DOI:
10.1039/C4RA05058D
(Paper)
RSC Adv., 2014,
4, 48671-48675
Aptasensor for label-free square-wave voltammetry detection of potassium ions based on gold nanoparticle amplification†
Received
4th July 2014
, Accepted 26th September 2014
First published on 26th September 2014
Abstract
Herein, we present a very simple and label-free square-wave voltammetry (SWV) aptasensor to detect potassium ions (K+), in which a K+-specific aptamer was used as an ion recognition element, and a redox couple [Fe(CN)6]3−/4− as a redox probe. At the bare gold electrode, the redox couple [Fe(CN)6]3−/4− can very easily access the electrode surface to produce a very strong SWV signal. At the K+-specific aptamer/gold electrode surface, in the presence of K+, the single-stranded DNA with guanine (G)-rich sequences could fold into a secondary structure, G-quadruplex, which resulted in less availability for a redox reaction, and led to a smaller SWV current. To improve signal intensity, gold nanoparticles (AuNPs) were anchored to a gold electrode surface which had been previously modified with self-assembled monolayers of p-aminothiophenol. The SWV peak current decreased with K+ concentration, and the plot of the SWV peak current against the logarithm of K+ concentration is linear over the range from 10 pM to 0.1 μM and 0.5 μM to 1 mM, with a detection limit of 0.13 pM. The aptasensor also showed a very good selectivity for K+ without being affected by the coexistence of other ions.
Introduction
Aptamers are artificial single-stranded oligonucleic acids selected in vitro through systematic evolution of ligands by exponential enrichment (SELEX).1 They have high affinity and selectivity toward a variety of targets, including small molecules,2 proteins,3 metal ions,4 drugs,5 and even whole cells.6 In particular, a single-stranded DNA with guanine (G)-rich sequences can fold into a secondary structure, G-quadruplex, via intramolecular hydrogen-bonding interactions in the presence of metal ion.7–9 The appearance of G-quadruplex DNA as a functionalized nucleic acid provides a chance for targets detection such as K+. A number of sensors have been constructed based on the G-quadruplex.10–15
Potassium ions, which is the most abundant metal ion in cells, play essential roles in biological systems, such as nerve transmission, maintenance of muscular strength and extracellular osmolarity, enzyme activation, apoptosis of a cell, regulation of blood pressure, pH, and the concentration of other ions in living cells.16–21 Normal serum K+ levels are between 3.8 and 5.4 mmol L−1,22 the abnormal K+ concentration is a symptom of several diseases, including kidney diseases, alcoholism, anorexia, bulimia, heart disease, diabetes, AIDS and cancer.23 Therefore, it is very important to be able to assess this ion at trace level with high sensitivity.
In recent years, a few aptamer-based analytical methods have been placed toward K+ recognition and detection. Kim et al.24 have developed a highly sensitive and selective homogeneous sensory system for K+ ions based on cationic conjugated polyelectrolyte (CPE) and molecular beacon aptamer (MBA) that operates in an excess presence of Na+ ions. A novel K+ detection method was reported by Kong et al.25 using a label-free G-quadruplex-forming oligonucleotide and a triphenylmethane fluorescent dye crystal violet (CV). Ren et al.26 designed three pairs of split G-quadruplex probes and investigated the sensitivity and selectivity of these systems in terms of potassium ion concentration and split modes of G-quadruplex. A tricationic phenylene–ethynylene (N3+) fluorophore is investigated by Yuanboonlim et al.27 as a fluorescent transducer in homogeneous aptasensing system for potassium ion assay in aqueous media. A simple system for colorimetric detection of K+ at room temperature was developed by Chen et al.,28 the system utilizes the network assembly of aptamer–AuNPs by K+. However, these suffer from drawbacks, including unstable florescence properties, potential false signals from contaminating colorants, fluorophores and quenchers, and frequently, a reliance on cumbersome optical equipment. Ge et al.29 have demonstrated that a practical nanoelectronic switch can be designed and utilized on a conductive electronic chip by using contractile DNA duplexes as the switchable medium. Such a prototype finds real-time application in sensing applications for K+ ions. Radi and O'Sullivan30 report the first use of aptasensor based on ferrocene-labeled aptamer and a signal-on architecture for electronic recognition of potassium ions in aqueous solution. A novel on/off electronic nanoswitch is for the first time described by Wu et al.31 based on the conformational change of DNA sequence possessing a single guanine (G)-rich stretch. However, they suffer from disadvantages such as relatively high detection limits and narrow detection ranges. Among these methods, the electrochemical methods have attracted most attention in the development of aptasensors due to their high sensitivity, fast response, simple instrumentation, low production cost, and portability.
