DOI:
10.1039/C4RA03504F
(Paper)
RSC Adv., 2014,
4, 34037-34044
Quantitative determination of methylene blue in environmental samples by solid-phase extraction and ultra-performance liquid chromatography-tandem mass spectrometry: a green approach†
Received
17th April 2014
, Accepted 16th July 2014
First published on 16th July 2014
Abstract
Industrial effluents with dyes may contain appreciable concentrations of materials with high chemical oxygen demand and suspended solids, posing adverse effects to both humans and aquatic life; therefore, these effluents require quantitative monitoring. In the present study, an analytical method based on solid-phase extraction (SPE) and ultra-performance liquid chromatography-tandem mass spectrometry (UPLC-MS/MS) has been optimized for the quantitative analysis of methylene blue (MB) in environmental samples. For the extraction of MB, a variety of solvents, including formic acid, were investigated to obtain optimum recovery. MB extraction using the SPE method was best achieved using methanol with 1 M formic acid. Chromatographic separation of MB and methylene violet 3RAX (MV 3RAX, internal standard) was accomplished on an Acquity® BEH C18 column using water (64.99%) with formic acid (0.01%) and acetonitrile (35%) in isocratic elution mode. Chromatographic separation for both MB and MV 3RAX was achieved in <2 min with good resolution and superior peak symmetry. MB and MV 3RAX were quantified with electrospray ionization coupled with MS/MS in the multiple reaction monitoring mode. Good quality parameters were achieved for instance linearity (r2 > 0.999), run-to-run and day-to-day precisions with relative standard deviations of <4%, sensitivity with a low limit of detection (LOD) of 0.1 ng mL−1 and limit of quantification (LOQ) of 0.4 ng mL−1 were obtained. Industrial wastewater samples (paper, textile, laundry and printing press) were analyzed, and an MB level was found in between 0.36 and 1.08 μg mL−1 with excellent recovery rates (95–99%) depending on samples.
1. Introduction
Because the use of dyes and pigments has increased as civilization has evolved, gallons of waste effluents are being generated. These waste effluents limit sunlight penetration, reduce photosynthetic activity and dissolved oxygen concentration and cause adverse effects on both flora and fauna. Methylene blue (MB; 3,7-bis(dimethylamino)-phenothiazin-5-ium chloride), a cationic dye, is widely used in textiles, printing, and research laboratories. Methylene blue is a well-known photosensitizer that has been extensively used as an optical probe in several biochemical systems.1 The U.S. Food and Drug Administration (FDA) has approved MB for various testing applications.1 However, MB poisoning can cause eye burns on direct contact in addition to nausea, vomiting, profuse sweating, and mental confusion and, if ingested, it can cause methemoglobinemia.2
To estimate the intake and threats to human health, it is highly essential to quantify the amounts of MB present in a variety of industrial wastewater effluents. However, because of a low level of MB concentration and a high number of matrix interferences found in wastewater samples, the development of a fast analytical technique that unambiguously determines MB in these complex matrices has been challenging. For MB assays, a number of analytical methods have been reported in various matrices. Typically, extraction and enrichment steps, including liquid–liquid extraction (LLE)3–5 and solid-phase extraction (SPE),6–8 are performed, followed by separation methods such as liquid chromatography (LC),9–12 capillary electrophoresis (CE)13–17 or ion chromatography (IC), coupled to either optical18 or mass spectrometric detectors.11 However, co-extracted substances from wastewater sample matrix often emerge and can impede the determination of MB. One of the most significant features of MB analysis is the authentication of chromatographic peaks using selective methods because several peaks with the same retention times as those of MB are often present in the chromatograms leading to false peak detection. Indeed, almost all these conventional extraction and detection methods are lengthy, require large amounts of organic solvents, which can be expensive (SPE employs significantly a smaller amount of organic solvent than LLE, but it can be comparatively expensive), and use large injection volumes with limited sensitivity and selectivity. To sort out this problem, the coupling of more selective methods such as mass spectrometry that permits the unequivocal detection of the compounds is required. In addition, to reduce sample extraction and enrichment cost, it is essential to develop economically and ecologically appealing SPE biomass materials. Globally, research continues for the exploration of new biosorbents and modifying them to enhance their performances for the removal and recovery of metal or metalloid species, organic compounds and particulates from synthetic and wastewater samples.19 Considering the environmental and economic factors, the expanded use of biosorbents in SPE is essential for developing an analytical method to identify and quantify the traces of MB in wastewater samples, benefiting from gains in sensitivity and selectivity, thereby reducing analysis time and solvent consumption.
