSNS is shown in Fig. 3. It was found that growth rate of VSNS elongated along the crystallographic b-axis compared with the other two growth directions.
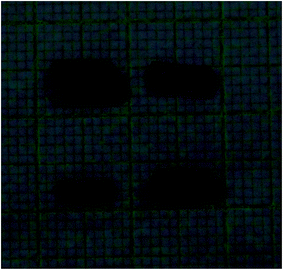 |
| Fig. 2 Photograph of VSNS crystal grown by slow evaporation. | |
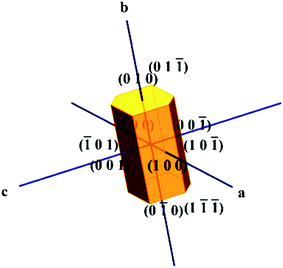 |
| Fig. 3 Morphology of VSNS crystal. | |
3. Results and discussion
3.1. Powder X-ray diffraction and single crystal studies
The fine powder of grown crystal was subjected to a Bruker X-ray diffractometer with CuKα radiation (λ = 1.540598 Å) to demonstrate the crystalline nature and purity. The sample was recorded over the range 10–60° at a scanning rate of 0.02° s−1 (2θ) at room temperature. The various planes of reflection at specific 2θ angles were indexed using the Powder X program. The experimentally observed XRD peaks are in good agreement with the single crystal XRD pattern based on the single crystal structure of VSNS as shown in Fig. 4. The appearances of well-defined sharp Bragg's peaks at specific 2θ angles confirm good crystalline property of the grown VSNS crystal.
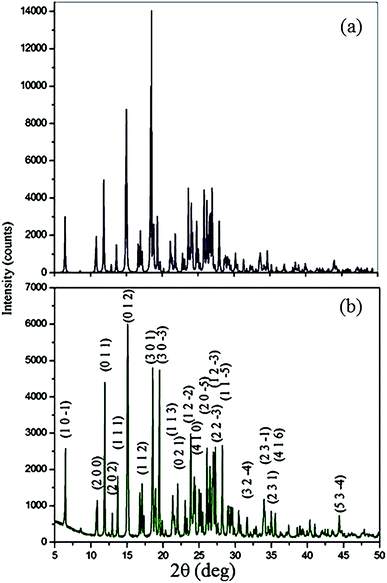 |
| Fig. 4 Powder XRD patterns of VSNS: (a) simulated and (b) experimental. | |
A suitable crystal of VSNS was subjected to single crystal X-ray structure studies using a Bruker Kappa APEX II diffractometer with MoKα (λ = 0.71073 Å) radiation. The grown crystal structure was determined by direct methods using SHELXS-97 and refined by full-matrix least squares against F2 using the SHELXL-97 software program.22 It was observed that the compound crystallized in centrosymmetric space group P21/n consisted of cation and anion with monoclinic crystal system and R factor equal to 0.0331. The ORTEP view of VSNS is shown in Fig. 5. A summary of the crystal data is given in Table 1. The maximum deviation from the plane of cation and anion molecule appears at C3 [0.101(2) Å] and C22 [−0.005(2) Å] and N1 [0.0358(2) Å]. It is clearly shown in VSNS structure that two planes of anion and cations are near to perpendicular with 83.8(1)°. The torsion angle value at C5–C6–C7–C8 = 178.89(15)° shows that the phenyl group adopted a trans conformation. There is a short contact between H4 and H7 because of the trans conformation and increase in bond angle from the standard value at C5 and C1 [C4–C5–C6 = 123.91(15)°]. The methoxy group [O2 = −0.0128(1) and C15 = 0.059(3)] slightly deviated from the mean plane of ring atoms C8, C9, C10, C11, C12 and C13 in VSNS.
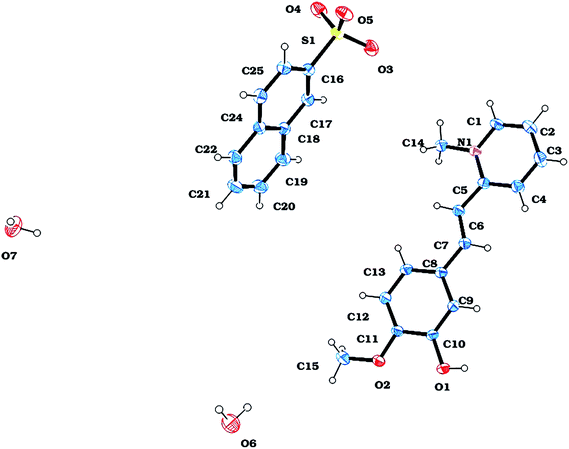 |
| Fig. 5 ORTEP view of VSNS. | |
Table 1 Crystal data and structure refinement for VSNS
Empirical formula |
C25H27NO7S |
Formula weight |
485.54 |
Temperature |
298(2) K |
Wavelength |
0.71073 Å |
Crystal system |
Monoclinic |
Space group |
P2(1)/n |
Unit cell dimensions |
a = 16.9655(8) Å, α = 90° |
b = 8.3200(4) Å, β = 106.275(2)° |
c = 17.1232(8) Å, γ = 90° |
Volume |
2320.14(19) Å3 |
Z |
4 |
Density (calculated) |
1.390 mg m−3 |
Absorption coefficient |
0.187 mm−1 |
F(000) |
1024 |
Crystal size |
0.25 × 0.20 × 0.15 mm3 |
Theta range for data collection |
1.49 to 25.00° |
Index ranges |
−18 ≤ h ≤ 19, −8 ≤ k ≤ 9, −16 ≤ l ≤ 20 |
Reflections collected |
13 898 |
Independent reflections |
3860 [R(int) = 0.0176] |
Completeness to theta = 25.00° |
94.4% |
Absorption correction |
None |
Max. and min. transmission |
0.9725 and 0.9548 |
Refinement method |
Full-matrix least-squares on F2 |
Data/restraints/parameters |
3860/0/329 |
Goodness-of-fit on F2 |
1.047 |
Final R indices [I > 2sigma(I)] |
R1 = 0.0331, wR2 = 0.0832 |
R indices (all data) |
R1 = 0.0389, wR2 = 0.0887 |
Largest diff. peak and hole |
0.249 and −0.349 e Å−3 |
In the crystal, packing of VSNS is stabilized predominantly by O–H⋯O and C–H⋯O hydrogen bonds and aromatic interactions. There is a network chain associated with water molecules and the neighbor asymmetric residue unit running through the ‘AC’ plane axis. It is also noted that O–H⋯O networks are involved in translational units along the b-axis through donor and acceptor atoms of O1⋯O7*⋯O6**⋯O3*** (stars are translational units). The potential hydrogen bonds to sustain the structure of VSNS are listed in Table 2. Selected bond lengths and angles of VSNS are given in Table 3. There are well-defined π–π interactions between symmetry generated pairs of the entities involved in the crystal packing, particularly H12 of C12 forming π-interaction with the centroid of other ring systems of C16–C17–C23 and C24–C25 with an angle of 151.7°. A three-dimensional view of the molecular packing diagram of VSNS is shown in Fig. 6.
