DOI:
10.1039/C4RA07285E
(Paper)
RSC Adv., 2014,
4, 37838-37848
Anticancer (in vitro) and antimicrobial effect of gold nanoparticles synthesized using Abelmoschus esculentus (L.) pulp extract via a green route
Received
18th July 2014
, Accepted 29th July 2014
First published on 29th July 2014
Abstract
Green synthesis of gold nanoparticles (Au NPs) using Abelmoschus esculentus (L.) pulp extract has been elaborately studied and reported here. The Au NPs have been characterized using several techniques. Optical analysis indicates adequate stability of the synthesized Au NPs, while FTIR analyses the fact that phytochemicals present in the Abelmoschus esculentus (L.) pulp extract play the key role in stabilizing the Au NPs. Morphological study shows that the nanoparticles are mostly spherical in shape with an average particle size of ∼14 nm, and these results are comparable with the particle size obtained from XRD. The selected area electron diffraction pattern indicates the crystalline nature of the Au NPs, which is further confirmed from XRD studies. The present study also demonstrates the in vitro efficacy of Au NPs against Jurkat cells. Results show that the IC50 dose of Au NPs is capable of significantly elevating intracellular reactive oxygen species and diminishing mitochondrial membrane potential, indicating the effective involvement of apoptosis in cell death. Furthermore, the synthesized Au NPs show a sufficient degree of antimicrobial activity against different types of bacteria. These results clearly show that the Abelmoschus esculentus (L.) pulp synthesized Au NPs have excellent medicinal applications.
Introduction
Vast exploration and the rapid exploitation of nanotechnology offers pathways for the development of new materials on the nanometer scale, including nanoparticles. These are usually defined as those particles where at least one dimension is less than 100 nanometers (nm); the particles could even be zero dimension as in the case of quantum dots. Now the question arises: Why are these nanoparticles so interesting even if they are quite challenging to handle and synthesize compared to their macroscopic bulk counterparts?” The answer lies in their distinctive features such as optical,1 antimicrobial,2,3 cancer therapeutic4 and catalytic5 properties, which can be tuned with the shape and size of the nanoparticles as an effect of quantum confinement of electrons. Nanoparticles play a pivotal role as the ‘bridging element’ between bulk materials and atomic or molecular structures. Metal nanoparticles with their unparalleled properties are extensively investigated due to their promising applications in diverse areas like electronics, coatings, packaging, and biotechnology. Nondestructive attachment of metal nanoparticles to single stranded DNA opens up the prospect of medical diagnostic applications.6,7 Transparent metallic nanoparticles are a promising vehicle in cosmetics, coatings and packaging applications, whereas their tendency to merge into a solid at lower temperature makes them an important substance in electronic applications (e.g. capacitors). Among the noble metal nanoparticles, gold has enormous potential in applications such as DNA sequencing,8 waveguides,9 catalysis,10 and electron microscopy markers.11 Gold nanoparticles (Au NPs), a highly versatile deep-tissue imaging agent for various detection and imaging applications, show their efficiency in clinical applications.12–15 Some interesting shapes of Au NPs, such as nanotripods,16 nanowires and nanosheets,17 nanoprisms,18 nanotriangles,19–21 nanotubes,22 and nanopyramids,23 have been synthesized through a chemical reduction method. Different existing synthetic techniques for metal nanoparticles, such as bottom-up and top-down approaches or pyrolysis and wet-chemical approaches, have several drawbacks, including surface imperfection, energy consumption, use of toxic solvents, and hazardous by-products. Synthesis of nanoparticles using microorganisms or different parts of plants can potentially eliminate these problems by making nanoparticles more biocompatible. Nanobiotechnology is a field where the nanoscale principle and techniques are used to understand and transform bio systems (living and non-living) and vice versa.24 While microorganisms such as fungi and bacteria play significant roles in metal nanoparticle synthesis, the use of parts of plants or whole plants in similar synthesis methodologies is a stimulating prospect that is currently under enormous investigation, having significant potential utilization. In this field, the first ever report of green synthesis of metal nanoparticles was published by Jose-Yacaman and co-workers.25,26 From an environmental viewpoint, this green process meets the requirements of utilization of non-toxic, environmentally benign, renewable materials towards nanoparticle synthesis. Nowadays, extensive studies on the synthesis of gold and silver nanoparticles using different natural products like lemongrass,20 Lactobacillus strains,27 Paederiafoetida L. leaf extract,28 Shewanella algae,29 Rhodococcus sp.,30 Fusarium oxysporum,31 neem,32 aloe vera,33 Cinnamomum camphora,34 are reported in the literature.
Recently, metal nanoparticles exhibiting novel chemical and physical properties due to their extremely small size and high surface area to volume ratio have received tremendous attention. The antibacterial properties of such noble metal nanoparticles have been studied by a number of researchers.35,36 Gold is known to have disinfecting effects and has been used in applications ranging from traditional medicines to culinary items. Moreover, several salts of gold, silver and their derivatives are commercially manufactured as antimicrobial agents.37,38 In small concentrations, gold is safe for human cells, but lethal for bacteria and viruses.39–41 Recently, Mishra et al.42 tested the antibacterial action of gold nanoparticles against different pathogenic bacteria. Worldwide statistical reports show that cancer is the third leading cause of death (after heart disease and stroke) in developed countries and the second leading cause of death (after heart disease) in the United States, according to http://www.cdc.gov. It has been reported that worldwide, 10 million new cancer cases occurred, 6 million deaths occurred, and 22 million people were living with cancer in the year 2000.43 Recent advancements in nanotechnology open a new area of interdisciplinary research for effective implication of nano-size ranged materials for cancer diagnosis and therapy.15 Au NPs, one of the most well studied materials, exhibit unique physicochemical properties, including surface plasmon resonance (SPR) and the ability to bind to amine and thiol groups. These properties of Au NPs allow surface modification as well as vast use in biomedical applications.44 Previously few studies have been carried out to explore the involvement of reactive oxygen species (ROS) in causing DNA damage following exposure to Au NPs in different cell types.45,46
In this work, we report the simple one-step green approach of synthesizing extracellular Au NPs using Abelmoschus esculentus (L.) pulp extract. The phytochemicals present in the Abelmoschus esculentus (L.) pulp extract are used as an effective reducing and capping agent. Abelmoschus esculentus (L.) pulp, a purely natural product with high medicinal value, has drawn our interest towards the green synthesis of metal nanoparticles, i.e., Au NPs via an eco-friendly process. The Au NPs have been characterized using several techniques like ultraviolet-visible (UV-Vis) spectroscopy, dynamic light scattering (DLS), transmission electron microscopy (TEM), X-ray diffraction (XRD) and Fourier transform infrared (FTIR) spectroscopy studies. The present study also shows the anti-proliferative activity of Au NPs against the human T cell myeloma cell line (Jurkat). Furthermore, it is observed that the synthesized Au NPs show a sufficient degree of antimicrobial activity against different types of bacteria.