To the best of our knowledge, previous studies have reported the use of commercially available SPE cartridges. These cartridges are expensive, ecologically unsafe and lengthy. None of the previous studies have reported the use of biomass for preparing SPE cartridges. In addition, the matrix used in previous studies was an aquatic product. Therefore, in the present research, efforts have been made to develop a novel, two-step analytical method based on solid-phase extraction using biosorbents and reversed-phased ultra-performance liquid chromatography-tandem mass spectrometry for extraction enrichment, and the quantification of MB in industrial wastewater samples as the matrix. A green SPE extraction method based on (pistachio shell) biomass was developed. The overall high throughput offered by the proposed method can be advantageous for this type of analysis.
2. Material and methods
2.1. Chemicals and reagents
The solvents and chemicals used in this study were of LC or analytical reagent (AR) grade. The chemical structure of the investigated compounds is shown in Fig. 1. MB (purity 82%) and formic acid (98%) were purchased from Panreac (Barcelona, Spain). Methylene violet 3RAX (MV 3RAX; purity 90%) was used as the internal standard (IS) and was obtained from Aldrich (St. Louis, Mo., USA). Methanol, acetone, dichloromethane, ethyl acetate and acetonitrile were purchased from Merck (Darmstadt, Germany). Water was purified through a Milli-Q water purification system (Millipore Corporation, Bedford, Mass., USA).
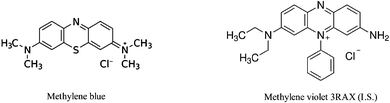 |
| Fig. 1 Chemical structures of investigated dyes. | |
Extraction columns (Extrelut-20) were provided by Merck (Darmstadt, Germany), and coupling pieces and stopcocks were from Varian (Harbor City, Calif., USA). In the SPE method and solvent evaporation, Visiprep™ and Visidry™ vacuum manifolds (Supelco, Gland, Switzerland) were used, respectively. Argon of high purity (99.99%) was supplied by Speciality Gas Centre (Jeddah, Saudi Arabia). An MB methanolic stock standard solution of 100 μg mL−1 concentration was prepared and used for further dilutions. Standard mixtures of MB with MV 3RAX (IS) at varied concentration levels (0.01–5.0 μg mL−1) were prepared to establish the range of linearity and for calibration curves in all the systems. Standards and samples were filtered through a 0.22 μm PTFE syringe filter (Chromafil® Xtra, Macherey-Nagel, Duren, Germany) before being injected into the UPLC-MS/MS system. MB quantification was achieved using the standard addition method at different concentration levels.
2.2. Instrumentation
Samples were analyzed using an Acquity® UPLC system equipped with a quaternary pump (Waters, Milford, Mass., USA). The reversed-phase analytical column used was an Acquity® BEH C18 column with a dimension of 50 mm × 2.1 mm id and a 1.7 μm particle size (Waters, Milford, Mass., USA). The best possible chromatographic separation of MB and MV 3RAX was achieved with a mobile phase consisting of 0.01% of formic acid in water (64.99%) and acetonitrile (35%) in isocratic elution mode at a flow rate of 300 μL min−1. The sample injection volume was 5 μL. The column was washed with water–methanol (50
:
50, v/v) for 5 min after every 20 injections.
The UPLC system was equipped with an electrospray ionization source (ESI, Z-spray) and Quattro Premier triple quadrupole mass analyzer (Micromass, Milford, Mass., USA). For MB and MV 3RAX, the MS system was operated in the positive ionization mode, and the data were acquired in multiple reaction monitoring (MRM). The optimized source working conditions for monitoring MB and MV 3RAX were capillary voltage, 3.5 kV; cone voltage, 68 V; source temperature, 120 °C; desolvation temperature, 350 °C; desolvation gas flow rate, 700 L h−1; and cone gas flow rate, 70 L h−1. High-purity nitrogen gas (99.99%), generated using a nitrogen generator model NM30LA (Peak Scientific, Inchinnan, United Kingdom) and argon were used as cone and collision gases, respectively. An Oerlikon rotary pump, model SOGEVACSV40 BI (Cedex, France) was used to provide the primary vacuum to the MS system. The MS/MS parameters, including collision energy voltages, dwell times and the precursors and daughter ions related to the selected transitions of MB and MV 3RAX, are illustrated in Table 1. The most abundant daughter ion was monitored to estimate MB and MV 3RAX, and the second-most-abundant daughter ion was monitored to authenticate MB and MV 3RAX identification. Data acquisition was performed by MassLynx V4.1 software (Waters, Milford, Mass., USA).