Among all the voltammetric techniques, square-wave voltammetry (SWV) has many advantages, such as the excellent sensitivity and the high speed. This high speed, coupled with computer control and signal averaging, allows for experiments to be performed repetitively and increases the signal-to-noise ratio. Compared to both linear sweep and cyclic voltammetry, square-wave voltammetry has a much broader dynamic range and lower limit of detection because of its efficient discrimination of capacitance current.
Here we describe a label-free electrochemical biosensor for detection of K+ ion based on gold nanoparticles (AuNPs) and K+-specific aptamer. Compared to other types of nanoparticles, AuNPs are superior for many reasons such as electrical conductivity, biocompatibility and ease of self-assembly through a amino group. AuNPs can also increase the active surface area of the electrode and the amount of immobilized DNA probes.32 Our fabrication process involves a self-assembled monolayer of p-aminothiophenol (p-ATP), which has been used as a medium to link AuNPs to the surface of a bare gold electrode. The immobilization of the recognition element (K+-specific aptamer) introduces electrical insulation and kinetic-transfer barriers at the electrode surface. The transduction principle is based on recognition-induced steric-hindrance in the presence of an [Fe(CN)6]3−/4− redox couple, which can be measured by SWV. The aptamer–K+ interaction leads to the conversion of a G-rich sequence from a loose random coil into a compact G-quadruplex, leading to a substantial decrease in SWV current. The analytical technique described here only involves the utilization of a single-stranded DNA aptamer and a redox probe in solution. Thus the proposed SWV aptasensor for K+ is very simple, rapid, cost-effective, highly sensitive and reproducible, especially, it is label-free, and requires no external modification on the biomolecules.
Experimental
Materials and apparatus
The single strand DNA oligonucleotide was synthesized by TaKaRa Biotechnology (Dalian, China) Co., Ltd. The sequence of oligonucleotide employed is: 5′-TTTGGTTGGTGTGGTTGGTTT-3′-(CH2)6-SH. Tris-(2-carboxyethyl) phosphine hydrochloride (TCEP) was obtained from the Alfa Aesar (Tianjing) company. Tris-base was purchased from Sigma-Aldrich.
An advanced electrochemical system (Princeton Applied Research (PARSTAT 2273)) was used for the electrochemical measurements. All experiments were performed using a conventional three-electrode system with a fabricated aptasensor or bare gold (diameter, 2 mm) as the working electrode, Ag/AgCl (sat. KCl) as the reference electrode, and a platinum as counter electrode. All potentials were referred to the reference electrode.
All other reagents were of analytical reagent grade. All solutions were prepared with doubly distilled water. 20 mM Tris–HCl buffer (pH 7.40) containing 1 mM Na4Fe(CN)6/Na3Fe(CN)6 was chosen as the supporting electrolyte for electrode characterization and K+ assays.
Preparation of AuNPs
Colloidal AuNPs were prepared by citrate reduction of HAuCl4 in aqueous solution according to the literature.33 The prepared Au colloidal nanoparticles were characterized by TEM (transmission electron microscopy) and UV-vis spectrometry as shown in Fig. S1.† Fig. S1(a)† exhibits the spherical AuNPs with an average diameter of ∼15 nm, Fig. S1(b)† presents the characteristic UV absorption peak of AuNP colloid at about 520 nm, consistent with the absorption of the AuNPs of this size.34
Electrode modification and immobilization of the aptamer
Prior to modification, the gold electrode was polished with 1.0, 0.3, 0.05 μm alumina powders respectively, and rinsed with bidistilled water and ethanol. The electrode was then sonicated in bidistilled water for 5 min to remove bound particles, then rinsed thoroughly with bidistilled water and dried in nitrogen stream. Afterwards, the pretreated electrode was immersed into ethanol solution containing 10 mM p-aminothiophenol (p-ATP) for 24 h at room temperature. After being thoroughly rinsed with ethanol and bidistilled water, the electrode was dried under a N2 stream and then immerged into the AuNPs colloid for 18 h. For immobilization of aptamer, the electrode was covered with a 10 μL droplet of the 1 μM K+-specific aptamer for 24 h at 100% humidity at room temperature. Of note, The ssDNA was prepared as follows: the aptamer (1 μM) including 1 mM Tris-(2-carboxyethyl) phosphine hydrochloride (TCEP was employed to reduce disulfide bond oligos) in immobilization buffer (20 mM Tris–HCl, pH 7.40) was heated to 90 °C for 5 min and then gradually cooled to room temperature. This step is helpful to maintain the structural flexibility of the aptamer for binding K+. For K+ assays, the ssDNA/AuNP/p-ATP/gold electrode was placed into 5 mL K+ solutions of various concentrations.
