DOI:
10.1039/C4RA06535B
(Paper)
RSC Adv., 2014,
4, 39948-39954
Carbon nanoparticles in ‘biochar’ boost wheat (Triticum aestivum) plant growth
Received
2nd July 2014
, Accepted 7th August 2014
First published on 7th August 2014
Abstract
Biochar is shown to contain raw carbon nanoparticles (rCNPs). The traditional use of biochar for the healthy growth of plants is due to its retention capability of nutrients for needs-based slow release. On aging, the rCNPs are aerially oxidized with the incorporation of hydrophilic carboxylic and hydroxyl groups on the biochar surface to increase its spongy structure that enhances its absorptivity to water and several ionic nutrients. The chemical oxidation of rCNPs in biochar introduces similar carboxylic acid and hydroxyl groups in transforming rCNPs to water soluble carbon nanoparticles (wsCNPs). These wsCNPs in solution enhance the growth rate of wheat plants. The concentration of wsCNPs used in this wheat plant growth study varied in the range from 10 to 150 mg L−1. The seeds treated with wsCNPs in this range showed higher growth rates, with the optimum growth found to occur with 50 mg L−1 wsCNPs, as compared with the control study. This suggests a concentration threshold of wsCNPs for the optimum stimulation of plant growth that the aged biochar can readily meet. Model experiments with ammonium nitrate as cationic and anionic nutrients show that both the rCNPs and wsCNPs hold the nutrient ions, although the negatively charged wsCNPs hold less effectively than the anionic nitrate. These carbon nanoparticles are capable of releasing cationic and anionic nutrients slowly over time. Such action is correlated with the booster action of CNPs in supplying nutrients to the young plants. Thus, wsCNPs may be a better choice than fertiliser or manure alone, due to the controlled and slow release of nutrients for better assimilation by plants.
1. Introduction
Carbon is one of the most key elements involved in fostering life. In addition to its existence in organic compounds responsible in biochemical processes, elemental carbon in its newer nano version, excluding coal, graphite or diamond, has gained added importance in several applications.1 The story of such carbon is related with some spectacular discoveries but also with issues such as carbon balance to global warming and climate change.2
Biochar‡ is a carbonized form of leftover biomass (waste stems and roots) in agricultural land, obtained by its pyrolysis under limited air. This is mixed with the soil during ploughing of the land for the next crop to enhance the fertility of the land. Smaller particles of carbon in the nano range are formed during biochar synthesis. These particles are called biochar dust, due to their smaller sizes.3a The land of the Amazon basin ‘terra preta’ is highly fertile owing to the high quantity of carbon as biochar, formed by carbonization processes over a long period of time.3 The carbon in biochar does not degrade readily and stays in the soil for several years. However, on aging, the defects on the surface of the carbon in biochar responds to a slow aerial oxidation, with a resulting peripheral incorporation of hydroxyl and carboxylic groups.3d The highly porous carbon in biochar retains micro nutrients for controlled release, which is necessary for germination and growth of the plants.3 It can adsorb water up to 4.5 times its dry weight, due to its porous structure, which mitigates the drought effect.4 Biochar applied in the soil has been shown to retain these promoting effects when tested for a short period of 2 years3c,d to longer periods, even up to a hundred years of observation in ‘terra preta’ of the Amazon basin soil.3b Biochar in its crushed form increases its surface area, which in turn enhances its effectiveness.3d Interestingly in soil, the slow aerial oxidation of biochar on its surface helps to enhance its ion exchange and nutrients retaining capacity.3d,5a Biochar is also well known to increase the colonization of mycorrhizal, rhizobia.3c It is known that graphene units with heteroatoms (N, O) are one of the main structural entities of the biochar surface and are responsible for nutrients adsorption.3a,5b These graphene units curve to create spherical-like carbon particles under thermal conditions.5c–g The pyrolysis of carbonaceous materials thus first generates graphene nucleation from the pentagonal ring, followed by a spiral shell growth, with the subsequent incorporation of pentagonal, hexagonal and heptagonal rings to facilitate carbon nanoparticles growth.5c–g
