DOI:
10.1039/C4RA09040C
(Paper)
RSC Adv., 2014,
4, 52366-52371
Effect of edge modification on transport properties of finite-sized, graphene nanoribbon-based molecular devices
Received
21st August 2014
, Accepted 30th September 2014
First published on 3rd October 2014
Abstract
The transport mechanisms of several finite-sized, graphene nanoribbon-based junctions have been computationally investigated using density functional theory and Green's functional method. Acceptor-type and donor-type functional groups were introduced, serving as electrode connection and edge modification, respectively. The introduction of acceptor and donor groups improve the electron transmission probabilities by several orders of magnitude. The electronic coupling mode, carrier concentration and distribution, and molecular orbital can be manipulated by the modification groups.
Introduction
In recent years, carbon-based materials, such as graphene and carbon nanotubes, have been widely studied both theoretically and experimentally due to their remarkable structural, electrical and chemical properties.1–6 Graphene has been a hot topic and an important candidate in the area of electronic applications because of its unique electronic transport properties.7–10 Numerous research works on two-dimensional graphene, quasi-one dimensional graphene nanoribbons (GNR) and zero-dimensional GNR slices have been reported.11–17 With the development of experimental methods, graphene can be cut to different configurations to achieve different functional requirements.18,19 Thus, dangling bonds appear at the edge carbon atoms of finite-sized GNRs. The passivation of the opening edge provides an effective way for GNR slice-based materials to be functionally modified.20 Many methods can be used to saturate the dangling bonds.21–25 When used as edge-functional modifications, the modification atoms or groups can tune the electronic distribution and concentration, and the hybridization and the symmetry of the systems,26 thus manipulating the molecular orbital. When used as electrode connections, the edge modification groups can tune the coupling method and the contact conductance of the junctions.27 Therefore, edge modification is important to the transport properties of finite-sized, GNR-based molecular devices.
In this article, several special edge modification group pairs were considered in order to study the transport mechanisms of the finite-sized, GNR-based junctions. Many valuable research studies on modified GNR-based molecular devices have been reported. However, introducing donor or acceptor groups to achieve edge passivation and electrode connection of the system at the same time is an effective and novel method of material modification. The carrier concentration and distribution have also been tuned. Furthermore, with the size becoming smaller, quantum effects become the main mechanism in the electronic transport process. Therefore, further investigations are required on the effect of edge modification on the conducting ability of finite-sized, GNR-based molecular devices.
Theory and calculation methods
The theoretical study of GNR-based, nano-scale quantum devices was researched at the level of first principles. Geometry optimization and analysis of the electronic properties are performed by using the density-functional theory for the B3LYP level28,29 with mixed-basis sets (the LanL2DZ basis set for electrode Au atoms, and 6-31G basis set for other atoms). Electron transport in the junctions can be described by electron scattering theory. Considering the simplest system of two electron reservoirs connected by one of the edge-modified GNR slices, under the external bias, electrons in the source electrode will be driven to the drain electrodes. The whole process is dominated by electrons scattering through different scattering channels, which is the molecular orbital in the central molecule core systems. A generalized, all-electrons first principle approach based on scattering theory and Green's functional theory was used to study the electron transport properties in GNR-based molecular electronic devices.
The electronic transport properties are calculated by using the quantum chemistry for molecular electronics (QCME) code.30–32 The tunnelling current density from the source to drain can be obtained with the following formula,
|
 | (1) |
where
nS(
E) and
nD(
E) are the density of states of the source and drain at the energy level
E, respectively.
f(
E) is the Fermi distribution function

.
Tl′l is the transmission probability describing the scattering process from the initial state |l〉 to the final state |l′〉, which can be expressed as
|
 | (2) |
where
VAB is the coupling energy between the layer sites A and B, and
gK′Kη represents the carrier-conduction contribution from the scattering channel
εη.