Table 2 Hydrogen bonds for VSNS [Å and deg]
D–H⋯A |
d(D–H) |
d(H⋯A) |
d(D⋯A) |
<(DHA) |
O(6)–H(2OA)⋯O(4)#1 |
0.82(3) |
2.03(3) |
2.835(2) |
164(3) |
O(6)–H(2OA)⋯S(1)#1 |
0.82(3) |
2.87(3) |
3.6196(16) |
152(2) |
O(6)–H(1OA)⋯O(3)#2 |
0.87(3) |
1.89(3) |
2.730(2) |
161(2) |
O(7)–H(3OA)⋯O(5)#3 |
0.75(3) |
2.04(3) |
2.785(2) |
170(2) |
O(7)–H(3OA)⋯S(1)#3 |
0.75(3) |
2.97(3) |
3.677(2) |
157(2) |
O(7)–H(4OA)⋯O(6)#4 |
0.86(3) |
1.86(3) |
2.714(2) |
177(3) |
O(1)–H(5OA)⋯O(7)#5 |
0.86(2) |
1.77(2) |
2.638(2) |
179(2) |
Table 3 Bond length and angles for VSNS
Atoms |
Length |
Atoms |
Angles |
C(1)–N(1) |
1.350(2) |
N(1)–C(1)–C(2) |
121.42(16) |
C(2)–C(3) |
1.381(3) |
C(4)–C(3)–C(2) |
119.31(18) |
C(3)–C(4) |
1.370(2) |
C(3)–C(4)–C(5) |
121.51(17) |
C(4)–C(5) |
1.390(2) |
N(1)–C(5)–C(4) |
117.04(14) |
C(5)–N(1) |
1.369(2) |
N(1)–C(5)–C(6) |
119.04(14) |
C(5)–C(6) |
1.449(2) |
C(4)–C(5)–C(6) |
123.91(15) |
C(6)–C(7) |
1.331(2) |
C(7)–C(6)–C(5) |
123.61(16) |
C(7)–C(8) |
1.455(2) |
C(13)–C(8)–C(9) |
118.03(14) |
C(8)–C(13) |
1.385(2) |
C(13)–C(8)–C(7) |
123.53(14) |
C(8)–C(9) |
1.401(2) |
C(9)–C(8)–C(7) |
118.44(15) |
C(12)–C(13) |
1.378(2) |
C(10)–C(9)–C(8) |
121.58(15) |
C(14)–N(1) |
1.474(2) |
O(1)–C(10)–C(11) |
117.09(14) |
C(15)–O(2) |
1.413(2) |
C(13)–C(12)–C(11) |
120.62(16) |
C(16)–C(17) |
1.364(2) |
C(12)–C(13)–C(8) |
120.88(15) |
C(16)–C(25) |
1.407(2) |
C(17)–C(16)–C(25) |
120.88(15) |
C(17)–C(18) |
1.414(2) |
C(16)–C(17)–C(18) |
120.22(15) |
C(18)–C(19) |
1.416(2) |
C(17)–C(18)–C(19) |
122.48(16) |
C(18)–C(23) |
1.417(2) |
C(17)–C(18)–C(23) |
118.84(15) |
C(19)–C(20) |
1.361(3) |
C(20)–C(19)–C(18) |
120.49(19) |
C(20)–C(21) |
1.395(3) |
C(19)–C(20)–C(21) |
120.72(19) |
C(21)–C(22) |
1.356(3) |
C(22)–C(21)–C(20) |
120.40(18) |
C(22)–C(23) |
1.413(2) |
C(24)–C(23)–C(22) |
121.99(16) |
C(23)–C(24) |
1.411(2) |
C(24)–C(23)–C(18) |
119.21(15) |
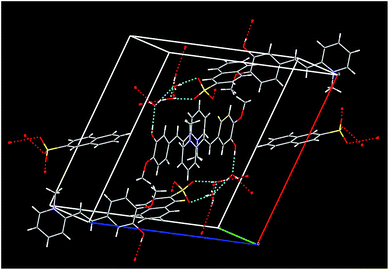 |
| Fig. 6 Molecular packing diagram of VSNS. | |
3.2. FT-IR spectral studies
The vibrational spectral analysis of VSNS was recorded to confirm the various modes of functional groups present in the grown crystals using a Shimadzu IRAffinity spectrometer in the scan region 4000–400 cm−1, as shown in Fig. 7. The absorption bands at 3375 and 3255 cm−1 are a result of the O–H stretching vibrations on isovanillin and the presence of a water molecule in the title compound. The aromatic C–H stretching mode was observed at 3062 cm−1 in the spectrum. The absorption peaks obtained at 2956 and 2835 cm−1 are the result of alkyl C–H stretching vibrations. The aromatic ring C
C stretching vibrations are assigned to peaks at 1618 cm−1, 1566 cm−1 and 1502 cm−1. In-plane O–H group deformation vibrations in the isovanillin ring were reported near 1257 and 1436 cm−1.23 The peak at 1388 cm−1 was assigned to C–N stretching and CH2 bending vibrations. The stretching frequency of S
O at 1178 cm−1 clearly indicated the presence of SO3 group in the crystal lattice. The absorption band at 1031 cm−1 established formation of the title compound by C–H olefin bond. The presence of a methoxy (O–CH3) group indicated the stretching mode in the region 1000–1100 cm−1. The very weak intensity band observed at 972 cm−1 is caused by C–H deformation mode of the title molecule.24 Thus, the assignments of the various absorption frequencies confirm formation of VSNS crystal.
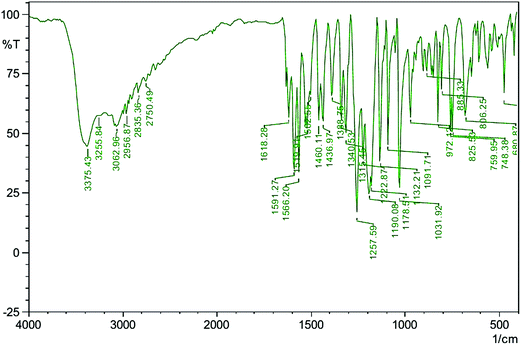 |
| Fig. 7 FT-IR spectrum of VSNS. | |
3.3. 1H NMR spectral studies
NMR spectroscopy is considered to be one of the most useful analytical techniques for determining the structure and nature of the immediate environment of a molecule. The experiments were performed on a Bruker instrument operating with a 400 MHz spectrometer. Fig. 8 shows the 1H NMR spectra of the title compound in DMSO-d6, with a quintet chemical shift at 2.540 ppm. Also, the chemical shift of a water molecule in DMSO-d6 occurred at 3.423 ppm. A methyl proton attached to a pyridine ring (N–CH3) gives a singlet peak at 4.363 ppm. Protons of the methyl group in an aldehyde ring (O–CH3) show a singlet peak at 3.882 ppm. Chemical shifts of two doublets were observed at 7.076 ppm and 7.751 ppm, caused by two olefin hydrogens (HC
CH). The pyridinium ring gives peaks for two doublets at 8.444 ppm and 8.865 ppm, corresponding to the third and sixth places of hydrogens (C–H), neighbors of the (N–CH3) bond. Also, one triplet peak at 8.483 ppm, and one doublet at 7.772 ppm are caused by the fourth and fifth position hydrogens. Aromatic protons of second and sixth places showed multiplet peaks in the range from 7.300 ppm to 7.394 ppm. Three multiplet peaks were observed at 7.550 ppm, 7.858 ppm, and 7.934 ppm, caused by the ring hydrogens of naphthalene. The singlet chemical shift at 8.194 ppm was assigned to the first place hydrogen of the naphthalene. A proton of the hydroxyl group shows a singlet peak at 9.343 ppm. Thus, the observed chemical shift establishes the molecular structure of the title compound.