The effect of nanocarbons in plant growth is relatively less explored compared with similar studies with the animal kingdom.6 Though specific uses of such carbon nanomaterials have been carried out on different plant species, including rice,6a gram,6b–c tobacco,6d–e and wheat.6f Earlier reports show that single-walled carbon nanotubes (SWCNTs), multi-walled carbon nanotubes (MWNTs), and carbon nano-onions (CNOs) readily penetrate the biological membrane barriers in mammals,7a plants,6d and also in microorganisms.7b It is also shown that water soluble carbon nanotubes (wsCNTs) become aligned due to an endo-osmotic root pressure in the xylem vessels of plants, which then enhances the water and nutrients uptake capacity.6b,8 In the presence of carbon nanotubes (CNTs), lignin biosynthesis9 suggests the formation of more biomass of xylem vessels than is shown to be directly related to the growth of the overall plant, in relation to the biochar effect.3 The essential nutrients required for a plant interact with the hydrophilic groups attached to the surface of the carbon nanomaterials by hydrogen bonds and by electrostatic interaction in the outer peripheries of CNPs6 and remain attached there on a temporal basis, and thus these carbon nanomaterials work as storage houses for micronutrients. Such retention then allows a sustained and slow release of these for the facile transport of the essential nutrients inside the xylem vessels,6 which is similar to retaining the activity of biochar.3d,5
The present work deals with the isolation of carbonaceous materials from biochar as raw carbon nanoparticles (rCNPs). As these are not effectively dispersible in water, the isolation of water soluble carbon nanoparticles (wsCNPs) from the rCNPs of biochar was carried out to compare its concentration dependent effect in the growth of wheat (Triticum aestivum) plant. We choose wheat for this study as it is the most important grain used as a staple food throughout the world. Furthermore, we show here that the surface groups on either rCNPs or wsCNPs play an important role in determining the nature of the effectively adsorbed ions by using nitrogen containing essential nutrient ions. These adsorbed ions are subsequently released slowly, in relation to the nutrient needs in the growth of young plants.
2. Experiment
2.1 Materials
All chemical reagents were of analytical grade and used without further purification. Water used in the control experiments, as well as for wsCNPs, to make the solutions was simple tap water to mimic the environmental conditions otherwise mentioned. The tap water quality parameters are known.†
2.2 Synthesis of water soluble carbon nanoparticles
We collected ‘biochar’ material produced by carbonization of the plant waste left in rice agriculture fields used in the present study. The ‘biochar’ collected was powdered and the fine powdered part was separated from the unburned parts by sieving. The powdered material was treated with water and stirred and then the stirring was stopped, whereby the heavier part containing silica and the other solid inorganic materials settled down fast, leaving the upper part containing floating carbon particles, which were decanted off. Repetition of this floatation procedure resulted in the separation of the essential carbon materials in almost pure form from the silica and mud. The carbon part was then filtered through a filter paper and washed twice with water to remove any residual soluble salts present therein. The carbon residue was then dried at 60 °C for 5 hours. This carbon is termed as raw carbon nanoparticles (rCNPs). The dried carbon material was treated with HNO3 and water (in a 1
:
1 ratio), for oxidation to produce the water soluble version as reported earlier.10,6b,c,11 The final isolated and dried water soluble carbon powder was used in the solution study and termed as wsCNPs.
2.3 Surface disinfestation of seeds
The surface disinfestation of seeds was carried out by their immersion in 10% sodium hypochlorite solution for 10 min followed by washing three times with double-distilled water, and then subsequently soaked in tap water† for control and in wsCNPs solution of different concentrations for 6 h before use.6b,c,f
2.4 Preparation of wsCNPs solution and germination
For the optimization, different concentration (10, 20, 40, 50, 80, 100 and 150 mg L−1) of wsCNPs were prepared as stock solution and used in comparison with the control (without wsCNPs). Though the dissolved wsCNPs remained in solution for an extended period of time, even then each solution was shaken well before use. For germination, the wsCNPs-treated wheat seeds were placed in cotton clothes of one square feet size soaked with the respective concentration of wsCNPs and, for control, under water for comparison. Cotton clothes were wetted using the same volume of respective solutions. Seeds covered with such wet cotton clothes were placed in polystyrene plates during the germination period.