Results and discussion
Sensing mechanisms of aptasensor
In this work, the fabrication steps and the sensing mechanism to this K+ aptasensor are shown in Scheme 1. At the AuNP/p-ATP/gold electrode, the redox couple [Fe(CN)6]3−/4− can be very easily accessed to the electrode surface to give a very strong signal. After the modification of K+-specific aptamer, the weaker SWV peak current is observed, which is attributed to the increasing kinetics barrier between [Fe(CN)6]3−/4− and the negatively charged phosphate backbone of the aptamer. In the presence of K+, the aptamer part bound to K+ and folded to a G-quaduplex structure (Fig. S2†). G-Quaduplex DNA has a much higher space charge density than ssDNA, and thus lead to even smaller SWV current. With increase of the concentration of K+, less and less redox couples could be approached to the electrode surface, therefore, SWV current decreases.
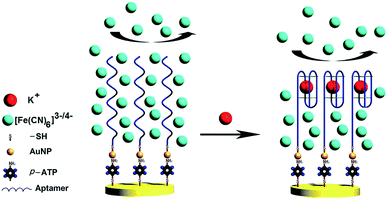 |
| Scheme 1 The proposed mechanism of the signal-off electronic, aptamer-based sensor. | |
Characterization of aptasensor
Electrochemical impedance spectroscopy (EIS) is an effective method for monitoring the changes in the surface feature of the modified electrodes in the assembly process. As shown in Fig. 1, it is observed that the bare gold electrode revealed a very small semicircular domain (Fig. 1, curve a), indicating a charge transfer resistance (Rct) value about 200 Ω. After the p-ATP was assembled on the surface of the electrode, the Rct increased from 200 Ω to about 1400 Ω (Fig. 1, curve b). When AuNPs were self-assembled on the surface of the p-ATP-modified gold electrode, the electrochemical response was a nearly straight line again (Fig. 1, curve c), which can be attributed to the fact that AuNPs can further enhance the electron transfer rate and improve the adsorption capacity of the electrode, and further increase the effective electrode surface area and thus have the potential to anchor more DNA probes to amplify the signal. After aptamer was immobilized onto the AuNP/p-ATP/gold electrode, the Rct significantly increased to 400 Ω (Fig. 1, curve d). This can be attributed to the fact that [Fe(CN)6]3−/4− received electrostatic repulsive forces from the negatively charged DNA phosphate backbones.
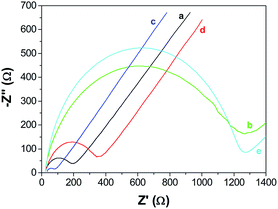 |
| Fig. 1 Nyquist plots of impedance spectra at (a) a bare gold electrode, (b) the p-ATP/gold electrode, (c) the AuNP/p-ATP/gold electrode, (d) the aptamer/AuNP/p-ATP/gold electrode, (e) 1 pM K+/the aptamer/AuNP/p-ATP/gold electrode in 20 mM Tris–HCl buffer containing 1 mM Na3Fe(CN)6–1 mM Na4Fe(CN)6 (pH 7.40). | |
Packing density of DNA probes
The signal of an electrochemical biosensor depends on the amount of probes on the electrode.35 The packing densities of DNA probes immobilized on both bare gold electrode, and AuNP/p-ATP/gold electrode were measured by electrochemical methods.36 By our experiments (Fig. S3†), the surface coverage of the DNA are estimated at 5 × 1012 molecules cm−2 and 8 × 1012 molecules cm−2, on the bare gold electrode and the AuNP/p-ATP/gold electrode, respectively. The active surface areas of a bare gold electrode and an AuNP-modified gold electrode were measured by CV in 0.5 M H2SO4 (Fig. S4†). Assuming a specific charge of 386 μC cm−2 is required for gold oxide reduction,37 The AuNP/p-ATP/gold electrode had a total active surface of 0.22 cm2, whereas that of the corresponding bare gold electrode was 0.047 cm2, indicating that the active electrode surface area had been enhanced approximately 4.68 times by AuNP modification. Therefore, the total amount of immobilized DNA probes would be 2.35 × 1011 molecules and 1.76 × 1012 molecules, for the bare electrode and the AuNP-modified electrode, respectively, a 7.49-fold increase. Therefore the proposed aptasensor with AuNP modification could detect more K+, enhancing the signal response.