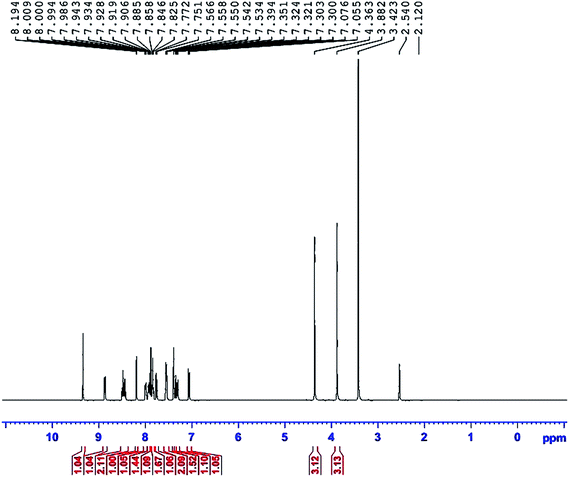 |
| Fig. 8 1H NMR spectrum of VSNS. | |
3.4. UV-Vis-NIR spectral analysis
The optical transmittance study is used for identifying the optical transmission range and cutoff wavelength of crystals. Also, the study can aid understanding of electronic transition states, during interaction of light with a molecule. The optical absorption spectrum of VSNS was recorded in the wavelength range 190 nm to 1100 nm with a crystal of thickness 1 mm using an ELICO SL 218 double beam UV-Vis-NIR spectrometer. From the absorption spectrum (Fig. 9), the absence of transmission of light in the UV region was revealed, as absorption of UV light involves strong electronic transitions from the ground state to the excited state (higher energy states). The optical cutoff wavelength of the VSNS crystal was found to be 390 nm, and the crystals had good optical transmittance percentage between 390 and 1100 nm (Vis-NIR region). For optoelectronic and NLO applications, the crystal must have good transparency in the Vis-NIR region.25 The lower cutoff wavelength of the grown crystal is caused by π–π* orbital electronic transitions through the stilbazolium chromophore. The band-gap energy at the cutoff wavelength was calculated using the relation:where h is the Planck constant, c is the velocity of light, and λ is the cutoff wavelength of the crystal. Hence, the calculated value of band-gap energy of the grown crystal was found to be 3.17 eV.
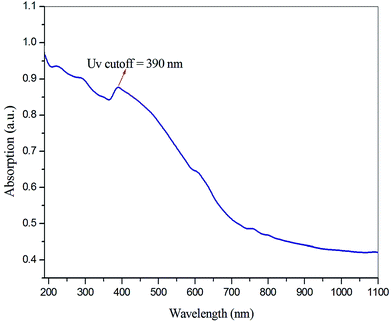 |
| Fig. 9 UV-Vis-NIR spectrum of VSNS. | |
3.5. Determination of energy band gap, extinction coefficient, and refractive index
The optical absorption coefficient (α) was determined using the following formula: |
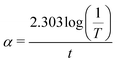 | (2) |
where T is the transmittance and t is the thickness of the crystal. As a direct band gap (Eg) has been determined from the transmission spectra, the optical absorption coefficient of the crystal (α) near the absorption edge is given by the following relation: |
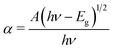 | (3) |
where A is a constant, Eg the optical band gap of the crystal, h the Planck constant, and ν is the frequency of incident photons. The plot of transmittance and reflectance for VSNS is shown in Fig. 10a. The band gap of the VSNS crystal was estimated by plotting (αhν)2 against the photon energy (hν) (Tauc's plot) as shown in Fig. 10b. The band-gap energy (Eg) of the grown crystal was estimated by exploring a straight line in the linear region near the onset of the absorption edge to the energy axis (αhν)2 = 0. From Fig. 10b, the estimated band-gap energy was found to be 3.252 eV. The wide band gap of the VSNS crystal confirms the large transmittance window in the visible region.26 The extinction coefficient (K) can be obtained from the following relation: |
 | (4) |
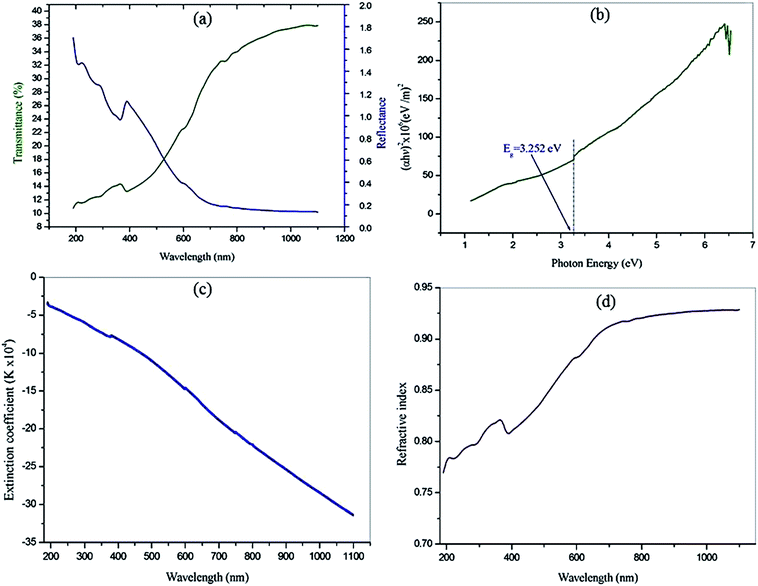 |
| Fig. 10 (a) The plot of transmittance and reflectance for VSNS. (b) Plot of (αhν)2 against the photon energy (hν). (c) Wavelength dependence of extinction coefficient (K). (d) Refractive index (n0). | |
The plot of extinction coefficient (K) as a function of wavelength is shown in Fig. 10c. From the graph, it is observed that the extinction coefficient (K) value increases with an increase in photon energy.
The transmittance (T) is given by:
|
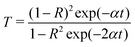 | (5) |
The reflectance (R) in terms of the absorption coefficient can be derived from the following relation:
|
 | (6) |
It is observed that reflectance (R) increases with an increase in photon energy, as shown in Fig. 10a.
|
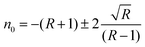 | (7) |
The linear refractive index (
n0) of the crystal was determined in terms of reflectance (
R) using the relation. The linear refractive index (
n0) of the grown crystal was found to be
n0 = 0.9284 at 1100 nm, and this was used to calculate the third nonlinear optical susceptibility of the material.
Fig. 10d shows the variation refractive index (
n0) as a function of photon energy for VSNS crystals. The optical studies revealed that the VSNS crystal possesses good optical behavior for use in device applications.
Thus, the extinction coefficient (K) and refractive index (n0) are dependent on the wavelength. It is understood that a higher value of photon energy will enhance the optical efficiency of the material.
