DOI:
10.1039/C4RA08333D
(Paper)
RSC Adv., 2014,
4, 50832-50839
Spherical V-MCM-48: the synthesis, characterization and catalytic performance in styrene oxidation
Received
8th August 2014
, Accepted 16th September 2014
First published on 17th September 2014
Abstract
A series of spherical, vanadium-containing, mesoporous V-MCM-48 catalysts with different V/Si atomic ratios were synthesized by a direct hydrothermal method. The structure and morphology of the samples and the states of vanadium in the materials were systematically characterized by XRD, N2 physisorption, FE-SEM, HRTEM, ICP, UV-vis, 51V MAS-NMR, ESR and XPS. The possible formation mechanism of spherical V-MCM-48 was proposed based on the Stöber process. Simultaneously, the catalytic activities of the samples were evaluated in the oxidation of styrene to benzaldehyde using H2O2 as oxidant. The results show that the V-MCM-48 samples have regular spherical morphology, homogeneous dispersion and highly ordered cubic mesostructure. Characterization results about the coordination states of vanadium species demonstrated that most of vanadium existed as tetracoordinated V4+ species in a silicate framework and a small amount of vanadium as isolated V5+ species on the surface. The prepared spherical V-MCM-48 exhibits much higher catalytic activity in the catalytic conversion of styrene to benzaldehyde. Spherical morphology contributes significantly to the improved catalytic performance of materials. V5+ species on the surface and/or produced from the oxidation of some V4+ in the framework act as active sites. Furthermore, the reaction parameters such as vanadium content in V-MCM-48, molar ratio of H2O2/styrene, temperature, time and solvents were optimized.
1. Introduction
Since the researchers at the Mobil Research and Development Corporation reported the existence of the M41S family,1 significant effort has been devoted to the synthesis and application of mesoporous molecular sieves in catalysis,2 sorption,3,4 drug delivery,5 biosensors6 and so on during the past few decades due to their ordered pore arrangement, narrow pore-size distribution, high specific surface area and thermal stability.
MCM-41 and MCM-48 are two typical members in the well-known M41S family. Compared with the one-dimensional (1D) pore system of MCM-41, the three-dimensional (3D) cubic pore network of MCM-48 is recognized as a more promising system with respect to catalytic applications7–9 because it is not only more favorable to the mass transfer in heterogeneous catalysis but also more resistant to pore blocking.10
It is well known that the morphology and the pore structure of supports play very important roles in determining both the diffusion of reactants and products in heterogeneous catalysis.10–15 However, many current studies have focused on the synthesis of MCM-41 with different morphologies, while less attention was paid on MCM-48.16 The synthesis of high-quality MCM-48 with special morphology remains difficult because it can only be produced under delicate experimental conditions. Minor changes in experimental conditions, including pH, temperature, aging time or concentration of the template, may lead to the absence of cubic mesoporous structure.10,17
Due to the lack of catalytic sites for pure mesoporous silicate materials, the incorporation of transition metals,7,18–24 including Ti, Mn, Fe, Cu and V, into the framework of M41S has been widely examined. The doping of transition metals can modify the surface acidity/basicity and the redox property of the mesoporous molecular sieves, and as a result, improve their catalytic activity. There are numerous reports concerning the guest incorporation into mesoporous silicate matrix, including the direct hydrothermal (DHT) method,25,26 the wet impregnation method,27 the grafting method28 and the template ion-exchange (TIE) method.7 In particular, the DHT method is considered to be advantageous because of its simplicity of process and higher efficiency in introducing heteroatoms into silicate framework, whereas other methods either produce more crystalline metal oxide or aggregated metal species outside of the framework, which result in easy leaching of active sites in the liquid-phase reactions.27,29,30 However, the heteroatom-doped mesoporous silica prepared via the DHT method often exhibit poor morphologies.7,29 The synthesis of mesoporous silicate with both heteroatom doping and regular morphology has to be prepared under optimal conditions. Some synthesis parameters should be controlled precisely for the simultaneous hydrolyzation of heteroatom and silicon species, which ensured the heteroatoms in the framework.7,20,25,31 Thus, the synthesis of mesoporous silicate with heteroatoms in the framework and special morphology is full of challenge, particularly in the case of MCM-48.
Vanadium-modified mesoporous molecular sieves exhibit high catalytic activity in many reactions, particularly in the oxidation of aromatics,13,17,32,33 including benzene, phenol and styrene. In this study, V-MCM-48 catalysts with regular spherical morphologies and different vanadium content were synthesized by the DHT method and characterized by various techniques. The catalytic performances of produced catalysts were systematically studied in the oxidation of styrene, which is of considerable commercial and academic interest for the synthesis of important products such as styrene epoxide, benzaldehyde and phenylacetaldehyde.7,34 The possible mechanism for the formation of spherical V-MCM-48 as well as the catalytic process was further discussed.
2. Experimental section
2.1 Materials
Surfactant. Cetyltrimethylammonium bromide (CTAB, 99%) was purchased from J&K Chemical Ltd. Tetraethylorthosilicate (TEOS, AR), acetone (AR) and ammonium metavanadate (NH4VO3, AR) were purchased from Sinopharm Chemical Reagent Co., Ltd. Ammonia solution (25 wt%), ethanol (AR), methanol (AR), acetic acid (AR), acetonitrile (AR), styrene (CP) and hydrogen peroxide (H2O2, 30 wt%) were purchased from Nanjing Chemical Reagent Co., Ltd.