2.5 Treatment of soil
Natural soil was taken and dried at 60 °C for 10 h to remove any excess water from it and any volatile substances, if present. Then, it was allowed to cool to attain room temperature. The weighed amount of soil placed in each earthen pot was fixed to culture the wheat samplings under similar conditions after germination under moist clothes.
2.6 Wheat plant growth
On the 5th day, the germinated wheat seeds were transferred into the soil and placed in different earthen pots with the germinated seeds placed under the same depth of the soil in each pot, and then allowed to grow in an open environment.
2.7 Characterization
The surface morphologies of rCNPs and wsCNPs were imaged using a field emission scanning electron microscope (FESEM), which is a SUPRA 40VP (Carl Zeiss NTS GmbH, Oberkochen, Germany) in high-vacuum mode operated at 5 kV. Transmission electron microscopy (TEM) analysis was performed using a Tecnai 20 D456 U-Twin Gun W, HT 200 kV. 400 mesh size carbon coated copper grid procured from Electron Microscopy Sciences, Hatfield, PA, was used for the TEM study. FT-IR spectra were recorded with a Bruker Vertex 70 FT-IR spectrophotometer as pressed KBr pellets. The samples were prepared using standard protocols.
2.8 NH4+ and NO3− ions release study
Nitrogen containing ions are some of the main ingredients of fertilizer and these essential nutrients are required for plant growth and were thus chosen for this study. Nitrogen deficiency leads to chlorosis (yellowing) of plant leaves, due to the drop in chlorophyll content. In a typical loading procedure, 200 mg of CNPs (both versions) was packed in a glass column of inner diameter 3 mm over a glass wool support. 20 mL of 10−4 M NH4NO3 solution was poured gently in to the top of the column and was left undisturbed to attain equilibrium for 12 h. The solution was then eluted through the column with an appropriate flow rate of 0.25 mL min−1. This first recovered elute was tested for NH4+ by Nessler's reagent12 and NO3− ion by Griess–Ilosvay reagent under reduction with Zn dust.10 The un-adsorbed NH4+/NO3− ions were quantified by using a JASCO V-530 UV-Vis spectrophotometer, following standard spectrophotometric methods of analyses. The column was then quickly washed with a portion of chilled water (15 mL), and then dried at 60 °C under a current of N2 gas through the column.
To understand the subsequent time-bound NH4+ and NO3− ion release, distilled water was added in the top of the column and it was eluted with a fixed flow rate (0.25 mL min−1) at intervals of 24, 72, 120, 168 and 240 h. A fixed volume of the elute in each of these time periods was taken and the concentration of NH4+ and NO3− ions released were spectrophotmetrically evaluated using the Nessler test12b and Griess–Ilosvay reagent with Zn dust.10 Similar experimental procedures were followed for both the rCNPs and wsCNPs forms.
3. Results and discussions
The carbon separated from the mud part from biochar, rCNPs, was imaged by FESEM and TEM microscopy and is shown in Fig. 1a and b. Once this is derivatized, the solubility pattern of wsCNPs can be seen, as in Fig. 3. The surface visualizations of wsCNPs were achieved by FESEM and TEM as shown in Fig. 1c and d. The nearly spherical morphologies of wsCNPs are confirmed by TEM (Fig. 1d). High resolution transmission electron microscopy (HRTEM) shows the multiwall, porous nature of wsCNPs (Fig. 3) and thus these can best be described as carbon nano-onions.6c FESEM and TEM clearly show the presence of carbon nanoparticles in the size ranges from 20 to 50 nm (histogram not shown). These wsCNPs contain an average of 20% of carboxylic groups attached (by weight), as found by acid–base titration.13 Extensive surface impregnation of the carboxylic acids and other enol groups make the outer surface of these wsCNPs porous. Initially, to dissolve this in water, sonication for a few minutes is essential. These wsCNPs remain in solution for a minimum period of 30 ± 5 days in water (Fig. 2).
