DOI:
10.1039/C4RA05887A
(Paper)
RSC Adv., 2014,
4, 63436-63441
Application of optical imaging technology on the in vitro assessment of mast cell degranulation
Received
18th June 2014
, Accepted 20th October 2014
First published on 20th October 2014
Abstract
Mast cells are major effector cells in both IgE-mediated immune responses and anaphylactoid reactions. During the process of anaphylaxis, mast cells synthesize and release inflammatory mediators from granules that induce anaphylactic symptoms. Currently, in vitro detection methods for mast cell degranulation include the colorimetric assay and morphological observations. These conventional methods are stable but have their own limitations in dynamic biological information being lost, cumbersome to operate and result with judgmental bias. To overcome these disadvantages, we developed a new approach to observe the dynamic process of degranulation in anaphylaxis. In brief, a RBL-2H3 cell line transfected with CD63-GFP (RBL-GFP) on secretory granules was established. After activated by compound 48/80 (C48/80), the degranulation process was observed by confocal laser scanning microscope (CLSM) within 10 min, CD63-GFP-labeled granules were traced and the maximum velocity was calculated by Imaris image processing and analysis software. Conventional colorimetric assay (β-HEX assay) and the morphological imaging method (Scanning Electron Microscopy, SEM) were selected as control methods. The results showed that the granules in RBL-2H3 cells moved faster towards the plasma membrane and the number of intracellular granules significantly reduced (P < 0.05) after the addition of C48/80. The maximum velocity of granules was about 0.17 μm s−1. Furthermore, morphological changes were observed in cell and nuclear volume and pseudopodia protrusion in comparison with before treatment. Therefore, this approach is a sensitive, easy to handle and low cost method, which could be applied to visualize the dynamic process of mast cell degranulation in vitro.
Introduction
Mast cells are major effector cells in both IgE-mediated immune responses and anaphylactoid reactions. During the process of anaphylaxis, mast cells synthesize and release inflammatory mediators from granules that induce anaphylactic symptoms.
The degranulation process is composed by a serial of steps, including granules transport, targeting, anchoring and fusion. Currently, in vitro detection methods for mast cell degranulation, including the colorimetric assay, which is used to detect active substances released by mast cells, such as β-hexosaminidase (β-HEX), tryptase, and histamine,1–3 and observation of morphological changes after degranulation by scanning and transmission electron microscopy.4,15,16 These conventional methods are stable but have their own limitations. Firstly, mast cell degranulation is a dynamic process; however, a series of biological information for degranulation of living cells is lost after several chemical fixations and visualization procedures which are performed for detecting corresponding indicators. Secondly, many colored substances, such as some herbal extractions, interfere with the results of the colorimetric assay, which cause many detection errors. Finally, some of the detection methods, such as electron microscopy assay, are cumbersome, complex, and expensive in the sample preparation process.
Moreover, all above mentioned methods focus on the late phase of degranulation, the consecutive morphologic changes of the individual cell, as well as the granules movement during degranulation, remain largely unclear. Therefore, a fast, simple and sensitive detection method for mast cell degranulation is urgently needed.
CD63 is a membrane surface marker located on the basophilic granule membranes in resting basophils, mast cells, and platelets. It is also known as the lysosome-associated lysosome targeting domain.9 We constructed a CD63-GFP (green fluorescent protein) plasmid and introduced it into rat basophilic leukemia (RBL-2H3) cells to observe the movements of CD63 on degranulation. Using these stably transfected cell lines, which express CD63-GFP on the secretory granules of RBL-2H3, we investigated the relationship of anaphylactoid reaction to the movement of fluorescence granules and morphological changes after sensitized with C48/80. Our data show that the new approach is coincident with the results of the β-HEX assay and SEM. Given its visualized, dynamic and simple handle feature, it may be a potential tool for allergen detection both in pharmaceutical factory and clinic.
Materials and methods
Cell culture and derivation of stable transformants of RBL-GFP
RBL-2H3 cells were incubated in MEM supplemented with 10% heat-inactivated fetal bovine serum (Hangzhou Sijiqing Biological Engineering Materials Co., Ltd, China), 100 U ml−1 penicillin, and 100 μg ml−1 streptomycin. 1 × 104 RBL-2H3 cells were plated on a 35 mm glass bottom petri dish (Hangzhou Shengyou Biotechnology Co., Ltd, China) and incubated overnight at 37 °C in a humidified incubator containing 5% carbon dioxide (Thermo Fisher Scientific Inc).