2.2 Synthesis
A series of vanadium-containing MCM-48 samples with spherical morphology were synthesized in the following manner. 1.0125 g cetyltrimethylammonium bromide (CTAB) was dissolved in a mixed solvent composed of 69 mL deionized water, 6.3 mL concentrated ammonia solution (25 wt%) and 32.4 mL ethanol. The pH value of the solution is about 11.0. 10 mL aqueous solution containing the desired amount of NH4VO3 and 2.1 mL tetraethylorthosilicate (TEOS, 0.0093 mol) were then slowly added into the aforementioned template solution under stirring. After being stirred for another 2 h, the resulting gel was transferred into Teflon-lined stainless-steel autoclaves and kept at 100 °C for 24 h. The sample obtained was washed three times with deionized water and ethanol and then dried at room temperature. The as-synthesized product was calcinated at 550 °C in a flow of air for 6 h to remove the template. The obtained samples are denoted as xV-MCM-48, where x is 100 times of the calculated molar ratio of V/Si used in the synthesis gel.
For comparison, V-MCM-48-M (irregular morphology) and V-MCM-48-I (prepared by impregnation) were synthesized referring to the reported literatures ref. 17 and 35, respectively.
2.3 Characterization
The XRD patterns of the samples were collected with a diffractometer (RigakuD/Max-RAX) equipped with a rotating anode and Cu Kα radiation (λ = 0.154178 nm).
N2 adsorption–desorption isotherms were measured on a Micromeritics ASAP-2020 analyzer at 77 K. Before the measurements, calcined samples were degassed in a vacuum at 300 °C for 5 h. Surface areas were calculated using the BET equation and pore-size distributions were obtained by the Barrett–Joyner–Halenda (BJH) method using desorption branch data.
High-resolution transmission electron microscopy (HRTEM) images were recorded on a JEM-2010 EX microscope operated at an accelerating voltage of 200 kV. The samples were crushed in A.R. grade ethanol, and the resulting suspension was allowed to dry on carbon film supported on copper grids.
Field emission scanning electron microscope (FE-SEM) was performed on a Hitachi S4800 Field Emission Scanning Electron Microscopy.
The vanadium content in the samples was determined using a Jarrell-Ash 1100 Inductively Coupling Plasma emission spectrometer. The samples were completely dissolved in hydrofluoric acid before analysis.
Diffuse reflectance UV-vis spectra were recorded by a Perkin-Elmer Lambda 35 spectrophotometer in the range of 200–800 nm with BaSO4 as reference.
The X-ray photoelectron spectra (XPS) were conducted on a PHI 5000 Versa Probe X-ray photoelectron spectrometer equipped with Al Kα radiation (1486.6 eV). The C1s peak at 284.6 eV was used as the reference for binding energies.
Magic angle spinning nuclear magnetic resonance (MAS-NMR) spectra were collected at a Larmor frequency of 105.181 MHz and a magnetic field strength of 9.4 T using a 4.0 mm MAS probe with spinning at 14 kHz on a Bruker Avance III spectrometer.
Electron spin-resonance spectroscopy (ESR) spectra were recorded on a Bruker EMX-10/12 ESR spectrometer with X-band at 110 K.
2.4 Catalyst test
The catalytic testing was carried out in a two-necked flask placed in a water bath. Typically, 0.1 g catalyst, 20 mL solvent and a desired amount of styrene were mixed. When the temperature reached 30–70 °C, a given amount of H2O2 was added dropwise into the reaction mixture under stirring. After reaction for 2–12 h, the catalyst was separated by centrifugation. The product distribution was analyzed by a SP-6890 gas chromatograph (Lunan Ruihong Chemical Instrument Co., Ltd., China) equipped with a 0.32 mm × 30 m SE-54 capillary column and a flame ionization detector (FID). The content of each component was calculated by a revised area normalization method.
3. Results and discussion
3.1 Mesostructure
The small-angle XRD patterns of the calcined V-MCM-48 samples with different vanadium contents are shown in Fig. 1. The sharp (211) reflection peak at 2θ ≈ 2.4° demonstrates the characteristic of a typical mesoporous structure. Three other weak peaks confirmed the presence of a highly ordered 3D cubic Ia3d mesophase, which are indexed to the (220), (420) and (332) reflections.36,37 Therefore, the results indicate that the 3D cubic Ia3d mesostructure was retained for the catalysts modified with vanadium. Moreover, the reflections of the 8V-MCM-48 sample become broad and weak, indicating that the over-doping (molar ratio used in the synthesis gel of V/Si > 0.06) of the vanadium species resulted in the decrease of the order degree of cubic mesostructure. In particular, no characteristic peaks of vanadium oxides were found in high-angle XRD patterns (figures not given), suggesting that the vanadium species should be incorporated into the framework of MCM-41 or disperse highly on the wall.25,38
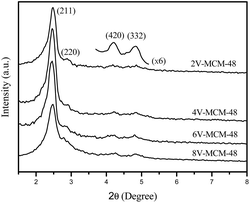 |
| Fig. 1 XRD patterns of the calcined V-MCM-48 samples. | |
The TEM image of 4V-MCM-48 is depicted in Fig. 2a. A well-ordered mesostructure is clearly visualized, and the pore size is found to be in the range of 2.3–2.5 nm, which is in good agreement with the result calculated from the BJH model using desorption data (Fig. 3b). A well-resolved cubic diffraction pattern is observed in the FT pattern (Fig. 2b), which demonstrates the regular three-dimensional pore structure of V-MCM-48 sample.10,17,37 These observations indicate that the moderate incorporation of vanadium atoms barely influence the cubic mesostructure of MCM-48.