Results and discussion
Models
As is well known, there are two types of edge termination for finite-sized GNRs, named zigzag (ZZ) and armchair (AC), respectively. In this article, we choose a 4 × 4 (4 carbon atoms on the zigzag edges and 4 carbon atoms on the armchair edges) prototype graphene nanoribbon slice with both zigzag and armchair edges as the basic molecular core. Here, all of the carbon atoms on both sides of the AC edges have been passivated by hydrogen atoms, and the carbon atoms on both sides of the ZZ edges have been modified by functional group pairs. As mentioned in ref. 25, the introduction of donor–acceptor group pairs on the ZZ edges of GNRs can achieve particular electronic properties. Furthermore, NO2–NO2 or NH2–NH2 functional group pairs, which can change the concentration of carriers while keeping the symmetry of the system, are also interesting models. The donor–acceptor groups can achieve edge passivation and electrode connection of the system at the same time. Thus, four modification types of edge-functional group pairs: hydrogen–hydrogen (H–H), donor-type modified pair NH2–NH2 (D–D), acceptor-type modified pair NO2–NO2 (A–A) and donor–acceptor groups-modified pair NH2–NO2 (A–D) for the dangling bonds of the ZZ edge carbon atoms have been considered. The molecular device models are composed of edge-modified GNRs sandwiched between Au(111) metallic electrodes. In this work, for simplicity, we use a triangle gold cluster connected with the edge-terminated groups of the molecular core (H, NH2, NO2) as the contact geometry in all model systems. The schematic diagrams of the edge-modified, GNR-based molecular devices with different edge passivation are shown in Fig. 1. For simplicity, we define the junction system as follows when Au electrodes connect to the AC edges – that is, we consider the transport properties along the crystallographic orientations of ZZ edges. The systems are defined as H–H ZZ, A–A ZZ, D–D ZZ or A–D ZZ. The H–H AC, A–A AC, D–D AC or A–D AC, for the junctions with Au electrodes, connect to the ZZ edges. For example, the notation A–A ZZ indicates a junction constructed by connecting the AC edges of the GNR slice (in which the ZZ edge carbon atoms are modified by NO2–NO2 functional pairs) with Au electrodes. There are two main effects of the modified group pairs: one is that they serve as edge-functional modification groups, and another is as the connection between the molecular core and Au electrodes. The electron transport properties are performed, respectively, along the two directions of the ZZ and AC edges of the junctions.
 |
| Fig. 1 The schematic diagrams of the edge-modified, GNR-based molecular devices with different edge passivation. X and Y stand for the edge passivation type. | |
The effect of edge modification on the transport properties of 4 × 4 GNR-based junctions
It is known that the conductance of molecular devices can be divided into two parts. One depends on the molecule–electrode interface, and the other depends largely on the molecular core. For the X–X ZZ model systems (X stands for the decoration type of the ZZ edge carbon atoms, X = H, A, D), the carbon atoms on AC edges have been passivated by hydrogen atoms in all the junctions, and the functional pairs (H–H, A–A, D–D or A–D) on the ZZ edge carbon atoms serve as the edge-modification pairs. That is, four junctions have been considered with the same connection type and different edge modification types. Edge modification pairs can change the carrier concentration of the system. The carrier distribution in the frontal molecular orbitals (MOs) of the GNR slices can be changed, and thus the electronic and transport properties of the GNR slice-based devices can be tuned. So in this part, we focus on the effect of edge modification on the transport properties. The calculated density of states (DOS), quantum conductance (G/G0, where G0 = 2e2/h is the conductance quantum unit and bias voltage = 2 V) and electron transmission probabilities (T) are shown in Fig. 2.