Experimental section
Materials
Analytical grade chloroauric acid was purchased from Sigma Aldrich and used as received without further purification. The Abelmoschus esculentus was collected from the local market of our institute.
Culture media and chemicals
RPMI 1640, penicillin and streptomycin were procured from Sigma Aldrich (St. Louis, MO). Fetal bovine serum (FBS) was purchased from GIBCO/Invitrogen. 4′,6-Diamidino-2-phenylindole dihydrochloride (DAPI) was purchased from Sigma-Aldrich. Commercially available dimethyl sulfoxide (DMSO), sodium dodecyl sulphate (SDS), rhodamine 123, ethidium bromide, acridine orange, 3-(4,5-dimethyl-2-thiazolyl)-2,5-diphenyl-tetrazolium bromide (MTT) reagents were purchased from Himedia, India. All other chemicals were from Merck Ltd. and SRL Pvt. Ltd., Mumbai, and were of the highest purity grade available. Solvents were dried according to standard procedure and distilled prior to use.47
Synthesis of gold nanoparticles
The required amount of small slices of fresh Abelmoschus esculentus (L.) pulp was taken into triple distilled water and left for 2 h in order to maximize the diffusion of phytochemicals present in the Abelmoschus esculentus (L.) pulp to the distilled water. After that, the pulp was taken out from the medium and the solution was subjected to centrifugation to remove any impurities present in the stock. The supernatant was carefully collected and used as the extract of Abelmoschus esculentus (L.) pulp. The extract was mixed into an aqueous solution of 1 mM chloroauric acid (4
:
1 v/v) at room temperature with continuous stirring for approximately 6 h. Then, the colloidal solution was left undisturbed for 18 h at room temperature. The formation of Au NPs was confirmed as observed by the colour change of this colloidal solution from colourless to light violet.
Cell line culture and maintenance
Jurkat (human acute myeloid leukemia), K562 (human chronic myeloid leukemia), and DL (Dalton's lymphoma) cell lines were obtained from NCCS, Pune (India). These cell lines were cultivated and maintained in RPMI-1640 complete media supplemented with 10% heat-inactivated fetal bovine serum (FBS), 100 U ml−1 penicillin, 100 μg ml−1 streptomycin and 4 mM L-glutamine under 5% CO2 and 95% humidified atmosphere at 37 °C in a CO2 incubator. Cells were used for different experiments until the number of cells reached 1.0 × 106 cells per ml.
Isolation of peripheral blood lymphocytes
For collection of peripheral blood lymphocytes (PBL), blood samples were collected from six healthy human volunteers by venepuncture in 5 ml heparin-coated vacutainers (Becton, Dickinson and Company, India) according to the method of Hudson and Hay.48 Five milliliters of blood were diluted 1
:
1 with PBS and layered onto a Histopaque 1077 (Sigma-Aldrich Co. LLC, US) using a Pasteur pipette and centrifuged at 400 g (1500 rpm) for 40 min at room temperature. The upper monolayer of buffy coat, i.e. lymphocytes, was transferred using a clean Pasteur pipette to a clean centrifuge tube and washed three times in balanced salt solution. The peripheral blood lymphocytes (PBL) were re-suspended in RPMI complete media supplemented with 10% FBS and incubated for a day at 37 °C in a 95% air/5% CO2 atmosphere in a CO2 incubator.
Drug preparation
One milligram per milliliter stock of Au NPs was prepared by concentrating the solution containing the prepared Au NPs using a rotary evaporator. The stock solution of Au NPs was then serially diluted with RPMI media to prepare working concentrations.
Experimental design
The PBL, Jurkat, K562 and DL cells were divided into 11 groups. Each group contained 6 petri-dishes (2 × 105 cells in each). The following groups were considered for the experiment and cultured for 24 h:
Group I: Control i.e., Cells + culture media, Group II: Cells + 1 μg ml−1 Au NPs in culture media, Group III: Cells + 5 μg ml−1 Au NPs in culture media, Group IV: Cells + 10 μg ml−1 Au NPs in culture media, Group V: Cells + 25 μg ml−1 Au NPs in culture media, Group VI: Cells + 50 μg ml−1 Au NPs in culture media.
After the treatment schedule the cells were collected from the petri dishes separately and centrifuged at 2200 rpm for 10 min at 4 °C to separate the cells and supernatant.49 The cells were washed twice with 50 mM PBS at pH 7.4. The intact cells were used for mitochondrial membrane potential and ROS measurement.
In vitro cell viability assay (MTT assay)
PBL, Jurkat, K562 and DL cells were maintained in RPMI-1640 supplemented with 10% FBS, penicillin (100 U ml−1), and streptomycin (100 μg ml−1) in a humidified atmosphere of 5% CO2 at 37 °C. The cytotoxicity of synthesized Au NPs on PBL, Jurkat, K562 and DL cells were determined by the MTT assay.50 The presence of any anticancer components in the Abelmoschus esculentus (L.) pulp extract was examined by performing a cell viability study on Jurkat cells by the MTT assay.50 Different doses of extract (0–500 μl) were applied to determine any anticancer role of the extract against Jurkat cells. The extract doses selected for this study covered the total volume of Au NPs doses applied. Cells (2 × 104 per well) were plated in 100 μl of medium per well in 96 well plates. After 48 h of incubation, the cells reached confluence, and then the cells were incubated in the presence of various concentrations of sample in 0.1% DMSO for 48 h at 37 °C. After removal of the sample solution and washing with phosphate buffered saline (pH 7.4), 20 μl per well (5 mg ml−1) of 0.5% 3-(4,5-dimethyl-2-thiazolyl)-2,5-diphenyl-tetrazolium bromide (MTT) in phosphate-buffered saline solution were added. After 4 h of incubation, 0.04 M HCl/isopropanol was added to each well. Viable cells were determined by the absorbance at 570 nm with reference at 655 nm, and measurements were performed 3 times. The optical density (OD) at 570 nm was measured on an ELISA reader (Bio-Red, Japan) using wells without the sample containing cells as blanks. All experiments were performed in triplicate, and the effect of the Au NPs on the proliferation of Jurkat cells was expressed as the % of cell viability using the following formula:
% cell viability = [ODsample − ODcontrol] × 100/ODcontrol |
Assay for antimicrobial activity of gold nanoparticles against microorganisms
Gold nanoparticles in sterilized deionized water were tested for their antibacterial activity by the agar diffusion method. Five bacterial strains, Bacillus subtilis [MTCC 736], Bacillus cereus [MTCC 306], Pseudomonas aeruginosa [MTCC 8158], Micrococcus luteus [MTCC 1538] and Escherichia coli [MTCC 68] were used for this analysis. These bacteria, grown in liquid nutrient agar media (HiMedia Laboratories Pvt. Ltd., Mumbai, India) for 24 h prior to the experiment, were seeded in agar plates by the pour plate technique. A cup was made using a cork borer (10 mm diameter) and was filled with the gold nanoparticle solution (0.2 mg ml−1) and then incubated at 37 °C for 24 h. Simultaneously, the control sets (only plant extract and sterilized deionized water respectively) were also examined against the same five bacterial strains, and every experiment was repeated three times.