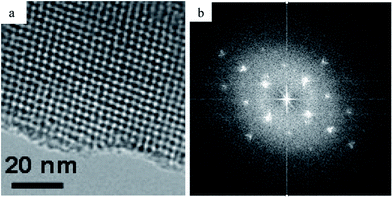 |
| Fig. 2 Representative TEM and SAED images of calcined 4V-MCM-48 samples. | |
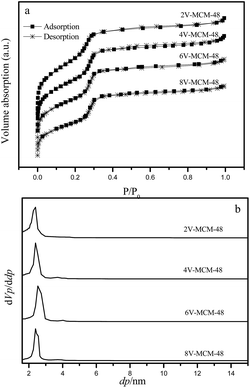 |
| Fig. 3 (a) N2 adsorption–desorption isotherms and (b) pore diameter distributions of calcined V-MCM-48 samples. | |
Fig. 3 shows the N2 adsorption–desorption isotherms and pore-size distributions of all of the samples. The typical IV type isotherms (Fig. 3a) are the characteristic of mesoporous solids.39 Due to the capillary condensations of nitrogen, the steep increases of absorption volume in the P/P0 range of 0.25–0.35 represent the highly ordered mesostructure of the materials and very narrow pore-size distributions, which are evident in Fig. 3b.10,35,37 Some structural parameters of the samples are listed in Table 1. As illustrated in Table 1, no apparent changes are observed for the most probable pore diameter of all the samples, which suggests that the incorporation of vanadium has little influence on the pore-size distribution. The BET surface area and total pore volume decrease with the increase of vanadium content, which perhaps results from the decreased order degree of the mesostructure caused by high amounts of vanadium doping, respectively. The total pore volumes decrease with the increase of vanadium content, which suggests the formation of extra-framework vanadium species.
Table 1 The structural properties of V-MCM-48 samples
Sample |
V/Si molar ratioa/% |
V/Si molar ratiob/% |
SBETc (m2 g−1) |
D211 (nm) |
a0d (nm) |
Dpe (nm) |
Wtf (nm) |
Total pore volume (cm3 g−1) |
Calculated from ICP data. Obtained from XPS data. BET surface area. Unit cell parameter (a0) calculated using a0 = d211√6. Pore sizes obtained from BJH analysis of desorption data. Wall thickness=(a0/3.0919)−[(pore size)/2]. Not available. |
2V-MCM-48 |
0.71 |
—g |
1090 |
3.56 |
8.73 |
2.39 |
1.63 |
0.73 |
4V-MCM-48 |
1.29 |
0.41 |
969 |
3.59 |
8.80 |
2.40 |
1.65 |
0.72 |
6V-MCM-48 |
1.95 |
0.59 |
944 |
3.59 |
8.80 |
2.42 |
1.64 |
0.65 |
8V-MCM-48 |
2.88 |
1.04 |
788 |
3.56 |
8.73 |
2.39 |
1.63 |
0.56 |
The recorded data as well as the aforementioned analyzed results demonstrate that we offer a convenient strategy to produce highly ordered cubic mesoporous materials incorporated with a moderate amount of vanadium. Although the overload of vanadium does not influence on the pore-size distribution, it will inevitably cause the distortion of mesostructure of materials.
3.2 Morphology
The representative SEM and TEM images of samples are shown in Fig. 4. As shown in Fig. 4a, the regularly and homogeneously dispersed spherical particles of 4V-MCM-48 sample are clearly visible. The TEM image (Fig. 4b) confirms the spherical morphology with a uniform particle size of about 0.5 μm. For the 8V-MCM-48 sample (Fig. 4c), although there are a few particles with other morphologies, the spherical particles are dominant. In addition, Fig. 4d shows the irregular sample synthesized as previously reported.17
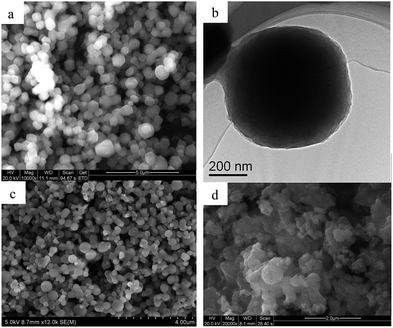 |
| Fig. 4 SEM images of (a) calcined 4V-MCM-48, (c) 8V-MCM-48 and (d) V-MCM-48-M samples and (b) TEM image of calcined 4V-MCM-48. | |
According to the Stöber process,40,41 small particles of silicate are initiated, which aggregate gradually to form a spherical morphology. In this study, we suggested the formation of spherical V-MCM-48 following the similar procedures. As shown in Scheme 1, the micelles are produced from the template solution (step I). Both the TEOS and vanadium sources hydrolyze to form disordered clusters (step II), which grow into large and packed particles (step III). To minimize the surface free energy,10,13 the large-sized particles will further aggregate into a spherical form.