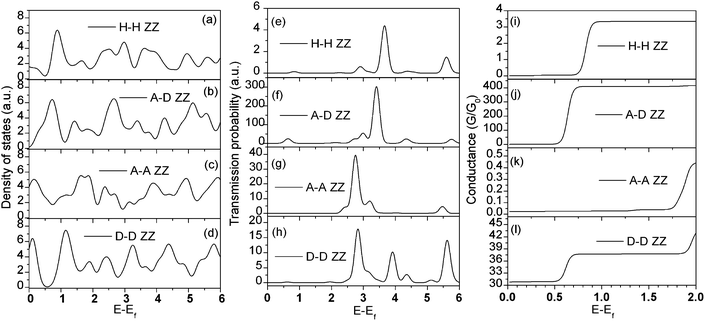 |
| Fig. 2 The density of states (DOS), transmission probability and quantum conductance (plot as G/G0 with G0 = 2e2/h being the conductance quantum, bias voltage = 2 V) curves of H–H ZZ, A–D ZZ, A–A ZZ and D–D ZZ 4 × 4 edge-modified, GNR-based junctions. | |
From Fig. 2(a)–(d), we can observe that with the introduction of the donor–acceptor groups, the DOS near the Fermi energy increases by about two or three times relative to that of the H–H ZZ system. Meanwhile, the DOS for the A–D ZZ system near the Fermi energy decreases because donor–acceptor pair groups inject the electron and hole simultaneously, providing the equilibrium distribution of carriers. The electrons of the four systems are delocalized, and thus can contribute to the improvement of electron transport probabilities. From Fig. 2(e)–(h), one can see that the transmission probabilities of the A–A ZZ, D–D ZZ and A–D ZZ systems are much higher than that of the H–H ZZ system. There are several obvious peaks of the transmission probabilities in some energy regions. New peaks indicate that the MOs at this energy region can be used as the conducting channel. Compared with the pure hydrogen-passivated system, the first strong peak of transmission probabilities is shifted to the lower energy region, which indicates that the junction can achieve resonant tunnelling (showing large conductance or current) at lower bias voltage. The A–D pair causes new peaks of transmission probability at some energy regions, providing more conducting channels. Therefore, the transmission of the A–D group modified systems is much stronger than that of the H–H system. The transport properties of the nanomolecular devices depend on the transmission probability and the DOS. Due to the finite size effect, the curves in Fig. 2(i)–(l) show that obvious quantum conductance is obtained in the four systems. The conductance stairs occur at the positions corresponding to peaks of the DOS and T. The coincidence between the peak position of the DOS, T and the conductance stairs indicates a resonant tunnelling process in the junctions. Comparing the four modified mechanisms, resonant tunnelling process is found in all the junctions, while there are obvious differences in the resonance level of each system. By introducing donor–acceptor groups, the conductance of D–D ZZ and A–D ZZ systems increases by an order of magnitude under lower bias voltage. As shown in Fig. 2(k), the conductance of the A–A ZZ system is smaller under low bias voltage. There are large increases until the bias voltage increases to about 2.4 V, which coincide with the results in Fig. 2(g) and 4(a). The Fermi energy of the electrode can be tuned by source and drain voltage. With increasing bias voltage, more molecular orbitals can be opened to serve as electron transport channels. Thus, the conductance can be increased under higher bias voltage.
 |
| Fig. 3 The density of states (DOS), transmission probability and quantum conductance (plot as G/G0 and bias voltage = 2 V) curves of H–H AC, A–D AC, A–A AC and D–D AC 4 × 4 edge-modified, GNR-based molecular devices. | |
 |
| Fig. 4 The current–voltage, quantum conductance (plot as G/G0 and bias voltage = 6 V), DOS and transmission probability curves for (a), (b) A–A ZZ, and (c), (d) A–A AC 4 × 4 edge-modified, GNR-based junctions (the solid and dashed lines correspond to the left and right axes, respectively). | |
The results of the isodensity analysis of the molecular orbits show that when the edge carbon atom is passivated by hydrogen atoms, sp2-like edge bonds are found. Hydrogen atoms do not change the sp2 hybridization of the systems. However, when the edge carbon atoms are modified with NH2 or NO2 groups, the degree of freedom in the vertical plane may cause sp2–sp3 re-hybridization at the edge bonds. On the one hand, the donor–acceptor functional groups can introduce sp2–sp3 re-hybridization; on the other hand, they change the carrier concentration and distribution of the GNR slices. In particular, for the A–D pair system, the NO2–NH2 pair groups break the inversion symmetry of the slices and also keep the equilibrium distribution of carriers. Furthermore, in the finite-sized GNR slices, the electronic properties such as the molecular orbital and the electron distributions can be tuned by the finite size effect. These mechanisms combined to tune the MOs of the GNR slices, thus changing the transport properties of the junctions. It is worth mentioning that for the junction models considered in this part, Au electrodes are connected with the molecular core through hydrogen atoms. This actually has a large contact resistance. Therefore, if one chooses other atoms with small contact resistance (such as sulphur atom), the conductance should increase significantly.