Characterization
The formation of Au NPs was monitored by measuring the UV-Vis spectrum of the reaction medium in the wavelength range from 300 to 700 nm. UV-Vis absorption spectrum of the sample was performed using an Agilent 8453 Spectrophotometer, USA. UV-Vis studies were performed using a quartz cell with a thickness of 1 cm in the wavelength range of 190–1100 nm. Dynamic light scattering (also known as photon correlation spectroscopy or quasi-elastic light scattering) technique was used for determining the particle size distribution and the nature of the particle's motion in the medium. The light scattering technique of the synthesized gold nanoparticles was observed using Malvern Zetasizer Version 6.20. Furthermore, the size, shape, and morphology of the resultant nanoparticles were confirmed by TEM. A specimen for the TEM sample was made by casting a drop of suspension on a carbon-coated copper grid, and the excess solution was removed by tissue paper and allowed to air dry at room temperature overnight. The TEM study was performed on a HRTEM, JEOL JEM 2010 at an accelerating voltage of 200 kV and fitted with a CCD camera. The crystal structure of the produced Au NPs was determined and confirmed by XRD analysis. The sample for XRD analysis was prepared by depositing the centrifuged sample on a microscopic glass slide and air-dried overnight. The diffractogram was recorded on a PANalytical, XPERT-PRO diffractometer using CuKα (λ = 1.54060 Å) as an X-ray source. Fourier transform infrared (FTIR) spectroscopy measurements were performed using a Perkin Elmer Spectrum Express Version 1.03.00. The scanning data was obtained from the average of 47 scans in the range between 4000 and 400 cm−1 with a resolution of 4 cm−1.
For anticancer activity
Calculation of IC50 value. The concentration required for a 50% inhibition of viability (IC50) was determined graphically. Multiple linear regressions were used to compare data using the Statistica version 5.0 (Statsoft, India) software package.
Intracellular ROS measurement. ROS measurement was performed using 2′,7′-dichlorodihydrofluorescein diacetate (H2DCFDA) according to the method of Roy et al.51 In brief, Jurkat cell lines were treated with Au NPs at 8.17 μg ml−1 for 24 h. After treatment, schedule cells were washed with culture media, followed by incubation with 1 μg ml−1 H2DCFDA for 30 min at 37 °C. Then, the cells were washed three times with fresh culture media. DCF fluorescence was determined at 485 nm excitation and 520 nm emission using flow cytometry, by setting appropriate filters. The cell population in M2 phase was noted as the ROS positive cells, where the viable/ROS negative cells were denoted as M1.
Measurement of mitochondrial membrane potential (ΔΨm)
The alteration of mitochondrial membrane potential by the spectro-fluorometric method was done according to our previous reported method.50 In brief, Jurkat cell lines (2 × 104 per well) were treated with Au NPs at 8.17 μg ml−1 for 24 h. After treatment, schedule cells were washed with culture media followed by incubation with 1.5 μM Rhodamine 123 for 10 min at 37 °C in a humidified incubator. Then, the cells were washed three times with culture media. The cellular fluorescence intensity of Rh 123 was monitored for 2 min using a Hitachi F-7000 fluorescence spectrophotometer. Cellular mitochondrial membrane potential was expressed as a percentage of the control cells at an excitation wavelength of 493 nm and an emission wavelength of 522 nm. Both excitation and emission slit widths were set to 5.0.
Cellular morphology analysis by acridine orange (AO)–ethidium bromide (EtBr) double staining
To confirm the probable pathway of cell death, we analyzed the cells by the EtBr-AO double staining method. A number of 2 × 104 Jurkat cells were seeded into each well of a 6-well plate and incubated for 24 h at 37 °C in a humidified 5% CO2 atmosphere. Au NPs at 8.17 μg ml−1 were then added to the well for 24 h. After incubation, cells were washed once with phosphate buffer saline (PBS). Ten microlitres of the cells were then placed on a glass slide and mixed with 10 μl acridine orange (50 μg ml−1) and ethidium bromide (50 μg ml−1). The cells were viewed under a fluorescence microscope (NIKON ECLIPSE LV100POL) with 400× magnification.52
Assessment of nuclear morphological changes by 4′,6-diamidino-2-phenylindole dihydrochloride (DAPI) staining
For DAPI staining, all the test cells were seeded into six well plates. A number of 2 × 105 cells per ml were treated with or without Au NPs (0 and 8.17 μg ml−1) for 24 h and were then isolated for DAPI staining according to the method of Lin et al.53 with some modification. After treatment, the cells were fixed with 2.5% glutaraldehyde for 15 min, permeabilized with 0.1% Triton X-100 and stained with 1 μg ml−1 DAPI for 5 min at 37 °C. The cells were then washed with PBS and examined by fluorescence microscopy (NIKON ECLIPSE LV100POL).
Caspase-3 activity
Involvement of apoptosis was measured by measuring caspase 3 levels using ELISA according to the manufacturer's instructions (eBioscience, India). In brief, Jurkat cell lines were treated with Au NPs at 8.17 μg ml−1 for 24 h and after treatment schedule 10 μl of Jurkat cell extracts were taken and used as samples to examine caspase 3 activity at the wavelength of 450 nm.
Statistical analysis
All the parameters were repeated at least three times. The data was expressed as mean ± SEM, n = 06. Comparisons between the means of control and treated groups were made by one-way ANOVA test (using a statistical package, Origin 6.1, Northampton, MA) with multiple comparison t tests, p < 0.05 as a limit of significance.