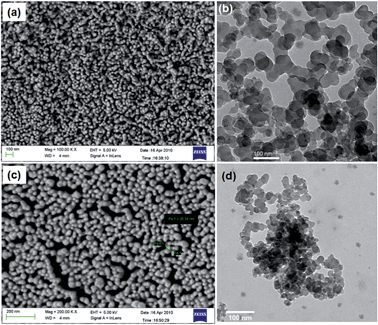 |
| Fig. 1 FESEM and TEM images of biochar: (a and b) rCNPs; (c and d) wsCNPs. | |
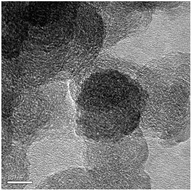 |
| Fig. 2 HRTEM image of wsCNPs. | |
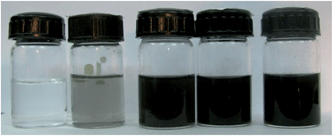 |
| Fig. 3 From left to right: control, 10, 50, 80 and 150 mg L−1 solutions of wsCNPs in water. | |
The FTIR of rCNPs and wsCNPs are shown in Fig. 4, which shows that these retain almost similar functional groups. The FTIR of rCNPs and wsCNPs show peaks around 1700 and ∼3420 cm−1, corresponding to the vibration due to the presence of >C
O and –OH groups, respectively. As expected for rCNPs, the carboxyl stretching vibration (appearing only as the shoulder) is related to the low abundance of carboxylate groups compared to that in wsCNPs, where the peak at 1717 cm−1 becomes prominent. The prominent peak around 1100 cm−1 is responsible for the presence of –C–O(H) vibrations and originates from hydroxyl groups attached in both the forms. This confirms the presence of hydrophilic groups on the surface of rCNPs3d,5a introduced by ageing and, for wsCNPs, the density of carboxylation is increased due to the oxidative treatment to enhance its solubility in water.
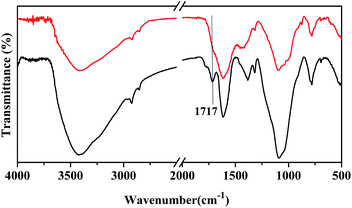 |
| Fig. 4 FTIR of rCNPs (red) and wsCNPs (black). | |
It was found that wsCNPs-exposed wheat seeds germinate faster than the control, which is consistent with previous reports.6 The optical images of germinated seeds for different sets of wsCNPs and control are shown in Fig. 5. After transferring the germinated seeds into the soil, we monitored the length of the shoot part of the wheat plants in every three day intervals. The shoot length of the control and wsCNPs-treated plants at intervals are graphically represented in Fig. 6. The shoot lengths of wheat plants increase in a proportional manner with 10–50 mg L−1 concentration of wsCNPs. When 80 mg L−1 of wsCNPs was used, the growth of the shoot length slightly decreased compared to that achieved under 50 mg L−1 treated wsCNPs. However, this is higher than that for 40 mg L−1 treated wsCNPs. The percentage shoot growths of wsCNPs-treated seeds with respect to the control seeds were calculated with the standard equation, and the results obtained are presented in Fig. 7.
where
Lcontrol and
LwsCNPs are the shoot lengths for the control and wsCNPs-treated seeds, respectively.
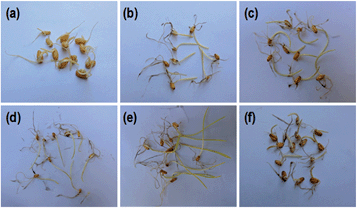 |
| Fig. 5 Germinated seeds (a) control; with wsCNPs of concentration (b) 10 mg L−1; (c) 20 mg L−1; (d) 40 mg L−1; (e) 50 mg L−1; and (f) 150 mg L. (These are not imaged from the same distance and the figures compare only the variation of the shoots in the germination). | |
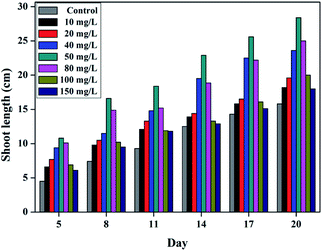 |
| Fig. 6 The growth of the control and wsCNPs-treated wheat plants. | |
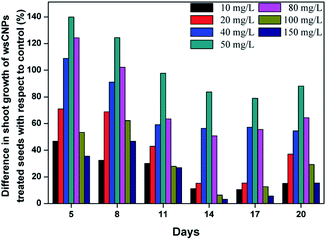 |
| Fig. 7 The growth difference in the shoot length of wsCNPs-treated and the control seeds with respect to the control seeds (in %). | |
Fig. 5–7 confirm that the growth of the shoot part of the wheat plants increase in the presence of wsCNPs compared to that with the control. The shoot lengths of wsCNPs-treated wheat plants are significantly longer compared with the control in the concentration range of 10–80 mg L−1. In the range of 100–150 mg L−1 of wsCNPs, the growth remains similar to the seeds treated with 10 mg L−1 of wsCNPs (Fig. 6).