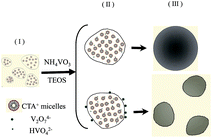 |
| Scheme 1 The proposed formation mechanism of the spherical V-MCM-48 based on the Stöber process. | |
Note that the pH value is ca. 11 and the −log(V/mol L−1) values decrease from 2.6 to 2.1 with the increasing amount of vanadium. According to the occurrence of various vanadate and polyvanadate species as a function of pH and total concentration of vanadium depicted in ref. 42 (page 984), the vanadium species mainly exist as two species, HVO42− and V2O74−.42 In addition, the proportion of V2O74− should increase with the increasing amount of vanadium considering the equation 2[HVO4−]2−
[V2O7]4− + H2O.42 Therefore, HVO42− is reduced to V(IV) by ethanol and hydrolyzes simultaneously with TEOS to form V-MCM-48 clusters with tetrahedral V4+ ions in the framework (step II). Simultaneously, V2O74− species with larger ionic diameters either stay in the solution, which results in materials of much lower vanadium content than expected, or serve as the source of V5+ species in MCM-48.
Therefore, in the cases of lower concentrations of V2O74− in the solution, the MCM-48 clusters may favor the growth into a spherical form. By contrast, at higher concentrations of V2O74−, the MCM-48 clusters will become the irregular particles due to electrostatic repulsion.
3.3 The states of vanadium species
UV-vis. As shown in Fig. 5, the UV-vis spectrum of pure MCM-48 support exhibits little absorption in the range of 200–700 nm.12 All of the V-MCM-48 samples show two distinct absorption bands, in which an intense broad band between 220 nm and 320 nm centered at 260 nm is assigned to low-energy charge-transfer transitions between tetrahedral oxygen ligands and central V4+ ion present in the framework, and the intense band centered at 370 nm is attributed to the isolated V5+ species with tetrahedral structure that incorporated on the silicate surface of MCM-48. The UV-vis absorption band at ca. 450 nm of the octahedral structure of the extra-framework V5+ may be covered by the tails of absorption lines decreasing up to ca. 550 nm.20,43 The band of bulk V2O5 crystallites (ca. 450 to 480 nm) is not observed for all samples,17,18 which accords well with XRD results.
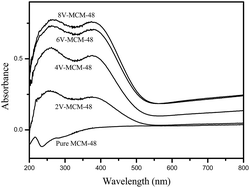 |
| Fig. 5 UV-vis spectra of calcined V-MCM-48 and pure MCM-48 samples. | |
It is found that the intensity of the two absorbance bands increases with the vanadium content, which is more evident for the bands centered at 370 nm indicating the presence of more V5+ species dispersed on the surface.
XPS. The XPS spectra of obtained materials are given in Fig. 6. The broad asymmetrical V2p3/2 spectra can be de-convoluted into two components at about 516.2 eV and 517.4 eV, which are assigned to V4+ and V5+, respectively.32,44 The peak area at 516.2 eV is found to be larger than that of 517.4 eV, indicating that the V4+ species is dominant in V-MCM-48 samples. Moreover, the ratio of peak areas at 517.4 eV relative to 516.2 eV increases with the amount of vanadium species, demonstrating more V5+ species disperse on the surface in the samples. These findings further support the conclusion obtained from UV-vis spectroscopy.
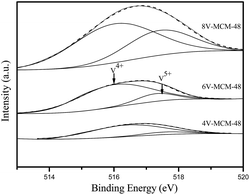 |
| Fig. 6 XPS V 2p3/2 spectra of calcined V-MCM-48 samples. | |
Furthermore, by the inspection of Table 1, it can be seen that the bulk vanadium contents in 4V-MCM-48, 6V-MCM-48 and 8V-MCM-48 samples determined by ICP are much higher than those of surface vanadium content obtained from XPS. This observation indicates that most of vanadium species are incorporated into the framework of MCM-48.
51V MAS-NMR. 51V MAS-NMR spectra of 4V-MCM-48, 6V-MCM-48 and 8V-MCM-48 samples were also recorded. As depicted in Fig. 7, the relatively weak signals at −585 ppm are observed in 6V-MCM-48 and 8V-MCM-48 samples, which are ascribed to the characteristic of V5+ in a tetrahedral environment.17,25,38,45 Moreover, the signal intensity becomes weaker with the decrease in the amount of vanadium, and no distinguished signal is observed for 4V-MCM-48 sample, possibly due to its weak signal-to-noise (S/N) ratio. This observation indicates that the V5+ species exist in greater amounts in the sample with the increasing amount of vanadium, which is consistent with the results of UV-vis and XPS measurements. Meanwhile, there are no characteristic peaks around −300 ppm observed in all samples, suggesting the absence of bulk V2O5 crystallites.17,38
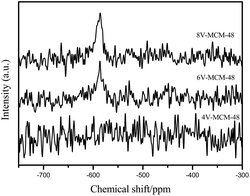 |
| Fig. 7 51V MAS-NMR spectra of calcined V-MCM-48 samples. | |
ESR. To further evaluate the states of vanadium, the ESR spectra of samples were acquired. As shown in Fig. 8, all of the samples exhibit an axially symmetrical signal of V4+, which results from the d1 electron interaction with nuclear spin In = 7/2 of 51V (natural abundance 99.8%).45 The spin Hamiltonian parameters A and g tensors were determined as g|| = 1.94, A|| = 197 G, g⊥ = 1.98, and A⊥ = 64 G, which is typified as tetrahedral V4+ species.25 In addition, the ESR signal intensity of the calcined V-MCM-48 samples increases linearly with the increasing vanadium content. No observation of spin–spin interactions among V4+ ions indicates that V4+ should be highly dispersed in the silicate matrix.25,45,46
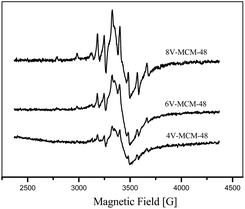 |
| Fig. 8 ESR spectra of calcined V-MCM-48 samples. | |
To summarize the characterization results from UV-vis, XPS, 51V MAS-NMR and ESR, we find that there are two types of vanadium species existing in spherical V-MCM-48 materials that we produced, i.e. the tetrahedral V4+ ions in the framework, and the V5+ species dispersed on the surface, as illustrated in Fig. 9.