Table 1 MRM parameters used with the MS/MS instrumenta
Analyte |
Precursor ion (m/z) |
Quantification |
Confirmation |
Collision energy (V) |
Daughter ion (m/z) |
Collision energy (V) |
Daughter ion (m/z) |
Dwell time was 0.025 s in all the cases. |
MB |
284 |
60 |
269 |
60 |
240 |
MV 3RAX |
343 |
72 |
299 |
72 |
327 |
Fourier transform infra-red (FT-IR) spectra of samples in the 4000–600 cm−1 range were recorded using a Varian 3100 spectrometer, USA. The wide-angle X-ray diffraction (WXRD) pattern of biosorbent was recorded using a Philips Xpert wide-angle X-ray diffractometer with nickel-filtered CuKα radiation (1.54056).
2.3. Sample analysis
2.3.1. Preparation of biosorbent for SPE procedure. Solid-phase extraction is a well-known practical extraction technique. It can be used to isolate the compounds of interest from a variety of complex matrices, including soil, water, urine, blood, animal tissue, and beverages.20–22 The solid-phase adsorbent is the most important part of the extraction system, and extraction recovery typically depends on adsorbent properties.20–22 The modification of adsorbent with different functional groups could change the extraction properties of the final adsorbent. Thus, it is essential to select the most suitable SPE adsorbent for specific application.In this study, pistachio shells (PS), a lignocellulose biomass was used as the SPE biosorbent. PS were collected from local dry fruit suppliers (Riyadh, Saudi Arabia), washed with Milli-Q water to remove dust and dirt, dried in an oven at 60 °C for 24 h. The dried PS were ground and sieved to 0.2–0.4 mm particle size. Biomass (PS, 1.0 g) was treated with 100 mL hydrogen peroxide (30% w/w) and the resulting mixture was kept in a water bath shaker at 50 °C for 60 min with constant stirring at 100 rpm to oxidize organic content. Afterward, the biomass was washed several times with Milli-Q water to remove the traces of hydrogen peroxide. The oxidized biomass was again treated with alkaline (0.1 M NaOH) solution in a water bath shaker at 25 °C for 24 h with constant stirring at 100 rpm. The alkaline treatment of lignocellulose containing biomass results in swelling, increasing its internal surface area by disrupting the lignin structure, making carbohydrates in the heteromatix more accessible to binding adsorbate, and decreasing both the degree of polymerization and cellulose crystallinity.23 Considering environmental and economic aspects, a lower environmental load in its life cycle, less corrosive ability, and economic feasibility are the major merits of using NaOH as a modifying agent.24 The NaOH-treated biomass was washed several times with Milli-Q water to achieve neutral pH. The resultant biomass was dried in an oven at 65 °C for 24 h to obtain constant weight, and the PS biomass was kept in sealed plastic bags and stored in a desiccator to avoid moisture contamination.
2.3.2. Sample extraction procedure. Wastewater samples were collected using glass bottles (250 mL) from different industries based in Saudi Arabia. The samples were filtered through Whatman filter paper (no. 42), (Maidstone, United Kingdom) and stored at 4 °C to avoid any microbial contamination. 1.0 g of biosorbent was used to fill an empty Extrelut column (6 mL) followed by washing with Milli-Q water (50 mL) and drying under vacuum for 10 min. The filtered wastewater samples (20 mL) were passed through the biosorbent at a controlled flow rate of 1 mL min−1. The biosorbent was then rinsed with Milli-Q water (50 mL) and dried under vacuum for 10 min. MB adsorbed on the Extrelut packing was eluted using methanol (50 mL) with 1 M formic acid at a controlled flow rate of 1 mL min−1. The sample extract was evaporated to dryness under a stream of nitrogen, and the analyte was reconstituted with 1 mL of MV 3RAX (I.S.) in methanol–water (50/50, v/v). The final extract was filtered through a 0.22 μm PTFE syringe filter prior to UPLC-MS/MS analysis.To assess the recovery rates of MB separated and analyzed using this SPE procedure, in addition to preventing the matrix effect influence on peak retention time in the UPLC-MS/MS chromatograms, two non-spiked and three spiked samples (50%, 200%, and 500%) were analyzed by the standard addition quantification method. The samples were spiked at the beginning of the extraction process.