3.6. Luminescence studies
In general photoluminescence (PL) in solids, the molecules are excited by external energy from a light source and the excitation state is released as luminescent light.27 To identify the nature of emission band of VSNS, the excitation spectrum was recorded using a using F-7000 FL spectrophotometer in the range of 400–750 nm at room temperature. The emission spectrum of the sample with excitation wavelength at 390 nm is shown in Fig. 11. From the emission spectrum, the title compound exhibits a strong and broad emission peak at 553 nm which is related to the π–π* localized electron between the donor and acceptor groups through stilbazolium chromophore (π-conjugated double bond), which lead to its luminescence characteristic nature. Thus, this result indicates that the VSNS crystal has a stronger photon energy absorption band in the UV region at 390 nm, and a weaker green emission radiation at 553 nm, which reveals its high purity with high degree of crystallinity of VSNS crystals.28 It this defect-free, good crystallinity is likely to be useful in optoelectronic device applications.
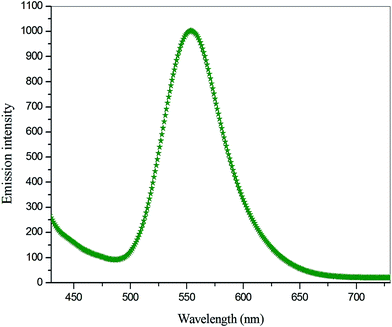 |
| Fig. 11 Emission spectra of VSNS. | |
3.7. Thermogravimetric and differential thermal analysis
To study the phase transition, melting point, and various stages of decomposition of the crystal system, thermogravimetric (TG) and differential thermal analysis (DTA) of grown crystals were carried out in the temperature range 35–550 °C using Netzsch STA-449F3 analyzer equipment in a N2 atmosphere. An initial mass of 4.340 mg of the powder sample of VSNS was used for the analysis. The obtained TG–DTA curve of the title compound is shown in Fig. 12. From the curve, it is noted that there is no weight loss and phase transition between the 35 °C and 196.6 °C, and it is evident that the title compound is very stable up to 196.6 °C. The title compound decomposes in three stages: during the first stage there is weight loss of 3.56% of the initial mass between 196.6 °C and 321.6 °C because of dehydration of water molecules and volatile substances. In the next stage, maximum decomposition occurs with weight loss of 60.74% starting at 321.6 °C. Beyond 387.5 °C weight losses occur again up to 549.7 °C, concluding complete decomposition of the title compound. In the DTA trace, the endothermic peak at 204.3 °C represents the melting point of VSNS crystal, and this is followed by rapid decomposition in the DTA peak at 371.6 °C, where the complete composition of VSNS crystal in the gaseous stage coincides with the maximum weight losses in TG curve. The residual mass of the title compound is 15.19% charred as carbon at 549.9 °C. Thus, the TG–DTA studies establish suitability for laser experiment applications of this crystal with texture to 196.6 °C.
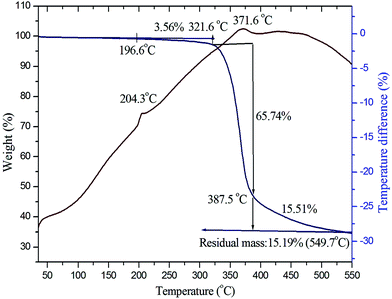 |
| Fig. 12 TG–DTA curves of VSNS. | |
3.8. Microhardness studies
Microhardness testing is a useful method for calculating mechanical properties of materials such as hardness number, fracture behavior, elastic stiffness constant, yield strength, and plasticity.29–31 Hardness properties of the material depend primarily on the crystal structure.32 Mechanical stability of a material is essential in fabrication of devices. The hardness measurements of the VSNS crystal were made on the well-defined prominent plane (1 0 0) using an MH-112 Vicker's hardness tester fitted with a pyramid indenter. An indentation time of 10 s was used for all indentations for all loads ranging from 10 to 100 g. The Vicker's hardness value of each load was calculated using the expression |
Hv = 1.8544P/d2 kg mm−2
| (8) |
where P is the load applied in kilograms (kg) and d is the average diagonal length of the indentation impression in µm. A plot drawn between the loads and the corresponding hardness number is depicted in Fig. 13. It is shown that the hardness number increases with the increase of applied load, which refers to the crystal exhibits reverse indentation size effect (RISE) behavior.33 The relation between load P and average indentation d is given using Meyer's law,34 and, as depicted in Fig. 14a, gives a straight line. The estimated value of Meyer index (n) determines the work hardening coefficient for VSNS crystal and is equal to 2.70, concluding that the grown crystal falls into the soft material category, in good agreement with the reverse indentation size effect (RISE).35 From a Meyer index value n > 2, the yield strength σy of the crystal can be calculated using the expression: |
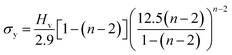 | (9) |
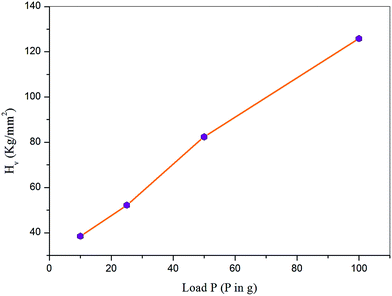 |
| Fig. 13 Variation of Hv with applied load P. | |
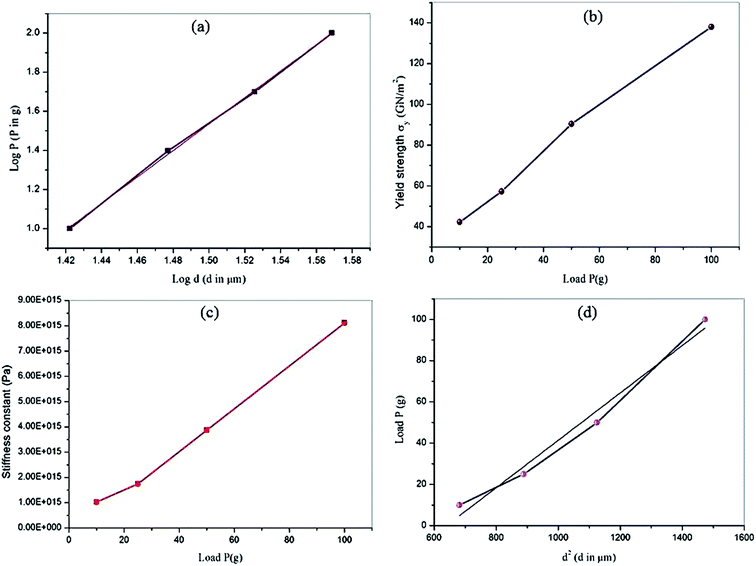 |
| Fig. 14 Meyer's plot (a), plot of yield strength vs. load (b), variation of stiffness constant with load P (c), and plot of load P vs. d2 (d). | |
A plot of load-dependent yield strength σy is shown in Fig. 14b. Also, the elastic stiffness constant was calculated using Wooster's empirical relation.36
The elastic stiffness constant (C11) gives an idea about strength and nature of bonding of atoms to their neighbors. Fig. 14c shows the plot between load and stiffness constant for the title compound. The calculated Vicker's hardness values, stiffness constant, and yield strength σy values of VSNS crystal for different loads from 10 to 100 g are given in Table 4. According to Hays and Kendall's approach,37 non-linear behavior of hardness material can be analytically explained using the expression P = W + A1d2, where W is the minimum load to initiate plastic (permanent) deformation in g, and A1 is the load-independent constant. The values of W and A1 were estimated from the plots drawn between the experimental data as P versus d2 shown in Fig. 14d. The estimated negative value of W reveals that the grown VSNS crystals exhibit behavior of reverse ISE.38 The corrected hardness Ho value (Table 5) of VSNS crystal was calculated using the relation:
Table 4 Microhardness, yield strength, and elastic stiffness constant values of VSNS
Load P (g) |
Hv (kg mm−2) |
σy (GN m−2) |
C11 × 1015 Pa |
10 |
38.51 |
42.23 |
1.0217 |
25 |
52.20 |
57.26 |
1.7403 |
50 |
82.42 |
90.40 |
3.8701 |
100 |
125.83 |
138.01 |
8.1140 |
Table 5 Results of constant W, A1, and Ho for VSNS
Hays–Kendall approach |
Results |
Resistance pressure (W) |
−9.15 (g) |
Load independent constant (A1) |
0.0480 (g µm−2) |
Corrected hardness (Ho) |
89 (g µm−2) |
Thus, the hardness study of VSNS crystal showed it has sufficient mechanical strength for various nonlinear device fabrications.