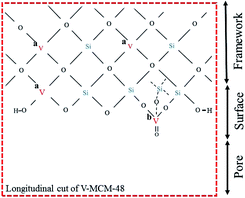 |
| Fig. 9 Proposed structure of V4+ (a) in silicate framework and V5+ (b) on the surface. | |
3.4 Catalytic activity
Catalytic performance of vanadium-containing MCM-48.
Active sites. Table 2 summarizes the catalytic activity of produced materials in styrene oxidation to benzaldehyde. Pure MCM-48 exhibited no catalytic activity due to the absence of active sites.12,13 Crystalline V2O5 exhibits improved catalytic activity, which indicates that the V5+ species act as active sites. Accordingly, V-MCM-48-I prepared by the impregnation method possesses a larger turnover number (TON) value, compared with crystalline V2O5, which should attribute to the dispersion of the V5+ species. Notably, the degree of dispersion of the V5+ species is further improved for V-MCM-48 prepared by DHT method,47 which results in remarkably higher catalytic activity and TON value.
Table 2 Catalytic performance of vanadium-containing catalystsg
Catalyst |
V/Sia (%) |
Conversion (%) |
TONb |
Selectivity (%) |
BZc |
PhAd |
SEe |
BAf |
The molar ratio of V/Si determined by ICP. Turnover number (TON). Benzaldehyde. Phenyl acetaldehyde. Styrene epoxide. Benzoic acid. Reaction condition: temperature, 30 °C; styrene, 2.2 mL; H2O2, 2 mL; catalyst, 0.1 mg; reaction time, 12 h; and acetonitrile, 20 mL. |
Pure MCM-48 |
0.00 |
— |
— |
— |
— |
— |
— |
2V-MCM-48 |
0.71 |
22.0 |
224 |
95.2 |
1.7 |
1.1 |
1.0 |
4V-MCM-48 |
1.29 |
39.2 |
257 |
95.5 |
1.8 |
1.2 |
— |
6V-MCM-48 |
1.95 |
44.1 |
231 |
94.5 |
1.8 |
1.1 |
1.2 |
8V-MCM-48 |
2.88 |
40.1 |
166 |
94.3 |
1.7 |
1.1 |
1.0 |
V-MCM-48-M |
1.35 |
25.6 |
166 |
91.0 |
1.0 |
2.4 |
1.0 |
4V-MCM-48-I |
1.22 |
10.2 |
98 |
91.7 |
1.3 |
1.9 |
1.1 |
8V-MCM-48-I |
2.98 |
18.4 |
70 |
90.3 |
1.2 |
2.1 |
1.0 |
V2O5 |
— |
11.1 |
21 |
91.3 |
1.6 |
1.8 |
1.2 |
Reaction mechanism. Considering that the V5+ acts as active sites, we propose that the oxidation of styrene over vanadium-doping the MCM-48 catalyst should involve the following reaction processes, similar to that over La-MCM-48.48 Three main reaction steps should be involved, as shown in Scheme 2: (1) V5+((SiO)3V
O) and V4+((SiO)3V–OH) species on the surface of materials react with H2O2 to form V-superoxo (VOOH) species; (2) styrene molecules react with V-superoxo species to form styrene epoxide; and (3) styrene epoxide is further oxidized by H2O2 to obtain benzaldehyde.27,48
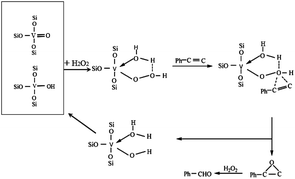 |
| Scheme 2 The proposed reaction mechanism for the oxidation of styrene over the V-MCM-48 catalyst. | |
Effect of spherical morphology. We know that the performance of a catalyst is often related to the amount of active sites, morphology and structural parameters of the support. As listed in Table 2, it is found that the catalytic activities of V-MCM-48 samples first increase and then decrease with the increasing content of vanadium. In addition, the respective structural parameters of all samples, such as total pore volume and BET specific surface area, are observed to be decreased with increased vanadium content (Table 1). Therefore, the unexpected dependence of the catalytic activity of materials on the vanadium content suggests that morphology plays an essential role for the remarkable catalytic performance of the materials. Specifically, for the 2V- and 4V-MCM-48 samples with similar spherical morphology, their catalytic activities are highly related to the amount of vanadium. By comparison, despite its improved vanadium content, an 8V-MCM-48 sample of irregular forms shows a dramatic decrease in catalytic activity. In fact, comparing with the V-MCM-48-M sample with irregular morphology, spherical 4V-MCM-48, which contains the same vanadium content, exhibits markedly improved catalytic activity. These observations can be explained by the fact that the regularly spherical morphology can speed up the mass transport involved in a catalytic reaction in heterogeneous catalysis thus contributing to an improved catalytic performance of materials.11–14 Next, we explored the optimization of reaction condition on the basis of 4V-MCM-48 catalyst.