These results clearly indicate that the growth rate of the wheat plants treated with 50 mg L−1 is optimum in comparison with the growth obtained from the other concentration of the wsCNPs used. The growth rate of wheat plant exposed with an 80 mg L−1 concentration of wsCNPs lies in between those found under exposure with 40 and 50 mg L−1 (Fig. 6 and 7). Therefore, the optimum concentration of wsCNPs as a growth stimulator may lie in the range of 40–80 mg L−1, and preferably around 50 mg L−1. Moreover, it has been shown earlier that the carbon nanomaterials enhance the overall growth of the plants.3c,d,6 If transport and the release of micronutrients is the exclusive task of this growth simulator, then it may be that going beyond the threshold, an excess supply of mineral nutrients may be deleterious to the plant and may retard its growth. It is to be noted that these carbon nanomaterials remain in the root section of the plant and do not travel to the higher shoot part, and thus the possibility for the distribution of these carbon nanomaterials in the products from such treated crops may not be there.6e,f It is true that no apparent distinction between the grains obtained using biochar or without its use can be made. The consumption of crops under biochar treatment over an extended period for several centuries do not show any ill effects due to the generation of human habituation with the consumption of such products. We have recently shown that the human race has achieved immunity from nanocarbon materials by the involuntary intake of such products with cooked food for centuries.14 As the use of biochar is an age old practice, so any distribution of such wsCNPs/rCNPs inside seeds or fruits on consumption may have developed immunities in humans.
The average size of root cells is roughly 1000 times larger than the diameter of wsCNPs (20–50 nm). These wsCNPs/rCNPs are known to get into plant roots and finally become embedded into the xylem vessels, as previously shown by us using electron microscopic studies.6c,d The porous nanoparticles hold the nutrients and are the active constituents of biochar,3d,5 which we show slowly release the mineral nutrients like ammonium and nitrate ions. The presence of carbon nanomaterials inside the xylem vessels enhances the water uptake and transportation, by a channel process or by adsorption process.6b
With the higher concentration of wsCNPs, the decrease in the growth and growth rate of wheat plants (Fig. 6 and 7) is an important inference, and which indicates that the optimized concentration of wsCNPs should be used in relation to their application as growth promoters. At higher concentrations of wsCNPs, the easy release of micro/macronutrients into the root site accumulate and increase in quantity, which may have a deleterious effect on the plant growth. Thus the rCNPs which cause biochar to be much less soluble in water have an advantage over wsCNPs, so far as their intimate association with the root of the plant. Therefore, biochar has the optimum capability to supply nutrients for the growth of plant. The difference between crops using fertilizers and manures based on biochar farming may be addressed in the following context. Biochar, by holding essential nutrients, slowly releases these need-based nutrients to a plant for its normal healthy growth in contrast to fertilizer treatment, where fertilizers rapidly release these mineral nutrients and can cause cytotoxicity in a plant, resulting in senescence. Thus, the sprinkling of fertilizer could supply such nutrients for growth access at the initial stages but could ultimately cause death of the plant. Frequently, excess watering is done in the field to wash away the local excess concentration of the fertilizer after a few days of its sprinkling, resulting in draining out of these essential nutrients and their percolating through the top soil layers and accumulating underground. To avoid this happening, expensive fertilizers are nowadays available that are wrapped in bioaccessible synthetic polymers that slowly releases micronutrients, similar to what one can simply achieve using biochar.