SWV measurement of K+
The aptamer-based sensor is sensitive and specific to its target ion. In order to test signal enhancement for aptasensor with AuNP modification, SWV was carried out at both the aptamer/gold electrode and the aptamer/AuNP/p-ATP/gold electrode. Fig. 2(A) and (C) show the SWV profiles for aptamer/gold electrode and the aptamer/AuNP/p-ATP/gold electrode, respectively, after reacting with various concentrations of K+. With increasing amounts of K+, the SWV peak current decreases gradually. In particular, Fig. 2(B) and (D) show the signal suppression in SWV as a function of K+ concentration. The signal suppression in SWV observed at a given K+ concentration, which is the fractional decrease in SWV peak current observed upon target binding. The signal suppression is calculated by the relative change in peak signal upon addition of target from the original signal observed in the absence of target. The maximum sensor suppression is reached when the concentration increases up to 0.1 mM for both the aptamer/gold electrode and the aptamer/AuNP/p-ATP/gold electrode. From inset of Fig. 2(B), for the aptamer/gold electrode, the SWV peak current is linear with logarithm of K+ concentration over the range from 50 nM to 0.1 mM and the detection limit is 12.1 nM as calculated in terms of the 3σ rule.38 In contrast, with modification of AuNPs, the very good linear relationship can be obtained in two regions: 10 pM–0.1 μM and 0.5 μM to 1 mM. By comparison, the signal suppression of the aptasensor with the AuNP modification is larger than that of bare gold electrode at each specific concentration of K+. The large signal change can result in a change in K+ concentration of several orders of magnitude, leading to higher aptasensor sensitivity. The detection limit has greatly decreased to 0.13 pM, as shown in inset of Fig. 2(D). AuNP modification lowers the detection limit by 5 orders of magnitude. The relative standard deviations for results from the aptamer/gold electrode and the aptamer/AuNP/p-ATP/gold electrode for each K+ concentration are listed in Table S1.† These indicate that the proposed biosensor offers excellent reproducibility (RSDs < 4.98%, n = 4). The Nyquist plots of aptamer/AuNP/gold electrode for K+ with different concentrations is shown in Fig. S5,† which further confirms the process above. Particularly, the biosensor equilibrates very rapidly. We observe about 99% total signal change within 30 s and complete saturation in less than 30 s (Fig. S6†). Therefore, it can be served as an on-line sensor for the detection of K+.
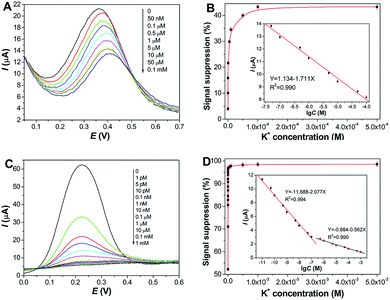 |
| Fig. 2 (A) Square-wave voltammograms of aptamer/gold electrode to different concentrations of K+. (B) The signal suppression in the SWV as a function of K+ concentration over the range from 50 nM to 0.1 mM. Inset: the SWV peak current is linear with logarithm of K+ concentration over the range from 50 nM to 0.1 mM. (C) Square-wave voltammograms of aptamer/AuNP/p-ATP/gold electrode to different concentrations of K+. (D) The signal suppression in the SWV as a function of lysozyme concentration over the range from 1 pM to 1 mM. Inset: the SWV peak current is linear with logarithm of K+ concentration over the range from 10 pM to 0.1 μM and 0.5 μM to 1 mM, respectively. | |
Selectivity of the proposed aptasensor
Not only does an aptasensor have to be sensitive to different concentrations of the target, it must also be specific. In order to evaluate the selectivity of the proposed sensor for the detection of K+ (0.1 μM), comparative trials were carried out by using Ca2+, Mg2+, Na+, Li+, NH4+, Rb+, Cs+, Sr2+ and Ba2+ at 10 μM concentrations, as the potential interference ions, when the same experimental conditions were used. As shown in Fig. 3(A), compared to background, no significant signal change was observed. After the addition of 0.1 μM K+, indicating perfect selectivity for this proposed sensor. In Fig. 3(B), the value at the y-axis can be normalized by the current decrease for K+ versus the initial background, the relative signal suppression for all other ions is only below 5%. We attributed the factor for high selectivity to the specific binding between and K+ the aptameric G-quadruplex structure.