3.9. Chemical etching studies
Etching of a surface is a very simple and powerful characteristic to analyze the quality of the grown crystal, and also provides information on structural defects and crystal growth mechanism. The NLO applications of grown crystal primarily depend on high-quality single crystals because defect configuration can occur during crystal growth causing distortion of efficiency of the optical beam in laser material processing. Hence, it is necessary to analyze any microstructural imperfections present in the grown crystals.39 In the present investigation, etching studies of VSNS crystal were carried out using methanol as etchant at room temperature at different durations: 10 s, 20 s, and 40 s. After the crystal was etched with methanol, the etched surfaces were dried using tissue paper and their etched pattern was examined using a Carl Zeiss optical microscope at 50× magnification. Fig. 15a shows the before etching pattern of as-grown VSNS crystal, whereas after etching Fig. 15b shows rectangular well-defined etch pits for 20 s. There was no change in morphology of the etch pits with increase of etching time, suggesting that the grown crystal has good optical quality with less dislocations, as shown in Fig. 15c (40 s).
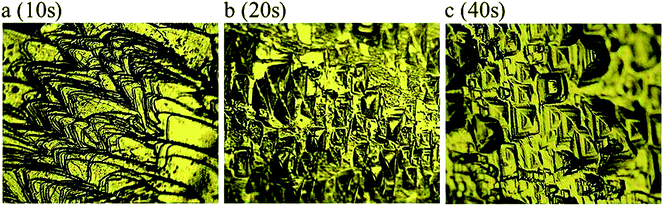 |
| Fig. 15 Chemical etching time of VSNS single crystal on (1 0 0) face with methanol solvent as an etchant (a) as grown crystal surface, (b) 20 s, and (c) 40 s. | |
3.10. Laser-induced damage threshold studies
The laser damage threshold of optical crystal is caused by various physical processes such as electron avalanche, multiphoton absorption, and photoionization for transparent materials, whereas in the case of high absorbing materials, the damage threshold is mainly caused by temperature rise, which leads to strain induced fracture.40,41 The utility of NLO crystal depends not only on the linear and nonlinear optical properties, but also largely on its surface quality providing the ability to withstand high power intensities.42 In the present study, laser damage threshold of grown VSNS crystal was measured using a Q-switched pulsed Nd:YAG (1064 nm) laser operating in transverse mode (TM00) and pulse width of 10 ns in the frequency rate of 10 Hz. A laser beam of diameter 1 mm was used to irradiate the crystal surface. The output of the incident laser beam was controlled with a variable attenuator and delivered to the crystal, which was placed at the focus of the converging lens of focal length 30 cm. The energy density of the input laser beam was measured using a power meter (model no. EPM 2000) at the point of damage to the crystal.
The energy density was calculated using the expression:
power density (Pd) = E/τπr |
where
E is the input energy density (mJ),
τ is the pulse width (ns), and
r is the area of the circular spot (mm). The calculated laser damage threshold value of VSNS was found to be 1.94 GW cm
−2, which is higher than that of KDP (0.20) and urea (1.50) crystals.
43 Hence, the grown crystal has a good surface because of its high laser damage threshold value. Thus, the title crystal could be useful for high power laser applications.
3.11. Dielectric studies
3.11.1. Dielectric constant and dielectric loss measurements. Dielectric measurements can provide information about electro-optical molecular responses, molecular anisotropy, structural changes, and transport phenomena within crystalline materials.44–46 The dielectric constant and loss of the grown crystal was carried out as a function of frequency varying from 50 Hz to 5 MHz at room temperature using a HIOKI 3532-50 LCR HiTESTER meter. The as-grown optical quality single crystals of 2 mm thickness with 1 0 0 face of VSNS were used for study of both dielectric constant and dielectric loss measurement. The sample surfaces were coated by silver paste to make uniform electrical contact with the surface of electrodes. The sample cell was placed in a thermal insulation container in which an electric heater was housed to ensure good thermal conditions of the cell. The dielectric constant (ε∞) of VSNS was calculated using the following equation:where C is the capacitance, t is the crystal thickness, εo is the vacuum dielectric constant, and A is the area of the crystal. Fig. 16 shows the variations of dielectric constant and log frequency. From Fig. 16, the values of dielectric constant are high in the lower frequency region, and, further, the dielectric constant decreases with increasing frequency. The higher value of dielectric constant at low frequencies (5 Hz) is a result of the contribution of all the four polarizations, namely, space charge, orientational, electronic, and ionic polarization. The low value at the higher frequency region may be caused by the inability of the dipoles to comply with the external field.47,48 Fig. 17 represents the variation of dielectric loss as a function of log frequency. From the graph, the values of dielectric loss decrease with increasing in frequency. Thus, the characteristic very low values of dielectric constant and dielectric loss at high frequencies for these crystals suggest enhanced optical quality with lesser defects. Thus, the crystal could be useful for optoelectronic applications and NLO devices.49
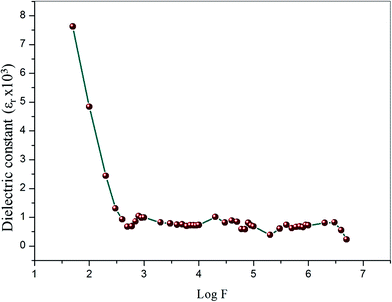 |
| Fig. 16 Variation of dielectric constant with log frequency at room temperatures for VSNS single crystal. | |
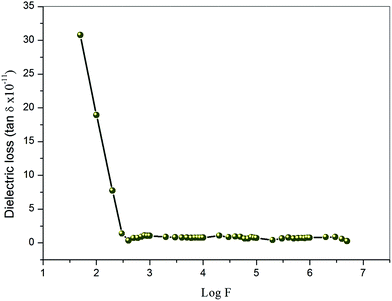 |
| Fig. 17 Variation of dielectric loss with log frequency at room temperatures for VSNS single crystal. | |
3.11.2. Solid state parameters of VSNS crystal based on single crystal XRD. The dielectric constant at high frequency is explicitly dependent on the valence electron of compound, plasma energy, Penn gap, and the Fermi energy. The Penn gap is calculated by fitting the dielectric constant with the plasmon energy.50 From the empirical formula, the formula weight of the grown crystal is 485.54 g mol−1, and the total number of the valence electron (Z) in the structural unit is Z = 180. The density of the title compound was found to be ρ 1.390 mg m−3. From the dielectric constant (ε∞), the dielectric constant at 1 MHz was calculated to be 716. From these data, the valence electron plasma energy (ħωp) can be calculated, as given by Jackson et al.51 |
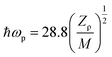 | (13) |
Explicitly, the ħωp dependent Penn gap (EP) and the Fermi energy (EF) in eV are derived from the following relations:49
|
 | (14) |
The electronic polarizability (α) of the title crystal can be calculated using the relation:52
|
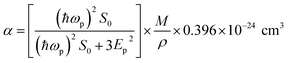 | (16) |
where
S0 is a constant for a particular material which is obtained using the relation
|
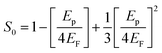 | (17) |
The value of electronic polarizability α was also confirmed using the Clausius–Mossotti relation, which agrees with the values obtained from eqn (16).