It is known that the dehydrogenase activity is associated with the ability of roots to retain nutrients and water, which is dependent on the concentration of these carbon nanomaterials.15 The decrease in dehydrogenase activity with higher concentrations of carbon nanomaterials has also been reported.6f However, in contrast to the previous report,6f we have observed the enhancement in the shoot length of the wheat plants. In the present case, plant roots are allowed to penetrate the soil layers to grow under normal conditions, which remained absent in the case study under the artificial growth media.6f In addition, it is expected that soil provides the optimum environment for the growth of the roots. It has also been shown that the root growth is affected in artificial growth media, causing artefact responses.16
3.1 NH4+/NO3− ions release study
Fertilizers like NH4NO3 provide NH4+ and NO3− ions to the plants as essential nutrients for their growth. These ions enter through the xylem vessels of plants. CNPs, due to their much smaller sizes, enter into the xylem vessels and help the plant by adsorbing essential ions and water.6b,c Interestingly, such ions are also adsorbed by biochar present in the soils. The carbon nanomaterials in the xylem vessels have electrostatic interactions with the essential ions, and this thus leads to adsorption on the surface.3,6b,c,f In a typical experiment, the slow release of adsorbed NH4+ and NO3− from rCNPs and wsCNPs are shown in Fig. 8. It is interesting to note that the surface groups play diverse roles in the ions being absorbed by these CNPs. The rCNPs, with very limited aerial oxidized surfaces, adsorb both the ions, as shown in Fig. 8. In the case of wsCNPs, where the surface is highly negatively charged due to the presence of more carboxylic acid and hydroxyl groups, NH4+ ions are adsorbed preferably over NO3− ion by wsCNPs (Fig. 8) as compared to r-CNPs. The chemical complexity of wsCNPs may allow adsorbing NO3− ions, although to a much lesser extent compared to that with the adsorption of rCNPs. Interestingly, its adsorption capability has been judged qualitatively for several other cations like potassium, calcium, and nickel as noteworthy, as all these are essential mineral nutrients known for plant growth. Thus, biochar can help to serve several other mineral nutrients needed for the healthy growth of a plant. Such adsorption and desorption of NH4+ and NO3− may be similar for other ions, albeit the rates may differ, and may even continue in cycles, as biochar is stable for years and only slowly is oxidised by air on its surface.3c,d,5 Therefore, our investigation confirms that, on aging, the aerial oxidation of biochar may function like wsCNPs as described herein. The role of biochar is even more important to trap both the anionic and cationic forms of nutrient ions, followed by their slow delivery as required by the plant for growth.3,6b,c,f
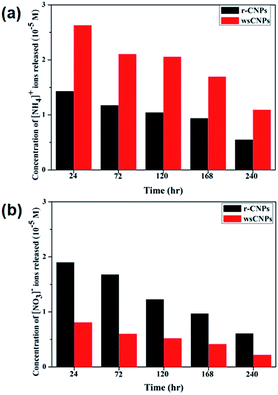 |
| Fig. 8 Release of adsorbed ions from rCNPs and wsCNPs at different time intervals: (a) NH4+; (b) NO3− ions. | |
4. Conclusions
The present work is intended to understand the science behind the age old use of ‘biochar’ in the agriculture field to increase crop productivity. We show that biochar is essentially comprised of surface defective carbon nanoparticles, which on aerial exposure with aging may be oxidized, thus incorporating carboxylated and hydroxylated groups to form the water soluble version of carbon nanoparticles. These functional groups, along with the porous structure of such biochar, readily trap several essential mineral ions. The slow and timely release of these mineral nutrients is optimally used in enhancing the growth of wheat plant under natural conditions. We show that there is a concentration threshold and that exceeding this may be related to the supply of extra nutrients causing harm in the growth of the plant. The rCNPs in biochar work to nurse the young sapling with the slow release of essential nutrients in a sustained manner. The use of fertilizer may have the effect of a localized high dose delivery of these elements, which could ultimately cause plant senescence and even death. Biochar in a sense works as a promoter or booster, by retaining the essential elements from the organic manure or from the synthetic fertilizer applied in the soil, for the controlled supply of the optimised mineral nutrients to the plant for its normal growth. In addition it retains water which it may provide to the thirsty plant sapling during the absence of water and especially in the arid zone. This helps the optimal use of fertilizer or manure and prevents these from being wasted, by retaining the nutrients for sustained release. Therefore, more detailed research work could be possible to relate its role in conjunction with the optimal use of manure and/or fertilizer for the growth of a plant. It is to be noted that the use of nano carbons in the form of ‘biochar’ over prolonged ages does not show any adverse effects on human health.