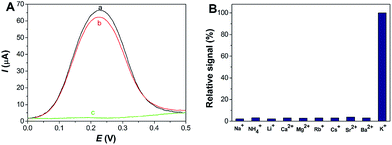 |
| Fig. 3 (A) Square-wave voltammograms for the aptamer/AuNP/p-ATP/gold electrode after reacting with (a) 0 M K+, (b) a mixture of 10 μM Ca2+, Mg2+, Na+, Li+, NH4+, Rb+, Cs+, Sr2+ and Ba2+. (c) 0.1 μM K+. (B) Relative response of the sensing system to the various ions. | |
Application of the aptasensor
To investigate if the method was applicable to real samples, we analyzed the urine samples from three healthy volunteers, first, We filtered the urine samples from four healthy volunteers through 0.2 mm membrane, and then diluted the samples 100-fold using deionized water, which was helpful to diminish false positive contributions from the matrix. The percent of accuracy was calculated by the ratio between the concentration obtained by our method and the one obtained by the immunoassay. The accuracy between 97.6% and 102.3% (n = 3) shows a satisfactory result, as shown in Table 1.
Table 1 Results of determination of K+ in urine samples
Samples |
Clinical data (mM) |
This method (mM) |
Percent of accuracy (%) |
1 |
30.5 |
31.2 |
102.3 |
2 |
50.7 |
49.5 |
97.6 |
3 |
100.9 |
101.2 |
100.3 |
Conclusions
In this work, we propose a simple, reversible signal-off SWV electrochemical aptasensor for detection of K+, in which a K+-specific aptamer was used as an ion recognition element, and a redox couple [Fe(CN)6]3−/4− as a redox probe. The proposed approach, in which AuNPs can increase the active surface area of the electrode and provide a new platform with more aptamer immobilization for target ions capture than the aptasensor without AuNP modification, exhibits remarkably high sensitivity. The very low detection limit of 0.13 pM was also achieved by this AuNP modification. The proposed sensing protocol exhibits reasonable selectivity for its target ions and excellent application in real samples.
Acknowledgements
All authors gratefully acknowledge the financial support of Scientific Research Project of Beijing Educational Committee (KM201410028006) and the Natural Science Foundation of China (no. 21371123).
Notes and references
- C. Tuerk and L. Gold, Science, 1990, 249, 505 CAS.
- C. Deng, J. Chen, L. Nie, Z. Nie and S. Yao, Anal. Chem., 2009, 81, 9972 CrossRef CAS PubMed.
- H. Chang, L. Tang, Y. Wang, J. Jiang and J. Li, Anal. Chem., 2010, 82, 2341 CrossRef CAS PubMed.
- M. S. Choi, M. Yoon, J. O. Baeg and J. Kim, Chem. Commun., 2009, 7419 RSC.
- C. Zhang and L. W. Johnson, Anal. Chem., 2009, 81, 3051 CrossRef CAS PubMed.
- Y. Wang, Z. Li, D. Hu, C. T. Lin, J. Li and Y. Lin, J. Am. Chem. Soc., 2010, 132, 9274 CrossRef CAS PubMed.
- J. T. Davis, Angew. Chem., Int. Ed., 2004, 43, 668 CrossRef CAS PubMed.
- F. He, Y. Wang, S. Wang, Y. Li and D. Zhu, J. Am. Chem. Soc., 2005, 127, 14286 Search PubMed.
- J. H. Guo, L. N. Zhu, D. M. Kong and H. X. Shen, Talanta, 2009, 80, 607 CrossRef CAS PubMed.
- E. Sharon, R. Freeman and I. Willner, Anal. Chem., 2010, 82, 7073 CrossRef CAS PubMed.
- K. Meguellati, G. Koripelly and S. Ladame, Angew. Chem., Int. Ed., 2010, 49, 2738 CrossRef CAS PubMed.
- K. Zhang, X. Zhu, J. Wang, L. Xu and G. Li, Anal. Chem., 2010, 82, 3207 CrossRef CAS PubMed.
- Y. Lin, C. Liu and H. Chang, Talanta, 2011, 84, 324 CrossRef CAS PubMed.