Optimization of reaction condition.
Molar ratio of H2O2/styrene. In the range of the molar ratio of H2O2/styrene from 0.5 to 2, the styrene conversion is observed to be increased significantly from 23% to 83%. At the higher molar ratio of H2O2/styrene (>2), the styrene conversion decreases. This observation accords well with the reaction mechanism as totally two H2O2 molecules are involved in the reaction. The selectivity to benzaldehyde is observed to be in the range of 89.6–97.7% at different molar ratios of H2O2/styrene. Therefore, we choose the molar ratio of H2O2/styrene equal to 2 for the current study.
Reaction temperature. We find that the styrene conversion first increases with temperature from 30 to 50 °C and then decreases slightly at a higher temperature (70 °C), which should involve the rapid decomposition of hydrogen peroxide. Simultaneously, the selectivity to benzaldehyde decreases at higher temperatures, possibly due to the deep oxidation of products. The highest yield of benzaldehyde is observed at 50 °C.
Reaction time. As observed in Fig. 10, the styrene conversion increases from 40.7% to 52.1% in a reaction time ranging from 2–12 h. Prolonged reaction time makes the styrene conversion almost unchanged with a slight decrease of selectivity to benzaldehyde. 12 h is the optimal reaction time.
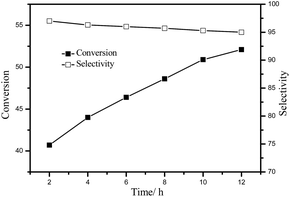 |
| Fig. 10 Effects of reaction time on conversion of styrene and selectivity to benzaldehyde. Reaction conditions: styrene, 2.2 mL; H2O2, 2 mL; catalyst, 0.1 mg; acetonitrile, 20 mL; and temperature, 50 °C. | |
Solvents. For most of the liquid-phase reactions, solvent is an essential factor because it affects the reaction kinetics as well as the product selectivity. Generally, the aprotic solvent is more effective in the catalytic oxidation of aromatics than the protic solvent.48 The solvent of higher polarity will increase the substrate concentration, which favors the catalytic reaction.49 As listed in Table 3, the lower styrene conversion is observed in methanol and acetic acid (protic) than in acetone and acetonitrile (aprotic). Because acetonitrile possesses a higher polarity than that of acetone, the styrene conversion in acetonitrile is found to be higher. The acidity of acetic acid is favorable to the open-ring (C–O–C) reaction in styrene epoxide as mentioned in the second step of the reaction mechanism, which results in a higher selectivity to benzaldehyde. On the contrary, methanol is involved in the third step of reaction mechanism, which makes the selectivity to benzaldehyde much lower.48
Table 3 Effects of solvents on the catalytic performancea
Solvent |
Conversion (%) |
Selectivity (%) |
BZ |
PhA |
SE |
BA |
Reaction condition: styrene, 2.2 mL; H2O2, 2 mL; temperature, 50 °C; catalyst, 0.1 mg; reaction time, 12 h; and solvent, 20 mL. |
Methanol |
22.1 |
37.3 |
0.8 |
7.0 |
23.6 |
Acetic acid |
39.1 |
93.8 |
0.9 |
1.0 |
1.9 |
Acetone |
42.9 |
84.5 |
0.94 |
2.3 |
0.9 |
Acetonitrile |
52.1 |
96.5 |
0.8 |
1.1 |
0.6 |
Stability. From the test of catalyst stability, we found that the conversion of styrene decreased from 39.2% (fresh catalyst), 28.5% (first recycle) to 12.3% (second recycle), owing to the leaching of a small amount of vanadium ions, and the selectivity to benzaldehyde is in the range of 90.2–95.5%. The result indicated that 4V-MCM-48 is more resistant to the leaching of vanadium ions because of the vanadium ions incorporated in the silicate framework, which is necessary for the stability of the vanadium-containing silicate catalyst.From the aforementioned experimental results, the 4V-MCM-48 catalyst exhibited the best activity with 83.0% conversion of styrene and 90.5% selectivity to benzaldehyde under the optimized reaction conditions of the 2
:
1 molar ratio of H2O2/styrene with acetonitrile as solvent at 50 °C for 12 h.
4. Conclusions
We have successfully synthesized a series of V-MCM-48 samples with various contents of vanadium, which exhibit the spherical morphology and highly ordered 3D cubic pore structures, by a one-step hydrothermal method. The spherical particles are formed following the Stöber process, in which small particles with 3D-cubic mesoporous structure start to form after the hydrolysis of silicon and vanadium sources on the pre-aggregated micelles of the template, and then grow into spherical morphology.