The transfection of the CD63-GFP plasmid was performed as described in the previous work from our laboratory.5 Briefly, the whole cDNA of CD63 in RBL-2H3 cells were obtained by reverse transcription-polymerase chain reaction (RT-PCR) with the 5′primer 5′AGCTTCGAATTCATGGCGGTGGAAGGAGGAATG3′ (EcoRI) and the 3′primer 5′ACCGGTGGATCCCGCATTACTTCATAGCCACTTCGA (BamHI). The pEGFP-N1 plasmid, connected with the CD63 cDNA by EcoRI and BamHI, was transformed into E. coli DH5a, and then into RBL-2H3 cells by the DNA fection kit (Tiangen, China). The RBL-2H3 cells expressed CD63-GFP plasmid were maintained in Minimum Essential Media (MEM) (Gibco) without Penicillin–streptomycin, selected in culture medium supplemented with 0.6 mg L−1 G418(Sigma, USA). 72 hours later, individual clones were isolated by flow cytometry and purified. Clones showing a homogenous level of expression were selected and further subcloned by serial dilution. Cells were trypsinized every 3 days and discarded after 12 passages.
β-HEX assays
Enzyme assays were performed on cell supernatants, according to the methods in ref. 6. Briefly, the RBL-GFP cells or RBL-2H3 were cultured in 96 wells culture plate and washed with modified Tyrode's buffer (MT) (NaCl, 137 mM; KCl, 2.7 mM; CaCl2, 1.8 mM; MgCl2, 1 mM; glucose, 5.6 mM; Hepes, 20 mM, pH 7.4; and bovine serum albumin, or BSA, 0.1%) once, then incubated with MT at 37 °C for 30 min. After stimulation with C48/80(10 μg ml−1) for 2, 4, 6, 8, 10 min, cells were incubated in MT on ice for 5 min and then centrifuged at 5000g, the supernatant was collected for enzyme activity analysis. For each well, 50 μl of supernatant was mixed with 25 μl of β-HEX in a 96-well plate (Costar, Inc.) and incubated at 37 °C for 60 min. After adding termination solution (0.1 M Na2CO3, 0.1 M NaHCO3, pH 10.7), the plates were read on a microplate reader (Biorad, Inc.) at 405 nm. The difference between RBL-GFP and RBL-2H3 in the secretion of β-HEX is also compared at 10 min activated by C48/80.
The release rate of β-HEX is calculated as below:
Scanning Electron Microscopy (SEM)
Cells were grown on glass coverslips and after antigen stimulation were fixed for 1 hour in 4% glutaraldehyde in 100 mmol L−1 phosphate buffer at pH 7.4. Samples were fixed for 1 hour in osmic acid and washed by 1 mol L−1 phosphate buffer 3 times, then gradually dehydrated using increasing concentrations of ethanol and then at critical point dried with CO2 and gold sputtering (E1010, Hitachi, Japan). The samples were observed using a Hitachi S3400N scanning electron microscope at 5 kV.
Observation of intracellular granules movement of RBL-GFP
2 ml RBL-GFP dilution (1 × 106 ml−1) were cultured 24 h in a 35 mm glass bottom Petri dish for the experiment. Real-time monitoring of granular movement during mast cell degranulation was observed with CLSM(FV-1000, OLYMPUS, Japan). The parameters for the optical imaging were the following: 512 × 512 pixels and 12-bit intensity resolution. The objective was 100× oil immersion (NA 1.45). Green fluorescent protein (GFP) was excited at 488 nm with laser intensity at 0.8%, 10 seconds per frame for 10 min after activation with C48/80. As the observation interval on RBL-GFP is 10 min, the effect of laser irradiation on cell viability was observed on rested RBL-GFP in parallel with the administration groups.
All data were processed with Auto Quant (version X2.1.3) and analysed with Imaris processing software.
Simultaneous observation of and morphological changes of RBL-GFP
In order to observe the morphological changes of the cells, cultured cells were loaded with 1 μM Calcein-AM(for cytoplasm) and 0.5 g ml−1 Hoechst 34580 (for the nucleus). After being loaded at 37 °C for 30 min, cells were observed with CLSM at 350 nm (Calcein-AM), 488 nm (GFP) and 515 nm (Hoechst 34580). 100× oil immersion objective (NA 1.45) was used and 5 μm steps in the Z-axial direction to make 3D-fluorescence imaging before (0 min) and after (5, 10 min) mast cell degranulation.