Results and discussion
UV-Vis absorption spectroscopy analysis
The UV-Vis spectra of as-synthesized Au nanoparticles were acquired to investigate the optical properties. The surface plasmon resonance (SPR) absorption band arises due to the coherent oscillation of the electrons in the conduction band of the nanoparticles induced by the electromagnetic field. The distinct colour of colloidal gold nanoparticle solution arises from their surface property known as SPR, attributed to the nanodimensions of gold. It is reported that gold nanoparticles exhibit a violet colour in aqueous solution due to the excitation of surface plasmon vibrations in the metal nanoparticles.54 When Abelmoschus esculentus (L.) pulp extract is mixed into an aqueous solution of the gold ion complex, the solution starts to change colour from colourless to light violet due to the reduction of gold ions present in the Abelmoschus esculentus (L.) pulp extract, which indicates the formation of Au NPs. Fig. 1 shows the UV-Vis spectra of the synthesized Au NPs which give a sharp absorbance peak at around 538 nm at room temperature. The peak originates from the excitation of electrons in the conduction band of Au NPs induced by the electromagnetic field.
 |
| Fig. 1 UV-Vis absorption spectra of Au NPs synthesized by treating 0.001 M aqueous chloroauric acid solution with Abelmoschus esculentus (L.) pulp extract at room temperature. | |
Analysis of the stability of nanoparticles
The stability of synthesized metal nanoparticles is strictly dependent on the degree of aggregation, which can be effectively reflected by changes in absorption characteristics, especially the displacement of the SPR band for gold nanoparticles in the UV-Vis spectrum.55 It is quite obvious that the strong aggregation of metal nanoparticles indicates the red shift of the SPR band observed in the UV-Vis spectra. In this work, we have investigated the stability of gold nanoparticles synthesized using Abelmoschus esculentus (L.) pulp extract after a 5 month storage period at room temperature, and the UV-Vis spectra (Fig. 2) give the SPR band at 538 nm without any significant change in absorbance intensity. The result indicates that the as-prepared gold nanoparticles are very stable at room temperature for several months with negligible aggregation.
 |
| Fig. 2 UV-Vis absorption spectra of Au NPs stabilized with Abelmoschus esculentus (L.) pulp extract (a) after reaction termination; (b) after storage for 5 months at room temperature. | |
Dynamic light scattering (DLS) measurement
The dynamic light scattering (DLS) technique is used to determine the particle size and particle size distribution profile of small particles in suspension. The average mean size of the gold nanoparticles is 23.27 nm, as shown in Fig. 3.
 |
| Fig. 3 DLS showing particle size distribution of gold nanoparticles prepared by treating 0.001 M aqueous chloroauric acid solution with Abelmoschus esculentus (L.) pulp extract at room temperature. | |
Transmission electron microscopy (TEM) measurement
A high-resolution transmission electron microscope (HRTEM) was used for morphological analysis of the as-synthesized Au NPs. It is clear from Fig. 4a that the synthesized Au NPs are mostly spherical in nature and a small number of hexagonal and trigonal nanoparticles are also present. Fig. 4b depicts the particle size histogram of the nanoparticles and it can be seen from the histogram that the gold nanoparticles have sizes of 4–32 nm and possess an average size of ∼13.96 nm. A small difference is observed in the diameter value obtained from TEM and DLS measurement mainly due to the process involved in the sample preparation. The particle size determined by TEM represents the actual diameter of the nanoparticles as it is measured at the dry state of the sample, whereas the size measured by the laser light scattering method (DLS) is a hydrodynamic diameter (hydrated state); therefore, the nanoparticles will have a larger hydrodynamic volume due to solvent effects in the hydrated state.56 The crystallinity of the Au NPs is further evidenced by the selected area electron diffraction (SAED) pattern with bright circular spots, which is shown in Fig. 4c. The elemental composition analysis by energy dispersive spectroscopy (EDS) indicates the presence of gold atoms. The result shows a strong optical absorption peak at 2.3 keV, which is typical for the absorption of metallic Au NPs shown in Fig. 4d.
 |
| Fig. 4 (a) HRTEM images of Au NPs; (b) particle size distribution histogram of Au NPs determined from HRTEM micrograph prepared at room temperature; (c) the selected area electron diffraction (SAED) image of Au NPs; (d) EDX spectrum of AuNP from HRTEM. | |
X-ray diffraction (XRD) measurement
Fig. 5 shows the XRD patterns of air-dried Au NPs synthesized from aqueous Abelmoschus esculentus (L.) pulp extract. A number of Bragg reflections can be seen with 2θ values of 38.33°, 44.51°, 64.76°, and 77.8°, corresponding to the lattice planes, which may be indexed to the (111), (200), (220), and (311) facets of gold, respectively. Thus, the XRD pattern illustrates that the formed gold nanoparticles are crystalline in nature. The broadened peaks (full width at half maximum) indicate that the crystallite size of the gold nanoparticles lies in the range of nanometers. This is further determined by the calculation of average crystallite size from the Scherrer formula:
where d is the average crystallite size, λ is the wavelength of radiation, and β is the full width at the half maximum at diffraction angle θ. The calculated crystallite size for Au NPs is found to be ∼14.38 nm using this equation, which is close to the average particle size of ∼13.96 nm obtained from TEM analysis.
 |
| Fig. 5 XRD patterns showing peaks corresponding to the diffraction from (111), (200), (220), and (311) planes of fcc lattice of Au NPs. | |
Fourier transform infrared (FTIR) spectroscopy analysis
The different phytochemicals such as vitamins and proteins present in Abelmoschus esculentus (L.) pulp extract play an important role in the reduction of metal ions and stabilization of the nanoparticles. The FTIR spectra for Abelmoschus esculentus (L.) pulp extract reveal several characteristic bands as given in Fig. 6a. The peak at 3402 cm−1 corresponds almost entirely to the N–H stretching vibrations of the peptide linkages.57,58 The peak at 2934.5 cm−1 belongs to the stretching vibration of methyl groups.59 The peaks at 1741.1, 1427, 1631, and 1079.7 cm−1 are attributed to C
O stretching vibrations of cyclic esters from the vitamins,60,61 germinal methyl groups,62 amide I groups of proteins,58 and the bending vibration of C–OH groups and the anti-symmetric stretching band of C–O–C groups of polysaccharides and/or chlorophyll,63 respectively. The FTIR spectra for gold nanoparticles synthesized from Abelmoschus esculentus (L.) pulp extract is represented in Fig. 6b. The reasonable shifts in the peak positions from 3402, 2934.5, 1741.1, 1427, 1631 and 1079.7 cm−1 to 3411.89, 2921.32, 1730.9, 1635 and 1019.18 cm−1, respectively, of the synthesized Au NPs indicates that the different phytochemicals present in the Abelmoschus esculentus (L.) pulp extract are involved in reducing and stabilizing the nanoparticles.