|
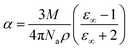 | (18) |
where
Na is the Avogadro number (
N = 6.023 × 10
23). The value of electronic polarizability
α can also be obtained using optical bandgap:
|
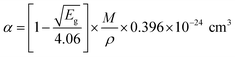 | (19) |
where
Eg is the band gap (eV) of the crystal, and all these calculated parameters for the title crystal are listed in
Table 6.
Table 6 Electric property for the grown VSNS crystal
Parameters |
Values |
Plasma energy (eV) |
20.67 |
Penn gap (eV) |
0.7731 |
Fermi energy (eV) |
16.72 |
Electronic polarizability (α) using Penn analysis |
13.77 × 10−23 cm3 |
Electronic polarizability (α) using Clausius–Mossotti equation |
13.79 × 10−23 cm3 |
Electronic polarizability (α) using optical band gap |
7.68 × 10−23 cm3 |
3.12. Z-scan measurements
The Z-scan technique is a highly sensitive and useful tool for determining the magnitude and sign of nonlinear index of refraction (n2) of solids, liquid solutions, and thin films. The nonlinear absorption coefficient (β) of materials was introduced by Sheik Bahae et al.17,18 It is a widely accepted technique for characterization of nonlinear optics by the scientific community. In this experiment, the third-order NLO properties of the title crystal were investigated using a He–Ne laser (5 mW) of wavelength at 632.8 nm as a source, with beam diameter 0.5 mm. The beam was focused with a Gaussian filter to convert the input laser beam into Gaussian form. The Gaussian beam TEM00 mode was passed through a convex lens with focal length 30 mm. This focal length completely depends on the incident Gaussian beam. The diameter of the Gaussian beam waist ω0 at the focal length was 12.05 µm. The sample was translated in the +Z to −Z axial direction by the control of the stepper motor to vary the incident intensity falling on the crystal surface. The far field transmittance intensity variations were measured through the closed aperture using a digital power meter (Field master GS-coherent). The recorded normalized transmittances in closed and open aperture curves for VSNS are given in Fig. 18 and 19. From the closed aperture Z-scan curve, the pre-focal valley to post-focal peak configuration of the aperture curve suggests that the third-order nonlinear refractive index change is positive. This indicated a self-focusing effect caused by reduced transmittance and large beam divergence through the far field aperture. For open aperture Z-scan data, the measurements were repeated as the sample was moved along the focal point and without placing the aperture to capture the entire transmitted beam by the detector. The essential criteria of sample thickness condition were satisfied for this measurement with the Rayleigh length (ZR = K > L, where L denotes its thickness, and ZR is the diffraction length of the Gaussian beam).18 The value of Rayleigh length of a Gaussian laser beam can be calculated using the relation: |
 | (20) |
where K is the wave vector (K = 2π/λ, 9.924 × 106 m−1) and ω0 is the beam waist radius at the focal point given by |
 | (21) |
where f is the focal length of the lens used, λ is the wavelength of the source, and D is the beam radius at the lens. The difference between the transmittance change of peak and valley (ΔTp–v) is evaluated by |
ΔTp–v = 0.406(1 − S)0.25|ΔΦ0|
| (22) |
where S is the aperture linear transmittance (S = 0.15), ra is the radius of the aperture, and ωa is the beam radius at the aperture. The linear transmittance and nonlinear refractive index (n2) of the crystal was calculated using the relations:53 |
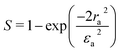 | (23) |
|
 | (24) |
where I0 is the intensity of the laser beam at the focal point (I0 = 26.50 MW m−2) and Leff is an effective thickness of the crystal which can be calculated using the relation Leff = [1 − exp(−αL)]/α. Here, α is the linear absorption, and L is the thickness of the crystal. From the open aperture curve, the nonlinear absorption coefficient (β) can be determined using the following relation:17 |
 | (25) |
where ΔT is the one valley value at the open aperture Z-scan curve. The value of β will be negative for saturated absorption and positive for two photon absorption processes.54 The calculated values of n2 and β were used to determine the real and imaginary parts of the third-order nonlinear optical susceptibility: |
 | (26) |
|
 | (27) |
where ε0 is the permittivity of free space (8.8518 × 10−12 F m−1), n0 is the linear refractive index of the crystal, and C is the velocity of light in vacuum. The absolute value of the third-order nonlinear optical susceptibility χ(3) is thus |
|χ(3)| = [(Re(χ(3)))2 + (Im(χ(3)))2]½
| (28) |
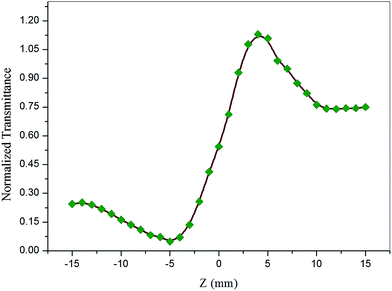 |
| Fig. 18 Self-focusing (closed aperture) Z-scan plot of VSNS crystal. | |
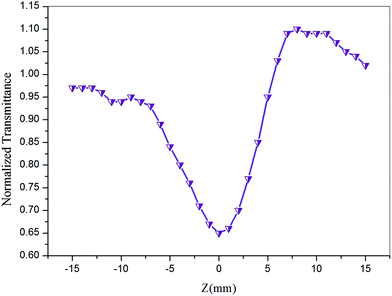 |
| Fig. 19 Open aperture mode Z-scan plot of VSNS crystal. | |
In addition, the second-order hyperpolarizability γ which defines the nonlinear induced polarization per molecule, is related to the third-order large susceptibility γ by:55
|
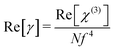 | (29) |
where
N is the number of molecules per unit volume and
f is the local field correction factor according to the Lorentz expression:
|
 | (30) |
The coupling factor ρ = Im((χ3)/Re(χ3)) is the ratio of the imaginary part (Im((χ3)) to the real part (Re(χ3)) of third-order nonlinear susceptibility. The calculated coupling factor ρ was found to be 57.57. This is because the contribution of nonlinear absorption change is more dominant than the nonlinear refraction and the electronic origin of nonlinearity.56 According to eqn (20)–(24), the third-order nonlinear refractive index (closed-aperture) is (n2, 5.214 m2 W−1) and the nonlinear absorption coefficient (open-aperture) (β, 5.954 m W−1). The absolute value of third-order nonlinear susceptibility (χ3, 6.564 × 10−5 esu) and the second-order molecular hyperpolarizability (γ, 7.986 × 10−34 esu) were calculated for VSNS crystal (Table 7). The larger value of second-order molecular hyperpolarizability γ was mainly because of the higher π-electron conjugation present in the VSNS crystal. The open aperture Z-scan curves exhibit a decrease in transmission with increase in the input intensity. This indicates the presence of reverse saturable absorption (RSA) (excited state absorption) with a positive nonlinear absorption coefficient, which leads to material for optical limiting applications.57,58 Reverse saturable absorption (RSA) means that the absorption of the molecule in the excited state is higher than in the ground state.59 Thus, the excellent nonlinear optical properties of the grown crystal make it a promising material for optical limiting applications.