All data were processed with Auto Quant (version X2.1.3) and analysed with Imaris processing software.
Image analysis
Imaris 7.4.0 software (Bitplane, Switzerland) was used for fluorescence image analysis. 2D track module of Imaris was used to track the movement speed of fluorescent granules.
The surface measurement module was used for reconstruction of three-dimensional structures of granules, nucleus, and the cytoplasm.
Statistical analysis
All experiments were performed at least three times. All data are expressed as mean ± SD. The significance of the difference between samples and control groups was analysed by t-test.
Results
Detection of β-HEX released from sensitized RBL-GFP
To characterize the ability of RBL-GFP, β-HEX release was used to verify that the transfected cells still had normal function of mast cell degranulation. The result show that 2 min after being treated with C48/80(10 μg ml−1), the release of β-HEX could be detected and continue to increase in a dose-dependent manner until 10 min (Fig. 1A), whereas the negative control group showed no obvious changes.
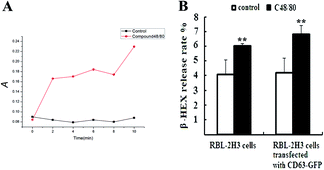 |
| Fig. 1 β-HEX release of RBL-GFP stimulated by C48/80. To characterize the ability of RBL-GFP, β-HEX release was used to verify that transfected cells still had normal function of mast cell degranulation. (A) RBL-GFP, treated with compound 48/80 at 10 μg ml−1 for 2, 4, 6, 8, 10 min. β-HEX release was determinated by colorimetric assay. The negative control was treated with the same volume of saline. (B) β-HEX release rate of RBL-2H3 and RBL-GFP after being activated with C48/80 at 10 μg ml−1 for 10 min. n = 3, mean ± SD **P < 0.01 vs. control. | |
Moreover, the release of β-HEX from the RBL-2H3 cells and RBL-GFP was compared after being activated with C48/80 for 10 min (Fig. 1B). The result showed that RBL-GFP behaved in similarity with the normal RBL-2H3 cell line, which means the transfection did not affect the degranulation character of RBL-GFP.
Morphological changes of RBL-GFP cells observed by SEM
Control RBL-2H3 cells exhibited a heterogeneous display of membranous ridges (Fig. 2A). When the cells are stimulated with C48/80 (10 μg ml−1), they are not activated in a synchronized manner—i.e., the amounts of time that it takes for the individual cells to undergo exocytosis and recovery differ. Therefore, the cells were at different degranulation and recovery stages (Fig. 2B–D). In Fig. 2B and C, a RBL-GFP cell had lost a lot of its surface protrusions 5–10 min after stimulation. These protrusions contain intracellular granules, in which it has been suggested that granule–plasma membrane fusions can be observed during exocytosis. In Fig. 2D, the cells that probably undergo intermediate stages of mast cell degranulation are shown, in which retracted membranous hole are present.
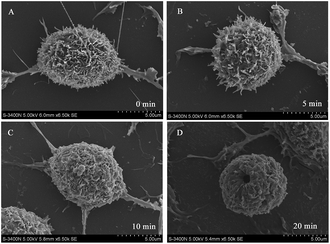 |
| Fig. 2 Morphological changes of RBL-GFP cells stimulated with C48/80. Cells were then fixed and processed for SEM and observed at 5 kV, 6500× (shown in method). (A) The microvillous dorsal surface of unstimulated RBL-GFP cells that spread and formed multiple membrane ruffles in response to antigen stimulation. (B) and (C) RBL-GFP cell had lost a lot of its surface protrusions 5–10 min after stimulation, (D) cells rounded up and membranous retracts could be observed (a big hole appears) after stimulation for 20 min. | |
Analysis of the quantity and velocity of granules during mast cell degranulation
Many different sizes of granules with a diameter ranging from 0.46–1.78 μm were observed (Fig. 3B). Uniform green fluorescence was expressed in the granule membranes of RBL-GFP, and movement changes of fluorescent granules were dynamically tracked by the CLSM. In resting cells, 80% of the granules did not move or only did irregular Brownian movements, with a speed of only (2.0 ± 0.2) × 10−2 μm s−1. Only 20% of granules moved fast along the longitudinal axis of a pseudopodium. During degranulation, granules moved gradually towards plasma membrane with a maximum speed of up to (17.0 ± 0.5) × 10−2 μm s−1 (Fig. 3A), and the number of granules was decreased from (1.76 ± 0.13) × 102 to (1.38 ± 0.08) × 102 (Fig. 3C). Many granules deviated from the focal plane, or fused into a larger one for extra cellular secretion.