 |
| Fig. 6 FTIR spectra of (a) pure Abelmoschus esculentus (L.) pulp extract and (b) extract stabilized Au NPs. | |
In vitro cell proliferation assay
PBL, Jurkat, K562 and DL cells were exposed to Au NPs at concentrations of 0, 1, 5, 10, 25 and 50 μg ml−1 for 24 h, and cytotoxicity was determined using the MTT assay (Fig. 7a). The results show that Au NPs up to the concentration of 50 μg/ml produce a significant reduction in the viability of Jurkat cells (P > 0.05 for each). The reduction in viability of Au NP-treated cancer cells occurs in a dose-dependent fashion. The Au NP-exposed Jurkat cell viability significantly decreased by 45.1%, 48.6%, 81.3% and 87.2% at 5, 10, 25 and 50 μg ml−1 doses. In the cases of K562 cells and DL cells, viability was significantly decreased by 38.38% and 50.165% and by 28.51% and 48.165% at 25 and 50 μg ml−1 doses, respectively. On the other hand, no significant reduction of PBL viability was noted with doses of Au NPs up to 25 μg ml−1. With a 50 μg ml−1 dose of Au NPs, PBLs viability was lost by 38.27%. This suggested that at higher doses (>25 μg ml−1) of Au NPs the cell specific toxicity is lost. From the above result, it is clearly revealed that Au NPs show maximal anticancer activity on Jurkat cells. Therefore, the remaining experiments were performed using these cells only. On the other hand, it was also noted that no significant loss of cell viability was observed for any dose of pulp extract used in the study (Fig. 7b). These findings clearly demonstrate the absence of any anticancer components in the extract and the killing of cancer cells can definitely be attributed to the Au NPs only.
 |
| Fig. 7 (a) In vitro cell proliferation assay of Au NPs treated PBL, Jurkat, K562, and DL cells. (b) Examination of cytotoxic effects of Abelmoschus esculentus (L.) pulp extract against Jurkat cells. Values are expressed as mean ± SEM of three experiments; * and # indicate significant differences (p < 0.05) compared to the control group. | |
The IC50 value of Au NPs against Jurkat cells was determined using the Statistica version 5.0 (Statsoft, India) software package. From this statistical calculation, it was found that the IC50 values of the Au NPs are 8.17 μg ml−1 and 68.18 μg ml−1 for Jurkat cells and PBLs, respectively. Because a 8.17 μg ml−1 concentration of Au NPs is found to be the IC50 and at this dose no significant loss of cell viability is observed for PBLs; therefore, further experiments were carried out using this concentration to see the effect of Au NPs against the leukemic cell line in vitro.
Cellular reactive oxygen species (ROS) level
Jurkat cells exposed to Au NPs for 24 h showed increased reactive oxygen species (ROS) formation as evidenced by the increase in fluorescence intensity of the DCF. We analyzed the results obtained by FACS by M2 and M1 percentage fluorescence variation. M1 was placed around the nonstressed Jurkat cells (viable). M2 was placed to the right of M1 to designate positive events. The % gated for M2 was 6.26% (viable Jurkat cells) and 81.50% (Jurkat cells treated with Au NPs). The results indicate that a significant (p < 0.05) elevation of ROS level is noted due to Au NP treatment (Fig. 8a and b). The fluorescence images (Fig. 8c and d) are highly correlated to the above results.
 |
| Fig. 8 Measurement of ROS levels in Au NPs pre-treated Jurkat cells by FACS analysis. DCF fluorescence intensity was expressed in term of ROS production. The cell population of M2 phase was noted as the ROS positive cells, whereas M1 denotes the viable/ROS negative cells. Here (a): untreated Jurkat Cells, (b): Jurkat cells treated with 8.17 μg ml−1 Au NPs. Fig. (c and d): Qualitative characterization of reactive oxygen species formation by H2DCFDA staining using fluorescence microscopy. Here, (c): untreated Jurkat cells, (d): Jurkat cells treated with 8.17 μg ml−1 Au NPs. | |
ROS are molecules and ions containing unpaired valence shell electrons, and being free radicals, they are highly active and have an important role in cell signaling, leading to oxidative cell damage.64 In physiologically active systems, ROS are produced intrinsically or extrinsically within the cell. Intracellular ROS generation occurs by a mitochondrial respiratory chain reaction and membrane-bound superoxide-generating enzyme i.e., nicotinamide adenine dinucleotide phosphate (NADPH) oxidase mediated reactions. Moreover, reduction of oxygen may either lead to H2O2 or OH˙ via dismutation and metal-catalyzed Fenton-like reactions, respectively.65–67 In our study, we found that Au NPs disrupted mitochondrial membrane integrity, as well as metabolic activity in Jurkat cells, confirmed by MTT assay and mitochondrial membrane potential measurements. Thus, in our present study, the probable cause of ROS generation is the impairment of mitochondrial electron transport chains and depletion of antioxidant defense systems (glutathione cycle). The involvement of ROS plays a great role in different types of anti-cancer research. Not only that, the involvement of ROS can be considered as an effective contribution of apoptosis in the etiology of cell death.68 Elevated levels of ROS stimulate the release of different pro-inflammatory markers, including TNF-α. This TNF-α activates nuclear factor-κB (NF-κB) and c-Jun N-terminal kinase (c-Jun NH2-terminal kinase, JNK), and ultimately leads to cell death by apoptotic and necrotic pathways.69
Alteration of mitochondrial membrane potential (MMP)
In our study, the mitochondrial membrane potential (MMP) was estimated in terms of rhodamine (Rh) 123 fluorescence intensity. Mitochondria are known to be involved in the process of programmed cell death; thus, we investigated the changes in mitochondrial membrane potentials in terms of Rh 123 fluorescence intensity. The results showed that the percentage of MMP decreased significantly (P < 0.05) in the Jurkat cell line with the increase of Au NP concentration, and reached 55.2% when treated with an IC50 dose (Fig. 9). The impairment of MMP in nanoparticle-treated Jurkat cells may be due to the malfunction of ATP synthesis and maintenance of ATP levels, leading to either apoptosis or necrosis. Cellular apoptosis does not depend only on depleted ATP synthesis; however, a certain drop in ATP levels may lead to apoptosis-induced cell death.70
 |
| Fig. 9 Measurement of mitochondrial membrane potential (MMP) Au NPs in pre-treated Jurkat cells. Values are expressed as mean ± SEM of three experiments; * indicates a significant difference (p < 0.05) compared to the control group. | |
EtBr-AO double staining cell morphological analysis
The results obtained from the EtBr-AO double staining are shown in Fig. 10. This staining revels that the viable cells with intact DNA and nuclei have a round and green nucleus. Early apoptotic cells have fragmented DNA, which appears as several green-colored nuclei. Late apoptotic and necrotic cell DNA is fragmented and stained orange and red.52 From the data, it is clear that Au NPs are able to decrease the number of viable cells tremendously. Most of the cells exhibit typical characteristics of apoptotic cells like plasma membrane blebbing and formation of apoptotic bodies. The number of cells stained orange increases significantly. A very small number of cells are stained red. These results indicate that most of the cells are not undergoing necrosis and cell death occurs primarily through apoptosis.