Table 7 Obtained nonlinear optical parameter from open- and closed-aperture Z-scan measurement data for VSNS crystal
Laser beam wavelength (λ) |
632.8 nm |
Lens focal length (f) |
30 mm |
Optical bath length |
85 cm |
Beam radius of the aperture (ωa) |
3.5 mm |
Aperture radius (ra) |
1 mm |
Sample thickness (L) |
0.63 mm |
Effective thickness (Leff) |
2.040 × 10−3 m |
Linear absorption coefficient (α) |
−3207 |
Nonlinear refractive index (n2) |
5.214 × 10−12 m2 W−1 |
Nonlinear absorption coefficient (β) |
5.954 × 10−5 m W−1 |
Real part of the third-order susceptibility [Re(χ3)] |
1.140 × 10−6 esu |
Imaginary part of the third-order susceptibility [Im((χ3)] |
6.564 × 10−5 esu |
Third-order nonlinear optical susceptibility (χ3) |
6.564 × 10−5 esu |
Second-order molecular hyperpolarizability (γ) |
7.986 × 10−34 esu |
4. Conclusions
A new stilbazolium derivative crystal was synthesized, and the single crystals were grown by a slow evaporation method for the first time. The crystal structure was confirmed by single crystal X-ray diffraction analysis, showing the arrangement of molecules and formation of hydrogen bonds in the grown crystal. The morphology of the grown crystal revealed the prominent growth to be along the b-axis. The powder X-ray diffraction study confirms that the crystalline perfection is fairly good. The presence of functional groups and molecular structure of grown crystal were confirmed by FTIR and NMR spectral analysis. The UV-Vis-NIR spectra of the VSNS crystals showed that they are highly transparent in nature in the Vis-NIR region, with the cutoff wavelength found to be 390 nm. The calculated optical band-gap value was found to be 3.252 eV using Tauc's plot. Optical parameters such as the absorption coefficient (α), reflectance (R), refractive index (n0), and extinction coefficient (K) were calculated to analyze the optical properties. The transparency of the grown crystal was good, and is appropriate for optoelectronic and NLO applications. Further, the photoluminescence shows green emission radiation. TG–DTA studies indicated that the compound is thermally stable up to its melting point 196.6 °C. Dielectric measurement of VSNS revealed the low dielectric constant and loss at higher frequencies, and hence this crystal is more attractive for optoelectronic applications and NLO devices. The electronic polarizability (α) of the grown crystal was calculated by Penn analysis using dielectric constant, and this agreed with that of the Clausius–Mossotti relation. The Vicker's hardness studies indicated that the material is suitable for nonlinear applications. The results of etching studies revealed the growth mechanism of the title crystal layer pattern with minimum dislocations. The higher value of surface laser damage threshold of VSNS suggests that these crystals may have favorable application in laser frequency conversion. The single beam Z-scan experimental results confirmed the large nonlinear absorption coefficient and positive value of third-order nonlinear refractive index, which are required for optical limiting applications. From the present investigation, we conclude that VSNS is a new candidate for NLO device applications.
Acknowledgements
The authors are thankful to the Defence Research and Development Organisation (DRDO), Government of India, for financial assistance (Project Ref. no. ERIP/ER/1103929M/01/1402). The authors are grateful to VIT University management for their constant support and for providing research facilities.
References
- C. Zhang, Y. L. Song and X. Wang, Coord. Chem. Rev., 2007, 251, 111 CrossRef CAS PubMed.
- P. N. Prasad and D. J. Williams, Introduction to Nonlinear Optical Effect in Molecules and Polymers, John Wiley & Sons, New York, 1991, pp. 2–10 Search PubMed.
- M. Spasenovi, M. Betz, L. Costa and H. M. Van Driel, Phys. Rev. B: Condens. Matter Mater. Phys., 2008, 77, 085201 CrossRef.
- D. S. Chemla and J. Zyss, Nonlinear Optical Properties of Organic Molecules and Crystals, Academic Press, London, 1987, vol. 1 Search PubMed.
- J. Zyss, Molecular Nonlinear Optics: Materials Physics and Devices, Academic Press, New York, Physics and Devices, 1993 Search PubMed.
- J. Badan, R. Hierle, A. Perigand and J. Zyss, in Nonlinear Optical Properties of Organic Molecules and Polymeric Materials, 233 D. 5, ed. D. J. Williams, American Chemical Society, Washington, DC, 1993 Search PubMed.
- W. Denk, J. H. Strickler and W. W. Webb, Science, 1990, 248, 73–76 CAS.
- C. C. Corredor, Z. L. Huang, K. D. Belfield, A. R. Morales and M. V. Bondar, Chem. Mater., 2007, 19, 5165–5173 CrossRef CAS.
- C. Q. Tang, Q. Zheng, H. M. Zhu, L. X. Wang, S. C. Chen, E. Ma and X. Y. Chen, J. Mater. Chem. C, 2013, 1, 1771–1780 RSC.
- M. Samoc, N. Gauthier, M. P. Cifuentes, F. Paul, C. Lapinte and M. G. Humphrey, Angew. Chem., Int. Ed., 2006, 45, 7376 CrossRef CAS PubMed.
- W. Guan, G. C. Yang, G. C. Liu, P. Song, L. Fang, L. K. Yan and Z. M. Su, Inorg. Chem., 2008, 47, 5245 CrossRef CAS PubMed.
- Y. Takahashi, H. Adachi, T. Taniuchi, M. Takagi, Y. Hosokawa, S. Onzuka, S. Brahadeeswaran, M. Yoshimura, Y. Mori, H. Masuhara, T. Sasaki and H. J. Nakanishi, J. Photochem. Photobiol., A, 2006, 183, 247 CrossRef CAS PubMed.
- B. J. Coe, J. A. Harris, I. Asselberghs, K. Wostyn, K. Clays, A. Persoons, B. S. Brunschwig, S. J. Coles, T. Gelbrich, M. E. Light, M. B. Hursthouse and K. Nakatani, Adv. Funct. Mater., 2003, 13, 347 CrossRef CAS.
- G. R. Meredith, in Nonlinear Optical Properties of Organic and Polymeric Materials, ed. D. J. Williams, ACS Symposium Series, 233, American Chemical Society, Washington, DC, 1983, pp. 27–56 Search PubMed.
- G. Seetharam Shettigar, K. Umesh, K. Chandrasekharan and Balakrishna Kalluraya, Synth. Met., 2007, 57, 142–146 CrossRef PubMed.