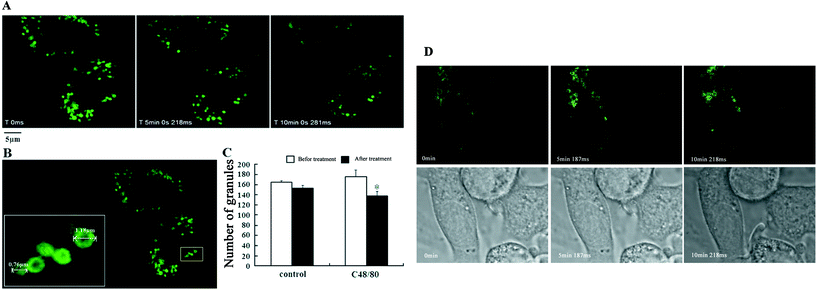 |
| Fig. 3 Movement analysis of secretory granules after being stimulated with compound 48/80 in RBL-GFP cells and cell viability detection of RBL-GFP Scanning with CLSM system A: time-lapse confocal images of secretory granules at 0, 5, 10 min after being stimulated with C48/80 (10 μg ml−1). The maximum velocity of granules was about 0.17 μm s−1 and the number of granules decreased compared with 0 min; B: the diameter ranging of granules was from 0.46 μm to 1.78 μm; C: granules counting in RBL-2H3 cells before (white bar) and after degranulation treated with compound 48/80 (black bar). All data points represent the mean ± SD of measurements made in quadruplicate.*P < 0.05 vs. values before degranulation; D: the cell state no significant change after a continuous observation for 10 min (488 nm, 100× oil immersion lens, sampling rate 12.5 μs per pixels, laser intensity 0.8%). | |
In order to exclude the laser radiation damage on the cells during the observation, a parallel observation of CLSM was conducted on resting RBL-GFP cells. The results showed that after a continuous observation, cells were alive without shrinkage and death, under the scanning condition for 10 minutes (Fig. 3D). The experimental conditions did not affect cell morphology.
Changes of cell 3-D structure after mast cell degranulation
Reconstruction of the 3-D structure was performed after scanning of the optical sections of mast cells. Surface renderings of cytoplasm, nucleus, and granules were processed by Imaris (Fig. 4A) and results were obtained using corresponding algorithms of Imaris.
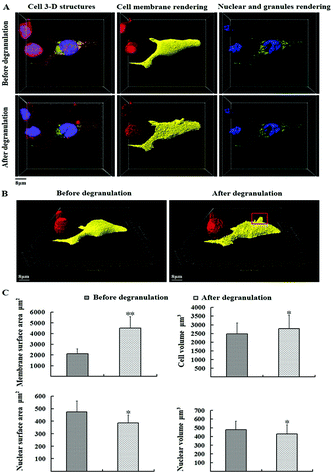 |
| Fig. 4 Morphological changes in RBL-GFP cells stimulated by C48/80. (A) 3-D construction and surface rendering of RBL-GFP cells loaded with Hoechst 34580 and volumetric indicator Calcein-AM. Morphological changes of cell surface area, cell volume and nuclear were observed before and after mast cell degranulation. Blue: nuclear, yellow: cell membrane, green: secretory granules; (B) newly formed Pseudopodia protrusion (red box in right panel) was observed after C48/80-induced degranulation; (C) the surface and nuclear changes of RBL-GFP measured by Imaris before and after degranulation. N = 6, mean ± SD. *P < 0.05, **P < 0.01 vs. control. | |
A newly formed Pseudopodia protrusion was observed after degranulation (Fig. 4B, red box), which was easily ignored without rendering.
As was shown in Fig. 4C, after mast cell degranulation, the cell surface area was increased from (2.1 ± 0.5) × 103 μm square to (4.5 ± 1.1) × 103 μm square, which was expanded 1.12 times as that before degranulation. Cell volume was expanded from (2.5 ± 0.3) × 103 μm cubic to (2.8 ± 0.3) × 103 μm cubic, with an increase of 12.2%. The nuclear surface area was decreased from (4.7 ± 0.9) × 102 μm square to (3.9 ± 0.6) × 102 μm square, with a 17% reduction. Nuclear volume was reduced from (4.8 ± 1.0) × 102 μm cubic to (4.3 ± 1.0) × 102 μm cubic, which was reduced by 11.8%. Thus, morphological changes in cell surface, cell volume, nuclear surface area could be recognized after mast cell degranulation.