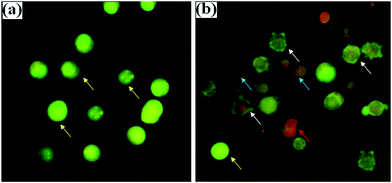 |
| Fig. 10 Apoptotic morphology study of Jurkat cells treated with Au NPs. Cells were treated with EtBr-AO and visualized under a fluorescence microscope. Here, (a): untreated Jurkat cells, (b): Jurkat cells treated with Au NPs. Yellow arrows indicate viable cells, white arrows indicate early apoptotic cells, blue arrows indicate late apoptotic cells and red arrows indicate necrotic cells. | |
Induction of caspase-3 activity and chromosome condensation by Au NPs
Caspase-3 plays a regulatory role in apoptotic cell death, which is induced after exposure to Au NPs (Fig. 11a). When Jurkat cells were treated with IC50 concentrations of Au NPs for 24 h, caspase-3 activity increased significantly compared to untreated cells. In addition to caspase-3 activity, chromatin condensation was also examined by DAPI staining. When Jurkat cells were treated with the above concentrations of Au NPs for 24 h, chromatin condensation and fragmentation was observed in the treated group (Fig. 11b and c). Caspase-3 activation and chromatin condensation/fragmentation in Jurkat cells suggest that Au NPs causes cell death by an apoptotic process.71
 |
| Fig. 11 Increase of chromosome condensation and caspase-3 activity in Jurkat cells after exposure of Au NPs for 24 h. (a) Caspase-3 activity; (b) control; (c) exposed to 8.17 μg ml−1 Au NPs. Values are expressed as mean ± SEM of three experiments; * indicates a significant difference (p < 0.05) compared to the control group. | |
Antimicrobial activity
The Au NP solution exhibits excellent antibacterial activity against the bacteria Bacillus subtilis, Bacillus cereus, Escherichia coli, Micrococcus luteus and Pseudomonas aeruginosa, showing a clearing zone around the holes with bacterial growth on petri plates by the cup plate method. The radial diameter of the inhibiting zones of Bacillus subtilis, Bacillus cereus, Escherichia coli, Micrococcus luteus and Pseudomonas aeruginosa are 26, 24, 15, 35 and 21 mm, respectively. The Au NPs at a concentration of 0.2 mg ml−1 show a range of specificity toward their antimicrobial activity (Fig. 12). Fig. 13 shows the clear inhibition zone made by Au NP solution against the strain of Bacillus subtilis only. The clear zone indicates bacterial growth restriction by diffused Au NP solution. At the same time, the control sets did not show any inhibition. The results are the mean of three separate experiments, each in triplicate. We have already discussed earlier in this manuscript that several gold containing salts show good antimicrobial activity. A high concentration of gold is harmful to both the consumer and the microbes; therefore, a smaller concentration (nano-range) is much more applicable for therapeutic purposes. This design of Au NP synthesis has great potential due to its antibacterial activity.
 |
| Fig. 12 Histogram showing the radial diameters of the inhibitory zones by Au NPs at the same concentration (0.2 mg ml−1) against different microorganisms. | |
 |
| Fig. 13 Antibacterial activity of Au NPs assayed by the agar diffusion method in petri plates. Au NPs poured in the circular well showed a zone of inhibition against Bacillus subtilis. The cavity of the Petri plate was filled with Au NP solution (0.2 mg ml−1). | |
Conclusions
We have described an eco-friendly and cost-effective protocol for synthesizing Au NPs using Abelmoschus esculentus (L.) pulp extract at room temperature. This extracellular synthesis of Au NPs makes these nanoparticles a potential candidate in various biomedical applications. It may be inferred that the phytochemicals present in the Abelmoschus esculentus (L.) pulp extract are responsible for capping the Au NPs as evidenced from FTIR studies. The Au NPs have an average size of ∼14 nm and show sufficient stability and crystallinity. The results addressed herein disclose the anticancer activity of green synthesized Au NPs. It is reasonable to infer that the developed Au NPs show potent anti-proliferative efficacy against the Jurkat cell line. Elevation of ROS and disruption of mitochondrial membrane potential in Au NP-exposed Jurkat cells suggests the possible contribution of apoptosis in the etiology of cell death. The involvement of apoptosis rather than necrosis was confirmed by the EtBr-AO double staining method. These Au NPs have great promise as antimicrobial agents. The application of Au NPs based on these findings may lead to valuable discoveries in various applications such as medical devices and antimicrobial agents.
Acknowledgements
The authors Md. M. R. Mollick gratefully acknowledge DST, Govt. of India, for providing fellowship under INSPIRE fellowship. B. Bhowmick wishes to thank the Council of Scientific & Industrial Research (CSIR) Project [Vide letter no. 02 (0077)/12/EMR-II dated 01.11.2012] and D. Mondal likes to thank CSIR, New Delhi, for his fellowship. We also acknowledge the Center for Research in Nanoscience and Nanotechnology (CRNN), University of Calcutta, for instrumental facilities.
Notes and references
- R. Joerger, T. Klaus and C. G. Granqvist, Adv. Mater., 2000, 12, 407 CrossRef CAS.
- D. Maity, M. M. R. Mollick, D. Mondal, B. Bhowmick, M. K. Bain, K. Bankura, J. Sarkar, K. Acharya and D. Chattopadhyay, Carbohydr. Polym., 2012, 90, 1818 CrossRef CAS PubMed.