- J. H. Klein-Wiele, M. A. Bader, I. Bauer, S. Soria, P. Simon and G. Marowsky, Synth. Met., 2002, 127, 53–57 CrossRef CAS.
- M. Sheik-Bahae, A. A. Said and E. W. Van Stryland, Opt. Lett., 1989, 14, 955–957 CrossRef CAS.
- M. Sheik-Bahae, A. A. Said, T. H. Wei, D. J. Hagan and E. W. Van Stryland, IEEE J. Quantum Electron., 1990, 760–769 CrossRef CAS.
- M. G. Kuzyk and C. W. Dirk, Characterization techniques and tabulations for organic nonlinear optical materials, Marcel Dekker, Inc., 1998 Search PubMed.
- W. S. Wang, K. Sutter, C. Bosshard, Z. Pan, H. Arend, P. Gunter, G. Chapuis and F. Nicolo, Jpn. J. Appl. Phys., 1988, 27, 1138–1141 CrossRef CAS.
- W. J. Kaminsky, Appl. Crystallogr., 2007, 40, 382 CrossRef CAS.
- G. M. Sheldrick, Acta Crystallogr., Sect. A: Found. Crystallogr., 2008, 64, 112–122 CrossRef CAS PubMed.
- G. Socrates, Infrared and Raman of Characteristic Frequencies, John Wiley and Sons Ltd., Chichester, 3rd edn, 2001 Search PubMed.
- V. Balachandran and K. Parimala, Spectrochim. Acta, Part A, 2012, 95, 354–368 CrossRef CAS PubMed.
- P. N. Prasad, Ser. Wave-Phenom., Springer, 1989, vol. 9, pp. 305–327 Search PubMed.
- D. D. O. Eya, A. J. Ekpunobi and C. E. Okeke, Acad. Open Internet J., 2006, 17 Search PubMed.
- S. Redrothu Hanumantharao, S. Kalainathan, G. Bhagavannarayana and U. Madhusoodanan, Spectrochim. Acta, Part A, 2013, 103, 388–399 CrossRef PubMed.
- S. Sudhahar, M. Krishna Kumar, B. N. Sornamurthy and R. Mohan Kumar, Spectrochim. Acta, Part A, 2014, 118, 929–937 CrossRef CAS PubMed.
- B. R. Lawn and E. R. Fuller, J. Mater. Sci., 1975, 10, 2016–2024 CrossRef CAS.
- J. H. Westbrook, Flow in rock salt structure, Report 58-R L, 2033 of GE Research Laboratory, USA, 1958 Search PubMed.
- C. C. Desai and J. L. Rai, Bull. Mater. Sci., 1983, 5, 453 CrossRef CAS.
- K. K. Rao and D. B. Sirdeshmukh, Bull. Mater. Sci., 1983, 5, 449–452 CrossRef CAS.
- K. Sangwal, Mater. Chem. Phys., 2000, 63, 145 CrossRef CAS.
- R. Vijayan, R. Ramesh Babu, R. Gopalakrishnan, P. Ramasamy and W. T. A. Harrison, J. Cryst. Growth, 2004, 262, 490–498 CrossRef PubMed.
- N. V. Prasad, G. Prasad, T. Bhimasankaran, S. V. Suryanarayan and G. S. Kumar, Indian J. Pure Appl. Phys., 1996, 34, 639 Search PubMed.
- W. A. Wooster, Rep. Prog. Phys., 1953, 16, 62–82 Search PubMed.
- C. Hays and E. G. Kendall, Metallography, 1973, 6, 275 CrossRef CAS.
- A. A. El-Fadl, A. S. Soltan and N. M. Shaalan, Cryst. Res. Technol., 2007, 42, 364–377 CrossRef.
- P. Pandi, G. Peramaiyan, M. Krishna Kumar, R. Mohan Kumar and R. Jayavel, Spectrochim. Acta, Part A, 2012, 88, 77–81 CrossRef CAS PubMed.
- W. L. Smith, Opt. Eng., 1959, 17, 489 Search PubMed.
- B. C. Stuart, M. D. Feit, A. M. Rubenchik, B. M. Shore and M. D. Perry, Phys. Rev. Lett., 1995, 74, 2248 CrossRef CAS.
- G. C. Bhar, A. K. Chaudhary and P. Kumbhakar, Appl. Surf. Sci., 2000, 161, 155–162 CrossRef CAS.
- N. Vijayan, G. Bhagavannarayana, K. R. Ramesh, R. Gopalakrisnan, K. K. Maurya and P. Ramasamy, Cryst. Growth Des., 2006, 6, 1542 CAS.
- H. M. Lin, Y. F. Chen, J. L. Shen and W. C. Chou, J. Appl. Crystallogr., 2001, 89, 4476 CAS.
- J. Philip and T. A. Prasada Rao, Phys. Rev. A, 1992, 46, 2163–2165 CrossRef CAS.
- H. J. Coles and S. V. Kershaw, J. Chem. Soc., Perkin Trans. 2, 1988, 987–996 CAS.
- B. B. Parekh and M. J. Joshi, Cryst. Res. Technol., 2007, 42(2), 127–132 CrossRef CAS.
- S. A. Mazen, F. Metawe and S. F. Mansour, J. Phys. D: Appl. Phys., 1997, 30, 1799–1808 CrossRef CAS.
- C. Balarew and R. Duhlew, J. Solid State Chem., 1984, 551, 1–6 CrossRef.
- N. M. Ravindra, R. P. Bharadwaj, K. Sunil Kumar and V. K. Srivastava, Infrared Phys., 1981, 21, 369 CrossRef CAS.
- J. D. Jackson, Classical Electrodynamics, Wiley Eastern, 1978, pp. 321 Search PubMed.
- N. M. Ravindra and V. K. Srivastava, Infrared Phys., 1980, 20, 67–69 CrossRef CAS.
- E. M. Van Stryland, M. Sheik-Bahae, M. G. Kuzyk and C. W. Dirk, Characterization Techniques and Tabulations for Organic Nonlinear Materials, Marcel Dekker Inc., 1998, pp. 655–692 Search PubMed.
- T. C. Sabari Girisun, S. Dhanuskodi, D. Mangalaraj and J. Phillip, Curr. Appl. Phys., 2011, 11, 838–843 CrossRef PubMed.
- M. T. Zhao, B. P. Singh and P. N. Prasad, J. Chem. Phys., 1998, 89, 5535 CrossRef PubMed.
- K. Naseema, M. Shyma, K. B. Manjunatha, A. Muralidharan, G. Umesh and V. Rao, Opt. Laser Technol., 2011, 43, 1286–1291 CrossRef CAS PubMed.
- J. Wang and W. J. Blau, J. Opt. A: Pure Appl. Opt., 2009, 11, 024001 CrossRef.
- F. Z. Henari, W. J. Balua, L. R. Milgrom, G. Yahyoglu, D. Philips and J. A. Lacey, Chem. Phys. Lett., 1997, 267, 229 CrossRef CAS.
- F. Z. Henari, J. Opt. A: Pure Appl. Opt., 2001, 3, 188 CrossRef CAS.
Footnote |
† CCDC 1013563. For crystallographic data in CIF or other electronic format see DOI: 10.1039/c4ra09112d |
|
This journal is © The Royal Society of Chemistry 2014 |