3. Results and discussion
3.1. Characterization of SPE biosorbent
3.1.1. FT-IR spectroscopic analysis. The major constituents of the PS biomass are hemicellulose, cellulose, and lignin.25 These constituents were confirmed from the obtained peaks in 1750–1500 cm−1 (Fig. 2a), which were attributed to the stretching vibrations of keto –C
O groups (1732 cm−1) and the C
C stretching vibrations of aromatic rings (1597 and 1494 cm−1) in lignin. The peaks at 1500–1314 cm−1 were observed because of the bending vibrations of O–H and aliphatic deformation vibration of the –CH3 and –CH2 groups present in cellulose and lignin. The absorption bands in 1300–1000 cm−1 were assigned to the stretching vibration of C–O in carboxyl groups, alcohol and phenol constituents.26 The presence of cellulose in the PS biosorbent was confirmed from the relatively high intensity peak at 1026 cm−1 because of the stretching vibration of C–O in alcohols and phenol.25 The obtained results proved that the cellulose constituent was left in PS biosorbent, and other constituents, such as lignin and hemicellulose, were dissolved after treatment with NaOH. The FT-IR spectra of PS biosorbent after MB biosorption was also recorded (Fig. 2a). The reduction in the intensity of absorption bands at 3311 and 1026 cm−1 was observed after biosorption. This confirmed that MB biosorption occurred through hydrogen bonding between the acidic oxygen groups (O–H and phenolic) on the PS and nitrogen atoms present in MB.
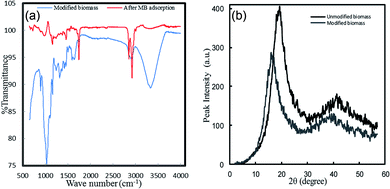 |
| Fig. 2 Fourier transform infra-red (FT-IR) spectra (a), and wide angle X-ray diffraction (WXRD) pattern (b) of PS biomass. | |
3.1.2. WXRD analysis. The WXRD patterns of the PS before and after NaOH treatment are shown in Fig. 2b. The intensity of the peak for the PS biosorbent after NaOH treatment was reduced, which confirmed that its amorphous nature was improved because lignin and hemicellulose constituents were leached out.
3.2. Optimization of SPE method
Because there are no earlier studies on the extraction of MB from wastewater samples using SPE and UPLC-MS/MS method, preliminary studies were performed on standard solutions of MB. To find the most favorable solvent for MB extraction using the SPE technique, various solvents, such as dichloromethane, ethyl acetate, acetone and methanol, were tested. The obtained results demonstrated that none of the aforementioned solvents extracts MB efficiently, and the obtained recovery rates were almost negligible. Thus, several experiments were carried out using a sample modifier, such as a formic acid proportion, in each extraction solvent to enhance the recovery rate of the target analyte. Among the tested solvents, methanol at varied formic acid concentrations (0.1–1 M) showed comparatively higher recovery values ranging from 75 to 104% (Fig. 3). From all the solvents tested, a methanolic solution of formic acid (1 M) produced the maximum MB recovery of 104%. As a result, a methanolic solution containing formic acid (1 M) was chosen as the most favorable extraction solvent for MB in the wastewater sample.