Acknowledgements
M.S. thanks the University Grant Commission, New Delhi, India, for UGC-Dr D.S. Kothari Postdoctoral fellowship. S.M. thanks University Grant Commission, New Delhi, India, for Junior Research Fellowship. S.S. thanks the Department of Science and Technology, New Delhi, India, for Ramanna Fellowship.
Notes and references
- D. Jariwala, V. K. Sangwan, L. J. Lauhon, T. J. Marksa and M. C. Hersam, Chem. Soc. Rev., 2013, 42, 2824 RSC.
- http://scienceprogress.org/2008/07/interview-carbon-age/.
-
(a) F. G. A. Verheijen, S. Jeffery, A. C. Bastos, M. van der Velde and I. Diafas, in Biochar Application to Soils – A Critical Scientific Review of Effects on Soil Properties, Processes and Functions, EUR 24099 EN, Office for the Official Publications of the European Communities, Luxembourg, 2009, p. 149 Search PubMed;
(b) B. Glaser, L. Haumaier, G. Guggenberger and W. Zech, Naturwissenschaften, 2001, 88, 37 CrossRef CAS;
(c) Z. M. Solaiman, P. Blackwell, L. K. Abbott and P. Storer, Aust. J. Soil Res., 2010, 48, 546 CrossRef CAS;
(d) F. P. Vaccari, S. Baronti, E. Lugato, L. Genesio, S. Castaldi, F. Fornasier and F. Miglietta, Eur. J. Agron., 2011, 34, 231 CrossRef CAS PubMed;
(e) L. Van Zwieten, S. Kimber, S. Morris, K. Y. Chan, A. Downie, J. Rust, S. Jooseph and A. Cowie, Plant Soil, 2010, 327, 235 CrossRef CAS.
- N. Mulcahy, S. Baronti, F. P. Vaccari and L. Genesio, The start of a charclassification and data base, in North american Regional Biochar Conference, University of Colorado, USA, 2009 Search PubMed.
-
(a) S. Brodowski, W. Amelung, L. Haumaier, C. Abetz and W. Zee, Geoderma, 2005, 128, 116 CrossRef CAS PubMed;
(b) J. Laine, Renewable Sustainable Energy Rev., 2012, 16, 5597 CrossRef CAS PubMed;
(c) O. Paris, C. Zollfrant and G. A. Zickler, Carbon, 2005, 43, 53 CrossRef CAS PubMed;
(d) A. N. Márquez, J. L. Valverde and M. A. Keane, Appl. Catal., A, 2009, 352, 159 CrossRef PubMed;
(e) A. K. Geim and K. S. Novoselov, Nat. Mater., 2007, 6, 183 CrossRef CAS PubMed;
(f) L. E. Murr and K. F. Soto, Mater. Charact., 2005, 55, 50 CrossRef CAS PubMed;
(g) L. E. Murr, Int. J. Environ. Res. Public Health, 2008, 5, 321 CrossRef CAS PubMed.
-
(a) S. Lin, J. Reppert, Q. Hu, J. S. Hudson, M. L. Reid, T. A. Ratnikova, A. M. Rao, H. Luo and P. C. Ke, Small, 2009, 5, 1128 CrossRef CAS PubMed;
(b) S. Tripathi, S. K. Sonkar and S. Sarkar, Nanoscale, 2011, 3, 1176 RSC;
(c) S. K. Sonkar, M. Roy, D. G. Babara and S. Sarkar, Nanoscale, 2012, 4, 7670 RSC;
(d) Q. L. Liu, B. Chen, Q. L. Wang, X. L. Shi, Z. Y. Xiao, J. X. Lin and X. H. Fang, Nano Lett., 2009, 9, 1007 CrossRef CAS PubMed;
(e) M. V. Khodakovskaya, K. Silva, A. S. Biris, E. Dervishi and H. Villagarcia, ACS Nano, 2012, 6, 2128 CrossRef CAS PubMed;
(f) X. Wang, H. Han, X. Liu, X. Gu, K. Chen and D. Lu, J. Nanopart. Res., 2012, 14, 841 CrossRef;
(g) H. Villagarcia, E. Dervishi, K. Silva, A. S. Biris and M. V. Khodakovskaya, Small, 2012, 8, 2328 CrossRef CAS PubMed;
(h) P. A. Raven, R. F. Evert and S. E. Eichhorn, Biology of Plants, W. H. Freeman and Company, New York, 6th edn, 1999 Search PubMed;
(i) A. Fahn, Plant Anatomy, Pergamon Press, New York, 5th edn, 1990 Search PubMed.