The effect of connection type on the transport properties of 4 × 4 GNR-based junctions
In this part, based on the X–X AC model systems, we focus on the effect of electrode connection type on the transport properties. For the X–X AC systems, the functional pairs (H–H, A–A, D–D or A–D) on the ZZ edge serve as electrode connection groups; the hydrogen atoms on AC edges serve as edge passivation. That is, four junctions have been considered with the same edge modification type and different connection types. The acceptor–donor functional pairs can tune the coupling mode between the molecular core and Au electrodes, and thus control the connection conductance of the GNR slice-based molecular devices. The calculated density of states (DOS), quantum conductance (plot as G/G0 and bias voltage = 2 V) and electron transmission probabilities (T) are shown in Fig. 3.
Compared with the H–H pair, new DOS peaks appear near the Fermi level because of the introduction of acceptor–donor functional groups. This is clearly shown in Fig. 3(a)–(d). These new peaks indicate that the electronic coupling between Au electrodes and molecular core is much stronger when using the functional group NH2 or NO2 as connection. Thus, the contact conductance is greatly enhanced. From the transmission probability curves shown in Fig. 3(e)–(h), one can see that the T of A–A AC, D–D AC and A–D AC systems is about one order of magnitude higher than that of the H–H AC system. From the G–V curves in Fig. 3(i)–(l), one can see that all four junctions exhibit significant quantum conductance and resonant tunnelling effect. The conductance stairs also occur at the peak positions of the DOS and T curves. The first obvious stair of quantum conductance of the A–A AC, D–D AC and A–D AC systems is a shift to lower energy region, which indicates that the electrons can be excited and achieve resonant tunnelling at lower bias voltage. The overall conductance has been improved especially at lower energy regions. Comparing with the ZZ systems (NH2 and NO2 serve as edge-functional modification groups), the effect of NH2 and NO2 groups on the T are mainly at low energy regions near the Fermi level, when they are used as electrode connection.
The comparison of transport properties for ZZ and AC systems
In order to compare the transport properties of the AC and ZZ systems, we choose two junctions as examples: A–A ZZ and A–A AC. In these two junctions, NO2 acceptor-type functional groups have been considered as the edge modification and connection types, respectively. It is known that the transport properties can also be tuned by the source–drain bias voltage, so we further studied the conductance and current under 6 V bias. The current–voltage, quantum conductance (plot as G/G0 and bias voltage = 6 V), DOS and transmission probability curves of A–A ZZ and A–A AC 4 × 4 edge-modified, GNR-based junctions (the solid and dashed lines correspond to the left and right axes, respectively) are shown in Fig. 4.
Compared with that of 2 V bias, in the 6 V case new peaks of the transmission probabilities appear at the higher energy region, and the quantum conductance is obviously increased. The Fermi energy of the electrode can also be tuned by source and drain voltage. More electrons can be excited, and more molecular orbitals can be opened with the increase of bias voltage. Thus, there are more channels for electron transport, and the conductance is increased. From Fig. 4(a)–(d), the quantum conductance and nonlinear current characteristic are clearly shown. The coincidence of the conductance stairs, inflection point of current and the peak position of DOS and T confirm the resonant tunnelling mechanism discussed above. The conductance of the two systems shows significant differences at the low-energy region near the Fermi level: the A–A ZZ system shows a large gap, while the A–A AC system has a detectable conductance. For the ZZ system, the connection type between Au electrodes and hydrogen atoms cause a large energy barrier. For the AC system, there is high electron coupling between Au electrodes and NO2 groups. Furthermore, the edge carbon atom arrangement of the GNR slices may partially influence electron coupling and the potential barrier. The overlap of electron clouds between carbon atoms on the zigzag edge is larger than that of the armchair edge. Therefore, ZZ systems, which have larger contact resistance, need more energy to run over the potential barrier. As further evidence, the isodensity of molecular orbits has also been studied. The results further indicate that the overlap of electron clouds between Au electrodes and NO2 groups is larger than that of H–H systems. The overall electron isodensity of the donor–acceptor modified systems is more delocalized. In short, the transport properties of the junction are sensitive to both functional groups serving as edge-modification and electrode connections. By effectively combining the two methods, the quantum conductance can be improved significantly.