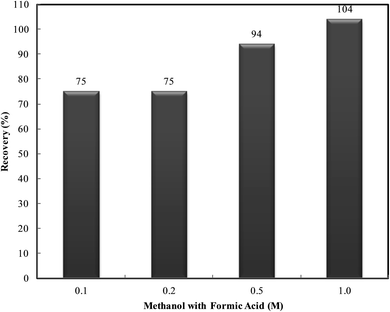 |
| Fig. 3 Effect of sample modifier (formic acid) on MB extraction using the SPE method. | |
3.3. Optimization of chromatographic separation
The development of a method for the high-throughput quantification of MB using UPLC is significant because this is the first method for the analysis of MB with this advanced technique. The UPLC method has been used to improve the chromatographic performance of established liquid chromatography in terms of speed, resolution and sensitivity.27,28 Various of analytical columns and conditions were investigated to optimize chromatographic separation in terms of analysis time, peak symmetry and resolution. The Acquity® UPLC BEH C8, C18 columns were tested. These columns incorporate trifunctional ligand bonding chemistries, which produce superior low-pH stability and ultra-low column bleed. This low-pH stability is combined with the high-pH stability of the 1.7 μm BEH particle to deliver the widest usable pH operating range. In addition, these new chemistries employ new, proprietary end-capping processes, which produce the highest efficiencies, sharpest peaks and maximum MS sensitivities. An Acquity® UPLC hydrophilic interaction chromatography (HILIC) column with a stationary phase having amide groups was also investigated, which are designed to retain and separate highly polar compounds. HILIC is an alternative of normal phase liquid chromatography (NP-LC) but the separation mechanism used in HILIC is more complicated than that used in NP-LC. Similar to NP-LC, HILIC uses conventional polar stationary phases, such as silica, amino or cyano,29 but the mobile phase used is similar to those employed in the reversed-phase liquid chromatography mode;29 it can be conveniently coupled to mass spectrometry (MS), particularly in the electrospray ionization (ESI) mode. A standard mixture solution of MB and MV 3RAX at 1.0 μg mL−1 was injected into the column of UPLC system, and the mobile phase was optimized with methanol, water and acetonitrile alone and at different proportions. The effect of the addition of formic acid (1–0.01%) in mobile phase was also studied. The most reliable results (resolution, high peak symmetry, reproducible and signal-to-noise ratio values) acquired for target analyte peaks were favorable when using Acquity® UPLC BEH C18 column and mobile phase, 65% of Milli-Q water with formic acid (0.1%, v/v) and 35% of acetonitrile in isocratic elution mode. The amount of formic acid in the mobile phase leads to Gaussian peaks and improves the peak symmetry of the investigated compounds. A fairly low mobile phase flow rate of 300 μL min−1 was established to be the most favorable for the analysis of MB and MV 3RAX, which favored efficient ionic evaporation and desolvation in the ion source (ESI) and a chromatographic peak wide enough to be defined by at least 15 scan points in their detection. With these optimum conditions, the retention time for MB and MV 3RAX was <2 min. Among the Acquity® UPLC BEH C8, C18 and HILIC columns tested for MB and MV 3RAX separation, the Acquity® UPLC BEH C18 column illustrated the highest capacity factor and was chosen for further analysis. As can be seen from Fig. S1 (ESI†), the obtained chromatograms of MB and MV 3RAX (standard mixture, 1.0 μg mL−1) shows good resolution and high-peak symmetry. An additional benefit of an UPLC method using a reversed-phase column is that this type of column is usually obtainable in laboratories with UPLC systems.
3.4. Optimization of MS detection
Mass spectrometric conditions were optimized by infusing a methanolic mixture solutions of MB and MV 3RAX at concentration levels of 5 μg mL−1, depending on the sensitivity of the compound. The experiments were performed in both positive and negative ionization modes. Initially, full-scan acquisitions were performed to select the precursor ion and optimum cone voltage. The investigated compounds offered higher sensitivity in the positive ionization mode and in concurrence with the previously developed LC-MS method.30 Ionization by ESI by adding H+ to the target compounds could not occur because MB and MV 3RAX are already positively charged; nevertheless, both the compounds could be analyzed, particularly because they build up intensive [M]+ ions. As a result, the positive ionization mode led to enhanced sensitivity for the studied compounds; thus, it was chosen for further experiments.
Subsequent to the affirmation of precursor ions, more than two daughter ions should be chosen when using LC-MS analysis according to European Union legislation.31 As stated by these guidelines, the first transition should be used for quantification, whereas the second transition should be used to authenticate the presence of target analytes during the analysis of real samples. In this study, once the precursor ions were chosen, daughter-scan acquisitions were carried out at various collision energies to select daughter ions and the most favorable collision energies. Two MRM transitions were acquired for both MB and MV 3RAX; the most abundant transition was generally chosen for quantification, and the other transition was selected for confirmation. The MS/MS transitions monitored in the positive ionization mode were 284 > 269, 284 > 240 at 60 V collision energy and 343 > 299, 343 > 327 at 72 V collision energy for MB and MV 3RAX, respectively. The dwell time was 0.025 s in both the cases. The individual mass spectra and fragmentation pattern for the investigated dyes have been illustrated in Fig. 4. The obtained mass transitions in the MRM mode were used for unambiguous and sensitive quantification.