The characterization results reveal the existence of two types of vanadium species, including the tetrahedral V4+ in the framework and surface-dispersed V5+ in the produced materials. We identify that the V5+ species on the surface and/or produced from the oxidation of some V4+ in the framework by H2O2 acting as active sites in the catalytic conversion of styrene to benzaldehyde. Although the catalytic performance of materials is closely related to the number of active vanadium species, the spherical morphology plays a dominant role due to the speeding up of mass transport. Under the optimal reaction condition, the conversion of styrene and the selectivity to benzaldehyde are 83.0% and 90.5%, respectively. Taken altogether, we offer a facile method to synthesize spherical V-MCM-48 with excellent catalytic activity.
Acknowledgements
The authors acknowledge the financial support of the National Natural Science Foundations of China (21276125, 20876077 and 21476108), the Major Projects of the Natural Science Foundation for the Higher Education Institutions of Jiangsu Province (10KJA530015), the Program for Scientific Innovation Research of College Graduates in Jiangsu Province (CXZZ13_0443), the Key Program of National Natural Science Foundation of China (no. 21136005) and the Project of Priority Academic Program Development of Jiangsu Higher Education Institutions (PAPD).
Notes and references
- C. T. Kresge, M. E. Leonowicz, W. J. Roth, J. C. Vartuli and J. S. Beck, Nature, 1992, 359, 710–712 CrossRef CAS.
- M. Egi, K. Sugiyama, M. Saneto, R. Hanada, K. Kato and S. Akai, Angew. Chem., Int. Ed., 2013, 52, 3654–3658 CrossRef CAS PubMed.
- S. Dib, M. Boufatit, S. Chelouaou, F. Sadi-Hassaine, J. Croissant, J. Long, L. Raehm, C. Charnay and J. O. Durand, RSC Adv., 2014, 4, 24838–24841 RSC.
- Y. Zhou, W. G. Lin, M. M. Wan, J. Yang and J. H. Zhu, J. Mater. Chem., 2012, 22, 23633–23641 RSC.
- F. Muharnmad, M. Guo, W. Qi, F. Sun, A. Wang, Y. Guo and G. Zhu, J. Am. Chem. Soc., 2011, 133, 8778–8781 CrossRef PubMed.
- S. H. Cheng, C. H. Lee, C. S. Yang, F. G. Tseng, C. Y. Mou and L. W. Lo, J. Mater. Chem., 2009, 19, 1252 RSC.
- S. Gomez, L. J. Garces, J. Villegas, R. Ghosh, O. Giraldo and S. L. Suib, J. Catal., 2005, 233, 478 CrossRef CAS PubMed.
- T. W. Kim, F. Kleitz, B. Paul and R. Ryoo, J. Am. Chem. Soc., 2005, 127, 7601–7610 CrossRef CAS PubMed.
- T. Suteewong, H. Sai, R. Cohen, S. T. Wang, M. Bradbury, B. Baird, S. M. Gruner and U. Wiesner, J. Am. Chem. Soc., 2011, 133, 172–175 CrossRef CAS PubMed.
- T. W. Kim, P. W. Chung and V. S. Y. Lin, Chem. Mater., 2010, 22, 5093–5104 CrossRef CAS.
- T. M. Suzuki, T. Nakamura, K. Fukumoto, M. Yamamoto, Y. Akimoto, Y. Akimoto and K. Yano, J. Mol. Catal. A: Chem., 2008, 280, 224–232 CrossRef CAS PubMed.
- A. I. Carrillo, E. Serrano, R. Luque and J. Garcia-Martinez, Appl. Catal., A, 2013, 453, 383–390 CrossRef CAS PubMed.
- Y. Chen, X. Shi, B. Han, H. Qin, Z. Li, Y. Lu, J. Wang and Y. Kong, J. Nanosci. Nanotechnol., 2012, 12, 7239–7249 CrossRef CAS PubMed.
- G. C. Bond, Heterogeneous Catalysis: Principles and Applications, 2nd edn, 1987 Search PubMed.
- Z. Yang, Y. Lu and Z. Yang, Chem. Commun., 2009, 2270–2277 RSC.
- C. C. Liu, X. M. Wang, S. Lee, L. D. Pfefferle and G. L. Haller, Microporous Mesoporous Mater., 2012, 147, 242–251 CrossRef PubMed.
- P. Selvam and S. E. Dapurkar, Appl. Catal., A, 2004, 276, 257–265 CrossRef CAS PubMed.
- S. Q. Hu, D. P. Liu, L. S. Li, A. Borgna and Y. H. Yang, Catal. Lett., 2009, 129, 478–485 CrossRef CAS PubMed.
- J. Strunk, W. C. Vining and A. T. Bell, J. Phys. Chem. C, 2010, 114, 16937–16945 CAS.
- Y. Kong, R. Zhang, X. J. Xu, Z. L. Wang and J. Wang, Chin. J. Inorg. Chem., 2008, 24, 1124–1127 CAS.
- C. Wu, Y. Kong, F. Gao, Y. Wu, Y. N. Lu, J. Wang and L. Dong, Microporous Mesoporous Mater., 2008, 113, 163–170 CrossRef CAS PubMed.
- Y. Kong, X. J. Xu, Y. Wu, R. Zhang and J. Wang, Chin. J. Catal., 2008, 29, 385–390 CrossRef CAS.