-
(a) G. B. Qu, Y. H. Bai, Y. Zhang, Q. Jia, W. D. Zhang and B. Yan, Carbon, 2009, 47, 2060 CrossRef CAS PubMed;
(b) S. Kang, M. Herzberg, D. F. Rodrigues and M. Elimelech, Langmuir, 2008, 24, 6409 CrossRef CAS PubMed.
- A. S. Crafts, Plant Physiol., 1938, 13, 791 CrossRef CAS PubMed.
- M. F. Serag, N. Kaji, M. Tokeshi, A. Bianco and Y. Baba, Integr. Biol., 2012, 4, 127 RSC.
-
(a) P. Griess, Ber. Dtsch. Chem. Ges., 1879, 12, 427 Search PubMed; as cited in F. Feigl, Spot Tests in Inorganic Analysis, Elsevier, Amsterdam, 1958, p. 330 Search PubMed;
(b) S. Roy and S. Sarkar, J. Chem. Soc., Chem. Commun., 1994, 275 RSC.
-
(a) P. Dubey, D. Muthukumaran, S. Dash, R. Mukhopadhyay and S. Sarkar, Pramana, 2005, 65, 681 CrossRef CAS;
(b) S. K. Sonkar, M. Saxena, M. Saha and S. Sarkar, J. Nanosci. Nanotechnol., 2010, 10, 4064 CrossRef CAS PubMed;
(c) A. Begum, S. K. Sonkar, M. Saxena and S. Sarkar, J. Mater. Chem., 2011, 21, 19210 RSC;
(d) M. Saxena and S. Sarkar, Diamond Relat. Mater., 2012, 24, 11 CrossRef CAS PubMed;
(e) M. Roy, S. K. Sonkar, S. Tripathi, M. Saxena and S. Sarkar, J. Nanosci. Nanotechnol., 2012, 12, 1754 CrossRef CAS PubMed.
-
(a) J. Nessler, Chem. Zentralbl., 1856, 27, 529 Search PubMed;
(b) http://www.mtf.stuba.sk/docs/doc/casopis_Vedecke_prace/32/vp_bartosova_michalikova_sirotiak_soldan.pdf.
- W. Huang, S. Fernando, L. F. Allard and Y.-P. Sun, Nano Lett., 2003, 3, 565 CrossRef CAS.
- M. Saxena and S. Sarkar, RSC Adv., 2014, 4, 30162 RSC.
- T. Taniguchi, R. Kataoka and K. Futai, Soil Biol. Biochem., 2008, 40, 1235 CrossRef CAS PubMed.
- W. Xu, G. Ding, K. Yokawa, F. Baluška, Q.-F. Li, Y. Liu, W. Shi, J. Liang and J. Zhang, Sci. Rep., 2013, 3, 1273 CAS.
Footnotes |
† pH = 7.3; total dissolved solids (mg L−1) = 495; hardness (as CaCO3) (mg L−1) = 160; alkalinity (as CaCO3) (mg L−1) = 310; nitrate (mg L−1) = 0.59; sulfate (mg L−1) = 51; fluoride (mg L−1) = 0.69; chloride (mg L−1) = 235; turbidity (NTU) = 0.2; arsenic (mg L−1) = 0.002; copper (mg L−1) = BDL; cadmium (mg L−1) = BDL; chromium (mg L−1) = 0.002; lead (mg L−1) = BDL; iron (mg L−1) = 0.276; zinc (mg L−1) = BDL; fecal coliform (cfu) = 0; E. Coli (cfu) = 0. [BDL = below detection limit]. |
‡ http://www.theguardian.com/commentisfree/2009/mar/27/biochar |
|
This journal is © The Royal Society of Chemistry 2014 |