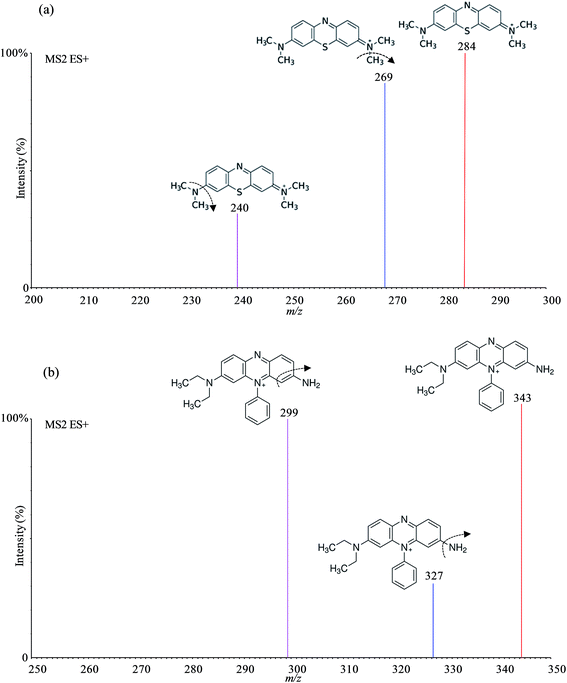 |
| Fig. 4 MS/MS spectra of MB precursor ions, m/z 284 (a), and MV 3RAX, m/z 343 (b) with the proposed fragmentation. | |
3.5. Method validation
Under the optimum chromatographic and MS conditions, the proposed UPLC-MS/MS method was validated by linearity, limit of detection, limit of quantification, repeatability (run-to-run precision), reproducibility (day-to-day precision) and recovery. The linearity of the method was investigated over a wide range of MB concentration (0.01–5 μg mL−1). The obtained peak area was plotted against the concentration, and the correlation coefficient (R2) was found to be higher than 0.999, which showed that the response was linear.
LOD and LOQ are terms used to illustrate the minimum concentration of a compound that can be consistently calculated by an analytical procedure. The LOD as well as LOQ were estimated for a signal-to-noise ratio (S/N) of 3 and 10, respectively; the obtained LOD and LOQ values were 0.1 ng mL−1 and 0.4 ng mL−1 in the standard, and 0.3 ng mL−1 and 0.9 ng mL−1 in the sample spiked at the lowest concentration level. The LOD and LOQ values were calculated using statistical LINEST function.
The run-to-run precision was estimated from five replicate injections of MB standard (0.5 μg mL−1) and a laundry sample with a known calculated concentration (0.36 μg mL−1) on the same day into the UPLC-MS/MS system. These determinations were evaluated on the basis of the calculation of relative standard deviation (%RSD) of the peak area values. The RSD values obtained for repeatability were 1.86% in the analysis of the standard and 2.52% in the analysis of laundry sample. The day-to-day precision was calculated by five replicate injections of the MB standard (0.5 μg mL−1) and a laundry sample with a known calculated concentration (0.36 μg mL−1) along three consecutive days into the UPLC-MS/MS system. These findings were estimated on the basis of the calculation of the RSD of the peak area values. The RSD values obtained for reproducibility were 2.35% in the analysis of the standard and 3.98% in the analysis of the laundry sample. The precisions of the proposed UPLC-MS/MS method for the analysis of low levels of MB either in standard or in real samples were found to be adequate for quantification.
The effect of sample matrix on the signal-to-noise ratio of target compound has been assessed using the standard addition quantification method. A total of four industrial wastewater samples were analyzed, and the recovery rates for MB were found to be between 95 and 99%, as detailed in Table 2. The low matrix effect can be due in part to the chromatographic conditions; the Z-configuration of the ionization source of the MS system, which prevented the entrance of neutral compounds in the MS. These results indicate that the matrix does not alter the signal of target compounds in this type of sample.