Discussion
Traditional Chinese medicine (TCM) injection is a new formulation from China. There are more than 70 types of TCM injection from the 1950s. It has been widely used in clinics for several decades and tested to be effective in China. However, in recent years, for production and quality control reasons, security incidents occurred frequently. It was reported that 60% of the adverse effects are anaphylactoid reaction.7 In view of the danger of allergic reactions, people are becoming increasingly aware of the importance of early prevention. Hence, early and rapid diagnosis of allergic reactions is one of the best tools for reducing the adverse effect of TCM injections.
The colorimetric assay and morphology detection are the main detection methods for in vitro mast cell degranulation. Usually, we use the end-point colorimetric assay to detect the release of β-HEX, tryptase, and histamine to determine antigens for mast cell degranulation. As the detection wave length is usually 405 nm, it will always be a problem for the research of herbal medicine, because dark color substances from herbal medicine covered the spectrum from 400–500 nm, this may interfere with the results of the colorimetric assay, which cause many detection errors. Therefore, it has greatly limited the application of the colorimetric assay in TCM safety control.
SEM is a typical morphology detection method. To get good images, samples need fixation, dehydration and dryness with critical point drier. However, mast cell degranulation is a dynamic process, and a series of biological information about the degranulation of the living cells is lost after this series of activities. Moreover, some reagents used in SEM, such as osmic acid, are toxic and expensive.
The early stage of mast cell degranulation consists of a series of steps, including transportation, orientation, and anchoring of granules, as well as their integration with the plasma membrane. Each step is strictly regulated.8 Thus to observe the movement of granules and monitor the membrane integration process directly is the most reliable and simple method.
In our previous study, RBL-2H3 cells that stably expressed CD63-GFP was established by plasmid transfection and secretory granules were directly visualized during degranulation. As excitation wave length of GFP is 488 nm and emission at 507 nm, it can successfully avoid the disturbance from the dark color of herbal extractions and TCM injection, therefore it is suitable for the allergen tracing research in TCM injection.
In this study, the dynamic changes of secretory granules and extraction of characteristic parameters of RBL-2H3 cells after degranulation were further focused on. Movement speed of CD63-GFP labeled granules were dynamically increased in degranulation. Therefore, we can judge mast cell degranulation only from the movement speed of the granules and access the risks of anaphylactoid reactions. It is simple and low cost.
The results showed that during mast cell degranulation, morphological changes, such as increased cell surface area, expanded cell volume, nuclear condensation, and reduced nuclear volume, were observed, which is important to determine the anaphylactoid reaction.
It was observed in this study that in resting cells, most granules nearly did not move or only did irregular Brownian movements, whereas in cells with degranulation, granules moved gradually towards the plasma membrane and within 10 min many granules deviated from the focal plane, resulting in reduced number of granules in the focal plane. Similarly to the findings of Amano T.,10 we also observed that in mast cells without degranulation, a small part of granules moved fast along the longitudinal axis of a pseudopodium. Studies have shown that the long-distance movement and exocytosis of CD63 labeled secretory granules depended on microtubule assembly and activity of protein kinase C.11–13 However, the translocation of some recycling endosomes to the plasma membrane depended neither on protein kinase C,14 nor on microtubule aggregation. Therefore, our next focus is microtubule rearrangement and its downstream signal transduction regulation of secretory granules or space targeting of other endosomes.
Conclusions
In this study, optical imaging technology was applied to study the dynamic process of mast cell degranulation, and images collected were qualitatively and quantitatively analyzed with Imaris software. Thus a new approach for rapid in vitro assessment of mast cell degranulation was created. Characteristic changes of mast cell degranulation were described from the aspects of dynamic changes of secretory granules, increased cell surface area after degranulation, nuclear condensation, and pseudopodia protrusion. Data of mast cell degranulation were dynamically obtained with multiple parameters, which overcame the deficiencies of the conventional methods. It will provide a new method for allergen screening in drug safety evaluations and allergen detection in clinics. Further work will carry on in qualitative and quantitative analysis.
Acknowledgements
This work is supported by Project supported by the National Natural Science Foundation of China no. 81373884. Basic scientific research fund from Ministry of Fina e of China no. ZZ2011010, no. ZZ2011011. National Natural Science Foundation of Beijing, China no. 7132157.