Conclusions
In this paper, 4 × 4 finite-sized graphene nanoribbon slices are chosen as the prototype structure. In order to understand how the acceptor–donor (NO2–NH2) functional groups affect the electronic structure and transport properties of finite-sized GNR slices, several edge-functional modification types have been considered. We focus on two series of GNR-based molecular devices: one for the functional groups that serve as edge modification, and the other for the functional groups serving as electrode connection. The contact conductance and overall conductance are sensitive to the connection and modification types. Compared with the pure hydrogen systems, the quantum conductance and electron transmission probabilities are improved by orders of magnitude by the introduction of acceptor–donor groups. In practical applications, if one effectively combines the two methods by choosing atoms or groups with small contact resistance and better functional edge modification, the quantum conductance should increase significantly.
Acknowledgements
This work was supported in part by the National Natural Science Foundation of China (11304001, 51272001, 51472003 and 11174002), the National Key Basic Research Program (2013CB632705), the Ph.D. Programs Foundation for the Youth Scholars of Ministry of Education of China (20133401120002), the Foundation of State Key Laboratory for Modification of Chemical Fibers and Polymer Materials Donghua University (LK1217), Key project of the Foundation of Anhui Educational Committee (KJ2013A035) and the Ph.D. Programs Foundation of Anhui University (33190134). Computational resources from the Shanghai Supercomputer Centre are acknowledged.
Notes and references
- S. Banerjee, M. Sardar, N. Gayathri, A. K. Tyagi and B. Raj, Phys. Rev. B: Condens. Matter Mater. Phys., 2005, 72, 075418 CrossRef.
- X. L. Li, X. R. Wang, L. Zhang, S. Lee and H. J. Dai, Science, 2008, 319, 1229–1232 CrossRef CAS PubMed.
- N. M. R. Peres, J. Phys.: Condens. Matter, 2009, 21, 323201 CrossRef CAS PubMed.
- A. H. Castro Neto, F. Guinea, N. M. R. Peres, K. S. Novoselov and A. K. Geim, Rev. Mod. Phys., 2009, 81, 109–162 CrossRef CAS.
- B. Kevin and M. Raghunath, Appl. Phys. Lett., 2010, 96, 063104 CrossRef PubMed.
- D. Zhou, Q. Y. Cheng, Y. Cui, T. Wang, X. X. Li and B. H. Han, Carbon, 2014, 66, 592–598 CrossRef CAS PubMed.
- Q. M. Yan, B. Huang, J. Yu, F. W. Zheng, J. Zang, J. Wu, B. L. Gu, F. Liu and W. H. Duan, Nano Lett., 2007, 7(6), 1469–1473 CrossRef CAS PubMed.
- J. M. C. Rauba, M. Strange and K. S. Thygesen, Phys. Rev. B: Condens. Matter Mater. Phys., 2008, 78, 165116 CrossRef.
- Z. Y. Li, H. Y. Qian, J. Wu, B. L. Gu and W. H. Duan, Phys. Rev. Lett., 2008, 100, 206802 CrossRef.
- W. Y. Kim, Y. C. Choi, S. K. Min, Y. Cho and K. S. Kim, Chem. Soc. Rev., 2009, 38, 2319–2333 RSC.
- G. M. Rutter, N. P. Guisinger, J. N. Crain, E. A. A. Jarvis, M. D. Stiles, T. Li, P. N. First and J. A. Stroscio, Phys. Rev. B: Condens. Matter Mater. Phys., 2008, 76, 235416 CrossRef.