- F. T. Kuo, S. Y. Chen, T. H. Lin, J. F. Lee and S. Cheng, RSC Adv., 2013, 3, 12604–12610 RSC.
- J. Sun, Q. Kan, Z. Li, G. Yu, H. Liu, X. Yang, Q. Huo and J. Guan, RSC Adv., 2014, 4, 2310–2317 RSC.
- F. Gao, Y. H. Zhang, H. Q. Wan, Y. Kong, X. C. Wu, L. Dong, B. Q. Li and Y. Chen, Microporous Mesoporous Mater., 2008, 110, 508–516 CrossRef CAS PubMed.
- M. A. Betiha, H. M. A. Hassan, A. M. Al-Sabagh, A. E. R. S. Khder and E. A. Ahmed, J. Mater. Chem., 2012, 22, 17551–17559 RSC.
- M. Piumetti, M. Armandi, E. Garrone and B. Bonelli, Microporous Mesoporous Mater., 2012, 164, 111–119 CrossRef CAS PubMed.
- S. Samanta, S. Das, P. K. Samanta, S. Dutta and P. Biswas, RSC Adv., 2013, 3, 19455–19466 RSC.
- G. Zhang, J. Long, X. Wang, Z. Zhang, W. Dai, P. Liu, Z. Li, L. Wu and X. Fu, Langmuir, 2010, 26, 1362–1371 CrossRef CAS PubMed.
- L. L. Lou, S. Jiang, K. Yu, Z. Gu, R. Ji, Y. Dong and S. Liu, Microporous Mesoporous Mater., 2011, 142, 214–220 CrossRef CAS PubMed.
- C. He, J. Li, P. Li, J. Cheng, Z. Hao and Z. P. Xu, Appl. Catal., B, 2010, 96, 466–475 CrossRef CAS PubMed.
- B. Guo, L. F. Zhu, X. K. Hu, Q. Zhang, D. M. Tong, G. Y. Li and C. W. Hu, Catal. Sci. Technol., 2011, 1, 1060–1067 CAS.
- L. Y. Hu, B. Yue, X. Y. Chen and H. Y. He, Catal. Commun., 2014, 43, 179–183 CrossRef CAS PubMed.
- Y. Wu, Y. J. Zhang, J. Cheng, Z. Li, H. Q. Wang, Q. L. Sun, B. Han and Y. Kong, Microporous Mesoporous Mater., 2012, 162, 51–59 CrossRef CAS PubMed.
- A. Benhamou, M. Baudu, Z. Derriche and J. P. Basly, J. Hazard. Mater., 2009, 171, 1001–1008 CrossRef CAS PubMed.
- A. Sayari, J. Am. Chem. Soc., 2000, 122, 6504–6505 CrossRef CAS.
- W. Zhao, Q. Li, L. Wang, J. Chu, J. Qu, S. Li and T. Qi, Langmuir, 2010, 26, 6982–6988 CrossRef CAS PubMed.
- M. Piumetti, B. Bonelli, M. Armandi, L. Gaberova, S. Casale, P. Massiani and E. Garrone, Microporous Mesoporous Mater., 2010, 133, 36–44 CrossRef CAS PubMed.
- W. Zhao, Q. Z. Li, L. N. Wang, J. L. Chu, J. K. Qu, S. H. Li and T. Qi, Langmuir, 2010, 26, 6982–6988 CrossRef CAS PubMed.
- K. T. Lee, A. N. Sathyagal and A. V. McCormick, Colloids Surf., A, 1998, 144, 115–125 CrossRef CAS.
- K. Schumacher, P. I. Ravikovitch, A. Du Chesne, A. V. Neimark and K. K. Unger, Langmuir, 2000, 16, 4648–4654 CrossRef CAS.
- N. N. Greenwood and A. Earnshaw, Chemistry of the Elements, 2nd edn, 1997 Search PubMed.
- C. Subrahmanyam, B. Louis, B. Viswanathan, A. Renken and T. K. Varadarajan, Appl. Catal., A, 2005, 282, 67–71 CrossRef CAS PubMed.
- C. T. Wang, M. T. Chen and D. L. Lai, Appl. Surf. Sci., 2011, 257, 5109–5114 CrossRef CAS PubMed.
- M. Chatterjee, T. Iwasaki, H. Hayashi, Y. Onodera, T. Ebina and T. Nagase, Chem. Mater., 1999, 11, 1368–1375 CrossRef CAS.
- A. Held, J. Kowalska-Kus and K. Nowinska, Catal. Commun., 2012, 17, 108–113 CrossRef CAS PubMed.
- M. Piumetti, B. Bonelli, P. Massiani, S. Dzwigaj, I. Rossetti, S. Casale, M. Armandi, C. Thomas and E. Garrone, Catal. Today, 2012, 179, 140–148 CrossRef CAS PubMed.
- W. C. Zhan, Y. L. Guo, Y. Q. Wang, X. H. Liu, Y. Guo, Y. S. Wang, Z. G. Zhang and G. Z. Lu, J. Phys. Chem. B, 2007, 111, 12103–12110 CrossRef CAS PubMed.
- D. Chen, N. Li, P. Sun and Y. Kong, Chin. J. Catal., 2009, 30, 643–648 CAS.
|
This journal is © The Royal Society of Chemistry 2014 |
Click here to see how this site uses Cookies. View our privacy policy here.