Table 2 MB concentration levels and recovery rates in wastewater samples
Sample |
MBa (μg mL−1 ± SD) |
Recovery (%) |
Standard deviation (SD) was obtained from the addition standard calibration curve. |
Paper |
0.54 ± 0.02 |
96 |
Textile |
1.08 ± 0.04 |
99 |
Laundry |
0.36 ± 0.01 |
95 |
Printing press |
0.83 ± 0.03 |
98 |
3.6. Application of UPLC-MS/MS method
The practical applicability of SPE and UPLC-MS/MS method was established for the analysis of MB in industrial wastewater samples. The detection of MB was not affected by the investigated sample matrices, as determined experimentally. The results of MB concentration are presented in Table 2, the lowest concentration of MB (0.36 μg mL−1) was found in the laundry sample; however, the highest MB concentration (1.08 μg mL−1) was found in the textile samples. As an example, Fig. 5 demonstrates the chromatograms of MB and MV 3RAX (IS) analyzed with the proposed UPLC-MS/MS method. The low matrix effect can be due in part to the optimized green SPE procedure, chromatographic conditions and the Z-configuration of ESI source of the MS system, which prevented the entrance of neutral compounds in the MS system. These results signify that the matrix does not alter the target analyte signal in such sample types. The analysis of blanks carried out routinely indicated that cross contamination during the analytical process did not occur. These results are a source of data on the presence of MB in industrial wastewater samples.
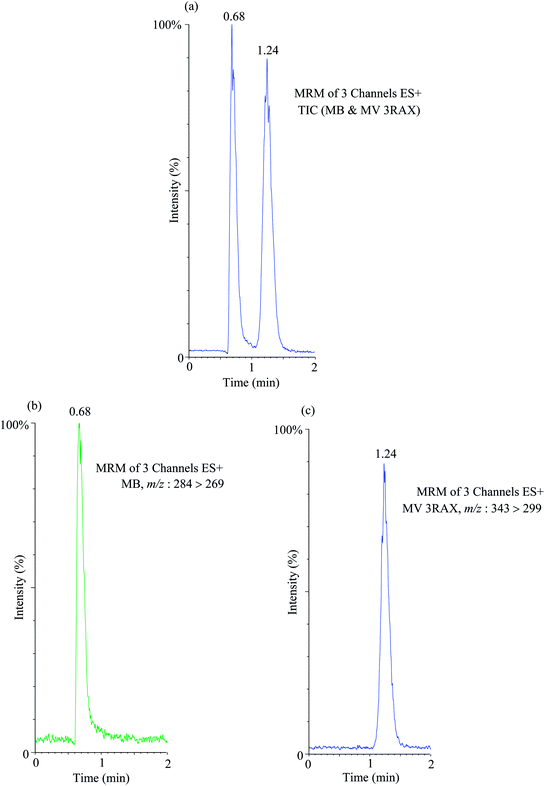 |
| Fig. 5 UPLC-MS/MS chromatograms of textile samples (a) MB & MV 3RAX, TIC (b) MB, m/z 284 → 269 (c) MV 3RAX, m/z 343 → 299. | |
4. Conclusions
A highly sensitive, speedy, cost-effective, eco-friendly and reliable method based on SPE and UPLC-MS/MS has been developed to quantify MB in industrial wastewater samples. The absence of matrix effects observed in the analysis and minimal sample pre-treatment without the loss of any target analyte offered by a low-cost SPE technique is a significant advantage because added steps during the sample preparation would increase analysis time, solvent consumption, variability and significant losses of the target analyte and sensitivity. The performance of the method in terms of good sensitivity, linearity and precision, and very short analysis time achieved with this method, as well as the results obtained in the analysis, enable a new analytical methodology for the routine analysis of MB in industrial wastewater samples. In the future, this proposed method could be extensively applied in the estimation of MB exposure of humans and aquatic animals.
Acknowledgements
This research was supported by the King Saud University, Deanship of Scientific Research, College of Science Research Center.