Notes and references
- J. Li, J. Jin, C. W. Guan, P. Li, J. S. Tu, H. M. Sun and Z. Y. Huang, Study of degranulation in mast cell RBL-2H3 induced by Tween 80, Drug Eval. Res., 2010, 005, 379–383 Search PubMed.
- W. Wang, Q. Zhou, L. Liu and K. Zou, Anti-allergic activity of emodin on IgE-mediated activation in RBL-2H3 cells, Pharmacol. Rep., 2012, 64(5), 1216–1222 CrossRef CAS.
- Y. Hagenlocher, I. Bergheim, S. Zacheja, M. Schäffer, S. C. Bischoff and A. Lorentz, Cinnamon extract inhibits degranulation and de novo synthesis of inflammatory mediators in mast cells, Allergy, 2013, 68(4), 490–497 CrossRef CAS PubMed.
- L. Zhu, H. M. Lu, C. X. Yu, X. L. He and D. H. Su, Observation on anti-IgE antibody-induced mast cell degranulation by electron microscopy, Chin. J. Immunol., 2002, 18(5), 357–357 Search PubMed.
- J. J. Hu, Y. M. Hou, Q. Zhang, H. T. Lei, Y. Wang and D. Q. Wang, Real-time detection of mast cell degranulation in anaphylactoid reaction, China J. Chin. Mater. Med., 2011, 36(14), 1860 Search PubMed.
- W. Wang, Q. Zhou, L. Liu and K. Zou, Anti-allergic activity of emodin on IgE-mediated activation in RBL-2H3 cells, Pharmacol. Rep., 2012, 64(1216), 1216–1222 CrossRef CAS.
- H. F. Zhang, C. K. Zhai and Y. Z. Zheng, Advancement of experimental studies on sensitization research of Traditional Chinese Medicine Injection, Chin. J. Exp. Tradit. Med. Formulae, 2010, 16(10), 204–206 Search PubMed.
- D. F. Liu, C. G. Zhang and J. M. wu, Study on mechanisms of mast cell degranulation, Clin. Biochem. Lab. Med., Foreign Med. Sci., 2004, 25(2), 137–139 Search PubMed.
- S. Levy and T. Shoham, The tetraspanin web modulates immune-signalling complexes, Nat. Rev. Immunol., 2005, 5(2), 136–148 CrossRef CAS PubMed.
- T. Amano, T. Furuno, N. Hirashima, N. Ohyama and M. Nakanishi, Dynamics of intracellular granules with CD63-GFP in rat basophilic leukemia cells, Journal of Biochemistry, 2001, 129(5), 739–744 CrossRef CAS.
- K. Nishida, S. Yamasaki, Y. Ito, K. Kabu, K. Hattori, T. Tezuka, H. Nishizumi, D. Kitamura, R. Goitsuka and R. S. Geha, FcεRI-mediated mast cell degranulation requires calcium-independent microtubule-dependent translocation of granules to the plasma membrane, J. Cell Biol., 2005, 170(1), 115–126 CrossRef CAS PubMed.
- U. Blank and J. Rivera, The ins and outs of IgE-dependent mast-cell exocytosis, Trends Immunol., 2004, 25(5), 266–273 CrossRef CAS PubMed.
- R. M. Z. Naal, E. P. Holowka, B. Baird and D. Holowka, Antigen-Stimulated Trafficking from the Recycling Compartment to the Plasma Membrane in RBL Mast Cells, Traffic, 2003, 4(3), 190–200 CrossRef CAS.
- M. Wu, T. Baumgart, S. Hammond, D. Holowka and B. Baird, Differential targeting of secretory lysosomes and recycling endosomes in mast cells revealed by patterned antigen arrays, J. Cell Sci., 2007, 120(17), 3147–3154 CrossRef CAS PubMed.
- S. D. Demo, E. Masuda, A. B. Rossi, B. T. Throndset, A. L. Gerard, E. H. Chan, R. J. Armstrong, B. P. Fox, J. B. Lorens, D. G. Payan, R. H. Scheller and J. M. Fisher, Quantitative measurement of mast cell degranulation using a novel flow cytometric annexin-V binding assay, Cytometry, 1999, 36(4), 340–348 CrossRef CAS.
- Z. Xiang, M. Block, C. Löfman and G. Nilsson, IgE-mediated mast cell degranulation and recovery monitored by time-lapse photography, J. Allergy Clin. Immunol., 2001, 108(1), 116–121 CrossRef CAS PubMed.
Footnote |
† These authors contributed equally to the work. |
|
This journal is © The Royal Society of Chemistry 2014 |