- M. M. R. Mollick, B. Bhowmick, D. Maity, D. Mondal, I. Roy, J. Sarkar, D. Rana, K. Acharya, S. Chattopadhyay and D. Chattopadhyay, Microfluid. Nanofluid., 2014, 16, 541 CrossRef CAS.
- J. Li, C. Wu, Y. Dai, R. Zhang, X. Wang, D. Fu and B. Chen, J. Nanosci. Nanotechnol., 2007, 7, 435 CAS.
- N. R. Jana, T. K. Sau and T. Pal, J. Phys. Chem. B, 1999, 103, 115 CrossRef CAS.
- C. Liu, X. Yang, H. Yuan, Z. Zhou and D. Xiao, Sensors, 2007, 7, 708 CrossRef CAS PubMed.
- A. M. Schrand, L. K. Braydich-Stolle, J. J. Schlager, L. Dai and S. M. Hussain, Nanotechnology, 2008, 19, 235104 CrossRef PubMed.
- R. Elghanian, J. J. Storhoff, R. C. Mucic, R. L. Letsinger and C. A. Mirkin, Science, 1997, 277, 1078 CrossRef CAS.
- L. N. Ng, B. J. Luff, M. N. Zervas and J. S. Wilkinson, Opt. Commun., 2002, 208, 117 CrossRef CAS.
- J. Li, C. Ma, X. Xu, J. Yu, Z. Hao and S. Qiao, Environ. Sci. Technol., 2008, 42, 8947 CrossRef CAS.
- W. Baschong and N. G. Wrigley, J. Electron Microsc. Tech., 1990, 14, 313 CrossRef CAS PubMed.
- J. F. Hainfeld, D. N. Slatkin, T. M. Focella and H. M. Smilowitz, Br. J. Radiol., 2006, 79, 248 CrossRef CAS PubMed.
- C. Alric, J. Taleb, G. Le Duc, C. Mandon, C. Billotey, A. Le Meur-Herland, F. Vocanson, M. Janier, P. Perriat, S. Roux and O. Tillement, J. Am. Chem. Soc., 2008, 130, 5908 CrossRef CAS PubMed.
- X. Huang, I. H. El-Sayed, W. Qian and M. A. El-Sayed, J. Am. Chem. Soc., 2006, 128, 2115 CrossRef CAS PubMed.
- W. Cai, T. Gao, H. Hong and J. Sun, Nanotechnol., Sci. Appl., 2008, 1, 17 CAS.
- A. A. Umar and M. Oyama, Cryst. Growth Des., 2009, 9, 1146 Search PubMed.
- J. Kim, S. Cha, K. Shin, J. Y. Jho and J. C. Lee, Adv. Mater., 2004, 16, 459 CrossRef CAS PubMed.
- T. H. Ha, H.-J. Koo and B. H. Chung, J. Phys. Chem. C, 2007, 111, 1123 CAS.
- A. Rai, A. Singh, A. Ahmad and M. Sastry, Langmuir, 2006, 22, 736 CrossRef CAS PubMed.
- S. S. Shankar, A. Rai, A. Ahmad and M. Sastry, Chem. Mater., 2005, 17, 566 CrossRef CAS.
- S. S. Shankar, A. Rai, B. Ankamwar, A. Singh, A. Ahmad and M. Sastry, Nat. Mater., 2004, 3, 482 CrossRef CAS PubMed.
- M. Spuch-Calvar, J. Pacifico, J. Pérez-Juste and L. M. Liz-Marzán, Langmuir, 2008, 24, 9675 CrossRef CAS PubMed.
- T.-H. Lin, N. C. Linn, L. Tarajano, B. Jiang and P. Jiang, J. Phys. Chem., 2009, 113, 1367 CrossRef CAS PubMed.
- C. R. Mihail, Curr. Opin. Biotechnol., 2003, 14, 337 CrossRef.
- J. L. Gardea-Torresdey, J. G. Parsons, K. Dokken, J. Peralta-Videa, H. E. Troiani, P. Santiago and M. Jose-Yacaman, Nano Lett., 2002, 2, 397 CrossRef CAS.
- J. L. Gardea-Torresdey, E. Gomez, J. Peralta-Videa, J. G. Parsons, H. E. Troiani and M. Jose-Yacaman, Langmuir, 2003, 19, 1357 CrossRef CAS.
- B. Nair and T. Pradeep, Cryst. Growth Des., 2002, 2, 293 CAS.
- M. M. R. Mollick, B. Bhowmick, D. Maity, D. Mondal, M. K. Bain, K. Bankura, J. Sarkar, D. Rana, K. Acharya and D. Chattopadhyay, Int. J. Green Nanotechnol., 2012, 4, 230 CrossRef CAS.
- Y. Konishi, K. Ohno, N. Saitoh, T. Nomura and S. Nagamine, Trans. Mater. Res. Soc. Jpn., 2004, 29, 2341 CAS.
- A. Ahmad, S. Senapati, M. I. Khan, R. Ramani, V. Srinivas and M. Sastry, Nanotechnology, 2003, 14, 824 CrossRef CAS.
- A. Ahmad, P. Mukherjee, S. Senapati, D. Mandal, M. I. Khan, R. Kumar and M. Sastry, Colloids Surf., B, 2003, 28, 313 CrossRef CAS.
- S. S. Shankar, A. Rai, A. Ahmad and M. Sastry, J. Colloid Interface Sci., 2004, 275, 496 CrossRef CAS PubMed.
- S. P. Chandran, M. Chaudhary, R. Pasricha, A. Ahmad and M. Sastry, Biotechnol. Prog., 2006, 22, 577 CrossRef CAS PubMed.
- J. Huang, L. Lin, Q. Li, D. Sun, Y. Wang, Y. Lu, N. He, K. Yang, X. Yang, H. Wang, W. Wang and W. Lin, Ind. Eng. Chem. Res., 2008, 47, 6081 CrossRef CAS.
- D. Maity, M. K. Bain, B. Bhowmick, J. Sarkar, S. Saha, K. Acharya, M. Chakraborty and D. Chattopadhyay, J. Appl. Polym. Sci., 2011, 122, 2189 CrossRef CAS PubMed.
- K. P. Bankura, D. Maity, M. M. R. Mollick, D. Mondal, B. Bhowmick, M. K. Bain, A. Chakraborty, J. Sarkar, K. Acharya and D. Chattopadhyay, Carbohydr. Polym., 2012, 89, 1159 CrossRef CAS PubMed.
- Y. A. Krutyakov, A. A. Kudrynskiy, A. Y. Olenin and G. V. Lisichkin, Russ. Chem. Rev., 2008, 77, 233 CrossRef CAS PubMed.