References
- M. Hossain, A. Kabir and S. Kumar, Dyes Pigm., 2012, 92, 1376–1383 CrossRef CAS PubMed
. - K. A. G. Gusmão, L. V. A. Gurgel, T. M. S. Melo and L. F. Gil, Dyes Pigm., 2012, 92, 967–974 CrossRef PubMed
. - B. Haddou, Z. Bouberka, Z. Derriche, A. Taibi and H. Bouabdesselam, Sep. Sci. Technol., 2007, 42, 2677–2691 CrossRef CAS
. - C. Das, M. Rungta, G. Arya, S. DasGupta and S. De, J. Hazard. Mater., 2008, 159, 365–371 CrossRef CAS PubMed
. - J. Z. Xu, L. Dai, B. Wu, T. Ding, J. J. Zhu, H. Lin, H. L. Chen, C. Y. Shen and Y. Jiang, J. Sep. Sci., 2009, 32, 4193–4199 CrossRef CAS PubMed
. - V. J. P. Vilar, C. M. S. Botelho and R. A. R. Boaventura, J. Hazard. Mater., 2007, 147, 120–132 CrossRef CAS PubMed
. - Z. Zhang, Z. Zhang, Y. Fernandez, J. A. Menendez, H. Niu, J. Peng, L. Zhang and S. Guo, Appl. Surf. Sci., 2010, 256, 2569–2576 CrossRef CAS PubMed
. - F. A. Ozdemir, B. Demirata and R. Apak, J. Appl. Polym. Sci., 2009, 11, 3442–3448 CrossRef PubMed
. - S. B. Turnispeed, J. E. Roybal, S. M. Plakas, A. P. Pfenning, J. A. Hurlbut and A. R. Long, J. AOAC Int., 1997, 80, 31–35 Search PubMed
. - R. D. Voyksner, C. S. Smith and P. C. Knox, Biomed. Environ. Mass Spectrom., 1990, 19, 523–534 CrossRef CAS PubMed
. - A. Raffaelli, S. Pucci, I. Desideri, C. R. Bellina, R. Bianchi and P. Salvadori, J. Chromatogr. A, 1999, 854, 57–67 CrossRef CAS
. - D. Tang and P. H. Santschi, J. Chromatogr. A, 2000, 883, 305–309 CrossRef CAS
. - J. Ren, B. Li, Y. Deng and J. Cheng, Talanta, 1995, 42, 1891–1895 CrossRef CAS
. - P. Jing, T. Kaneta and T. Imasaka, J. Chromatogr. A, 2002, 959, 281–287 CrossRef CAS
. - Y. Hu, J. Cheng and Y. Deng, Analyst, 1997, 122, 1089–1093 Search PubMed
. - T. Higashijima, T. Fuchigami, T. Imasaka and N. Ishibashi, Anal. Chem., 1992, 64, 711–714 CrossRef CAS
. - S. E. Mylon and G. Benoit, Environ. Sci. Technol., 2001, 35, 4544–4548 CrossRef CAS
. - N. F. Gaudette and J. W. Lodge, J. Anal. Toxicol., 2005, 29, 28–33 Search PubMed
. - J. Wang and C. Chen, Biotechnol. Adv., 2009, 27, 195–226 CrossRef CAS PubMed
. - N. J. K. Simpson, Solid-Phase Extraction: Principles, Techniques, and Applications, CRC, 2000, ISBN 978-0-8247-0021-8 Search PubMed
. - E. M. Thurman and M. S. Mills, Solid-Phase Extraction: Principles and Practice, Wiley-Interscience, 1998, ISBN 978-0-471-61422-7 Search PubMed
. - J. S. Fritz, Analytical Solid-Phase Extraction, Wiley-VCH, 1999, ISBN 978-0-471-24667-1 Search PubMed
. - V. B. Agbor, N. Cicek, R. Sparling, A. Berlin and D. B. Levin, Biotechnol. Adv., 2011, 29, 675–685 CrossRef CAS PubMed
. - K. Y. Foo and B. H. Hameed, Bioresour. Technol., 2012, 116, 522–525 Search PubMed
. - B. Peters, Fuel Process. Technol., 2011, 92, 1993–1998 CrossRef CAS PubMed
. - G. Socrates, Infrared Characteristic Group Frequencies, Wiley, New York, 1980 Search PubMed
. - M. E. Swartz, J. Liq. Chromatogr. Relat. Technol., 2005, 28, 1253–1263 Search PubMed
. - J. E. Mac Nair, K. C. Lewis and J. W. Jorgenson, Anal. Chem., 1997, 69, 983–989 CrossRef CAS
. - Y. Guo and S. Gaiki, J. Chromatogr. A, 2005, 1074, 71–80 Search PubMed
. - J. Burhenne, K. D. Riedel, J. Rengelshausen, P. Meissner, O. Muller, M. Gerd, W. E. Haefeli and W. S. Ingeborg, J. Chromatogr. B: Anal. Technol. Biomed. Life Sci., 2008, 863, 273–282 CrossRef CAS PubMed
. - EU Commission Decision 2002/657/EC of 12 August 2002, implementing Council Directive (EC) 96/23 concerning the performance of analytical methods and the interpretation of the results (text with EEA relevance), Off. J. Eur. Comm.L221 (2002) 8.
Footnote |
† Electronic supplementary information (ESI) available. See DOI: 10.1039/c4ra03504f |
|
This journal is © The Royal Society of Chemistry 2014 |