- H. Lee, J. Ihm, M. L. Cohen and S. G. Louie, Nano Lett., 2010, 10, 793–798 CrossRef CAS PubMed.
- F. T. Wang, L. Chen, Ch. J. Tian, Y. Meng, Zh. G. Wang, R. Q. Zhang, M. X. Jin, P. Zhang and D. J. Ding, J. Comput. Chem., 2011, 32(15), 3264–3268 CrossRef CAS PubMed.
- B. Albert, R. W. Michael and B. Z. Per, J. Polym. Sci., Part A: Polym. Chem., 2012, 50(15), 2981–2992 CrossRef.
- L. Zhang, L. Zhou, M. Yang, Z. Liu, Q. Xie, H. Peng and Z. Liu, Small, 2013, 9(8), 1134–1143 CrossRef CAS PubMed.
- H. Liu, H. Wang, J. Zhao and M. Kiguchi, J. Comput. Chem., 2013, 34(5), 360–365 CrossRef CAS PubMed.
- X. K. Kong, C. L. Chen and Q. W. Chen, Chem. Soc. Rev., 2014, 43, 2841–2857 RSC.
- K. S. Kim, Y. Zhao, H. Jang, S. Y. Lee, J. M. Kim, K. S. Kim, J. H. Ahn, P. Kim, J. Y. Choi and B. H. Hong, Nature, 2009, 457, 706–710 CrossRef CAS PubMed.
- S. Bae, H. Kim, Y. Lee, X. Xu, J. S. Park, Y. Zheng, J. Balakrishnan, T. Lei, H. R. Kim, Y. Song, Y. J. Kim, K. S. Kim, B. Ozyilmaz, J. H. Ahn, B. H. Hong and S. Lijima, Nat. Nanotechnol., 2010, 574, 1–5 Search PubMed.
- G. Daniel, J. W. Li, W. M. John and T. W. Carter, Nano Lett., 2010, 10, 3638–3642 CrossRef PubMed.
- K. Yousuke, K. Fukui and T. Enoki, Phys. Rev. B: Condens. Matter Mater. Phys., 2006, 73, 125415 CrossRef.
- H. X. Zheng and W. Duley, Phys. Rev. B: Condens. Matter Mater. Phys., 2008, 78, 045421 CrossRef.
- L. Rosales, M. Pacheco, Z. Barticevic, A. Latgé and P. A. Orellana, Nanotechnology, 2008, 19, 065402 CrossRef CAS PubMed.
- B. Xu, J. Yin, Y. D. Xia, X. G. Wan, K. Jiang and Z. G. Liu, Appl. Phys. Lett., 2010, 96, 163102 CrossRef PubMed.
- E. J. Kan, Z. Y. Li, J. L. Yang and J. G. Hou, J. Am. Chem. Soc., 2008, 130, 4224–4225 CrossRef CAS PubMed.
- W. Y. Kim and K. S. Kim, Acc. Chem. Res., 2010, 43, 111–120 CrossRef CAS PubMed.
- E. J. H. Lee, K. Balasubramanian, R. T. Weitz, M. Burghard and K. Kern, Nat. Nanotechnol., 2008, 3, 486–490 CrossRef CAS PubMed.
- A. D. Becke, J. Chem. Phys., 1993, 98, 5648–5652 CrossRef CAS PubMed.
- C. Lee, W. Yang and R. G. Parr, Phys. Rev. B: Condens. Matter Mater. Phys., 1988, 37, 785–789 CrossRef CAS.
- J. Jiang, C. K. Wang and L. Yi, QCME-V1.1 (Quantum Chemistry for Molecular Electronics) Search PubMed.
- C. K. Wang and Y. Luo, J. Chem. Phys., 2003, 119, 4923–4928 CrossRef CAS PubMed.
- J. Jiang, M. Kula and Y. Luo, J. Chem. Phys., 2006, 124, 034708 CrossRef PubMed.
|
This journal is © The Royal Society of Chemistry 2014 |
Click here to see how this site uses Cookies. View our privacy policy here.