- J. F. Hernandez-Sierra, F. Ruiz, D. C. C. Pena, F. Martinez-Gutierrez, A. E. Martinez, A. J. P. Guillen, H. Tapia-Perez and G. M. Castanon, Nanomedicine: Nanotechnology, Biology and Medicine, 2008, 4, 237 CrossRef CAS PubMed.
- P. Prema and S. Thangapandiyan, Int. J. Pharm. Pharm. Sci., 2013, 5, 310 CAS.
- M. F. Zawrah, I. Sherein and A. B. D. El-Moez, Life Sci. J., 2011, 8, 37 Search PubMed.
- D. MubarakAli, N. Thajuddin, K. Jeganathan and M. Gunasekaran, Colloids Surf., B, 2011, 85, 360 CrossRef CAS PubMed.
- A. Mishra, S. K. Tripathy and S. Yun, J. Nanosci. Nanotechnol., 2011, 11, 243 CrossRef CAS PubMed.
- D. M. Parkin, Lancet Oncol., 2001, 2, 533 CrossRef CAS.
- S. Jain, D. G. Hirst and J. M. O'Sullivan, Br. J. Radiol., 2012, 85, 101 CrossRef CAS PubMed.
- A. Chompoosor, K. Saha, P. S. Ghosh, D. J. Macarthy, O. R. Miranda, Z. J. Zhu, K. F. Arcaro and V. M. Rotello, Small, 2010, 6, 2246 CrossRef CAS PubMed.
- M. K. K. Oo, Y. Yang, Y. Hu, M. Gomez, H. Du and H. Wang, ACS Nano, 2012, 6, 1939 CrossRef PubMed.
- D. D. Perrin, W. L. F. Armarigo and D. R. Perrin, in Purification of Laboratory Chemicals, Pergamon Press, Oxford, 2nd edn, 1980 Search PubMed.
- L. Hudson and F. C. Hay, in Practical Immunology, Blackwell Pub., Oxford, 3rd edn, 1989 Search PubMed.
- S. Chattopadhyay, S. P. Chakraborty, D. Laha, R. Bara, P. Pramanik and S. Roy, Cancer Nanotechnol., 2012, 3, 13 CrossRef CAS.
- S. K. Dash, S. Chattopadhyay, T. Ghosh, S. Tripathy, S. Das, D. Das and S. Roy, ISRN Oncol., 2013, 2013, 709269 Search PubMed.
- A. Roy, A. Ganguly, S. BoseDasgupta, B. D. Benu, C. Pal, P. Jaisankar and H. K. Majumder, Mol. Pharmacol., 2008, 74, 1292 CrossRef CAS PubMed.
- K. Ho, L. S. Yazan, N. Ismail and M. Ismail, Cancer Epidemiol., 2009, 33, 155 CrossRef CAS PubMed.
- C. C. Lin, S. T. Kao, G. W. Chen, H. C. Ho and J. G. Chung, Anticancer Res., 2006, 26, 227 CAS.
- P. Mulvaney, Langmuir, 1996, 12, 788 CrossRef CAS.
- A. Khan, A. M. El-Toni, S. Alrokayan, M. Alsalhi, M. Alhoshan and A. S. Aldwayyan, Colloids Surf., A, 2011, 377, 356 CrossRef CAS PubMed.
- F.-P. Gao, H.-Z. Zhang, L.-R. Liu, Y.-S. Wang, Q. Jiang, X.-D. Yang and Q.-Q. Zhang, Carbohydr. Polym., 2008, 71, 606 CrossRef CAS PubMed.
- P. Kannan and S. A. John, Nanotechnology, 2008, 19, 085602 CrossRef PubMed.
- S. C. Whiteman, Y. Yang, J. M. Jones and M. A. Spiteri, Ther. Adv. Respir. Dis., 2008, 2, 23 CrossRef CAS PubMed.
- S. Li, Y. Shen, A. Xie, X. Yu, L. Qiu, L. Zhang and Q. Zhang, Green Chem., 2007, 9, 852 RSC.
- H. Bar, D. K. Bhui, G. P. Sahoo, P. Sarkar, S. Pyne and A. Misra, Colloids Surf., A, 2009, 348, 212 CrossRef CAS PubMed.
- L. B. Luo, S. H. Yu, H. S. Qian and T. Zhou, J. Am. Chem. Soc., 2005, 127, 2822 CrossRef CAS PubMed.
- A. Tripathy, A. M. Raichur, N. Chandrasekaran, T. C. Prathna and A. Mukherjee, J. Nanopart. Res., 2010, 12, 237 CrossRef CAS.
- N. Jain, A. Bhargava, S. Majumdar, J. C. Tarafdar and J. Panwar, Nanoscale, 2011, 3, 635 RSC.
- K. J. Davies, IUBMB Life, 2000, 50, 241 CrossRef CAS.
- V. Vallyathan and X. Shi, Environ. Health Perspect., 1997, 105, 165 CrossRef CAS.
- V. J. Thannickal and B. L. Fanburg, Am. J. Physiol., 2000, 279, L1005 CAS.
- D. V. Pereira, F. Petronilho, H. R. Pereira, F. Vuolo, F. Mina, J. C. Possato, M. F. Vitto, D. R. de Souza, L. da Silva, M. M. da Silva Paula, C. T. de Souza and F. Dal-Pizzol, Invest. Ophthalmol. Visual Sci., 2012, 53, 8036 CAS.
- M. Jeyaraj, M. Rajesh, R. Arun, D. MubarakAli, G. Sathishkumar, G. Sivanandhan, G. K. Dev, M. Manickavasagam, K. Premkumar, N. Thajuddin and A. Ganapathi, Colloids Surf., B, 2013, 102, 708 CrossRef CAS PubMed.
- H. M. Shen and S. Pervaiz, FASEB J., 2006, 20, 1589 CrossRef CAS PubMed.
- C. Adrie, M. Bachelet, M. Vayssier-Taussat, F. Russo-Marie, I. Bouchaert, M. Adib-Conquy, J. M. Cavaillon, M. R. Pinsky, J. R. Dhainaut and B. S. Polla, Am. J. Respir. Crit. Care Med., 2001, 164, 389 CrossRef CAS PubMed.
- R. U. Janicke, M. L. Sprengart, M. R. Wati and A. G. Porter, J. Biol. Chem., 1998, 273, 9357 CrossRef CAS PubMed.
|
This journal is © The Royal Society of Chemistry 2014 |
Click here to see how this site uses Cookies. View our privacy policy here.