- W. Cai, Y. Fan, Z. Jiang and J. Yao, Talanta, 2010, 81, 1810 CrossRef CAS PubMed.
- Y. Peng, L. D. Li, X. J. Mu and L. Guo, Sens. Actuators, B, 2013, 177, 818 CrossRef CAS PubMed.
- S. J. Lippard and J. M. Berg, University Science Books, Mill Valley, CA, 1994 Search PubMed.
- M. Teresa, S. R. Gomes, K. S. Tavares and J. Oliveira, Analyst, 2000, 125, 1983 RSC.
- H. He, M. A. Mortellaro, M. J. P. Leiner, R. J. Fraatz and J. K. Tusa, J. Am. Chem. Soc., 2003, 125, 1468 CrossRef CAS PubMed.
- H. C. Kuo, C. F. Cheng, R. B. Clark, J. J. C. Lin, J. L. C. Lin, M. Hoshijima, V. T. B. Nguyen-Tran, Y. Gu, Y. Ikeda, P. H. Chu, J. J. Ross, W. R. Giles and K. R. Chien, Cell, 2001, 107, 801 CrossRef CAS.
- S. P. Yu, L. M. T. Canzoniero and D. W. Choi, Curr. Opin. Cell Biol., 2001, 13, 405 CrossRef CAS.
- W. Walz, Neurochem. Int., 2000, 36, 291 CrossRef CAS.
- B. Yu, L. Nie and S. Yao, J. Chromatogr. B: Biomed. Sci. Appl., 1997, 693, 43 CrossRef CAS.
- X. F. Zhou, F. Y. Su, Y. Q. Tian, C. Youngbull, R. H. Johnson and D. R. Meldrum, J. Am. Chem. Soc., 2011, 133, 18530 CrossRef CAS PubMed.
- B. Kim, I. H. Jung, M. Kang, H. K. Shim and H. Y. Woo, J. Am. Chem. Soc., 2012, 134, 3133 CrossRef CAS PubMed.
- D. M. Kong, J. H. Guo, W. Yang, Y. E. Ma and H. X. Shen, Biosens. Bioelectron., 2009, 25, 88 CrossRef PubMed.
- J. T. Ren, J. H. Wang, J. Wang, N. W. Luedtke and E. K. Wang, Biosens. Bioelectron., 2012, 31, 316 CrossRef CAS PubMed.
- W. Yuanboonlim, W. Siripornnoppakhun, N. Niamnont, P. Rashatasakhon, T. Vilaivan and M. Sukwattanasinitt, Biosens. Bioelectron., 2012, 33, 17 CrossRef CAS PubMed.
- Z. B. Chen, Y. Q. Huang, X. X. Li, T. Zhou, H. Ma, H. Qiang and Y. F. Liu, Anal. Chim. Acta, 2013, 787, 189 CrossRef CAS PubMed.
- B. X. Ge, Y. C. Huang, D. Sen and H. Z. Yu, Angew. Chem., Int. Ed., 2010, 49, 9965 CrossRef CAS PubMed.
- A. E. Radi and C. K. O'Sullivan, Chem. Commun., 2006, 3432 RSC.
- Z. S. Wu, C. R. Chen, G. L. Shen and R. Q. Yu, Biomaterials, 2008, 29, 2689 CrossRef CAS PubMed.
- M. T. Castaneda, S. Alegret and A. Merkoci, Electroanalysis, 2007, 19, 743 CrossRef CAS.
- G. Frens, Nature (London), Phys. Sci., 1973, 241, 20 CrossRef CAS.
- A. Doron, E. Katz and I. Willner, Langmuir, 1995, 11, 1313 CrossRef CAS.
- A. W. Peterson, L. K. Wolf and R. M. Georgiadis, J. Am. Chem. Soc., 2002, 124, 14601 CrossRef CAS PubMed.
- H. Z. Yu, C. Y. Luo, C. G. Sankar and D. Sen, Anal. Chem., 2003, 75, 3902 CrossRef CAS.
- R. Szamocki, A. Velichko and C. Holzapfel, Anal. Chem., 2007, 79, 533 CrossRef CAS PubMed.
- H. Kaiser, Pure Appl. Chem., 1973, 34, 35 CrossRef CAS.
Footnote |
† Electronic supplementary information (ESI) available. See DOI: 10.1039/c4ra05058d |
|
This journal is © The Royal Society of Chemistry 2014 |